DOI:
10.1039/D1MA01050F
(Paper)
Mater. Adv., 2022,
3, 2185-2190
Preparation of heterostructured TiO2/MoS2 for efficient photocatalytic rhodamine B degradation†
Received
10th November 2021
, Accepted 9th January 2022
First published on 12th January 2022
Abstract
Coating a few layers of MoS2 nanosheets on a substrate is an effective approach to enhance catalytic activity for photocatalytic degradation. Herein, we fabricated heterostructured TiO2/MoS2 (H-TiO2/MoS2) with high structural stability via a simple two-step solvothermal approach. H-TiO2/MoS2 was composed of TiO2 as a hard core and MoS2 nanosheets as a shell, which could increase the electron transfer rate between TiO2 and MoS2 and enable active edge sites of MoS2 to be maximally exposed. Besides, H-TiO2/MoS2 indicated enhanced light absorption in the UV to Vis range when compared to TiO2 nanoparticles, and slightly lower than that of MoS2. This is beneficial for the enhancement of the photocatalytic degradation performance. Therefore, H-TiO2/MoS2 displayed a strong adsorption ability toward organic dyes and showed excellent performance in the photocatalytic degradation of rhodamine B with the concentration decreased by 99.4% due to the synergistically stimulative effect. The work will enlighten the development of highly efficient molybdenum sulfide-based heterostructured photocatalysts.
Introduction
Nowadays, semiconductor photocatalysts are attracting increasing attention owing to their high efficiency in easing the energy crisis and reducing environmental pollution. Recently, a large variety of semiconductor photocatalysts have been explored including TiO2,1,2 SrTiO3,3etc., which were mainly active in the ultraviolet range, and C3N4,4 Cu2O, Co3O4,5 CdS,6etc. that have high visible light activity. TiO2, as an n-type photocatalytic semiconductor, is efficient for the separation of electrons and holes. Furthermore, it has both good chemical and physical stability, relatively low cost and nontoxicity, and thus has a wide range of applications in various fields, such as self-cleaning and removal of hazardous compounds. However, the large band gap of TiO2 (3.2 eV) has become the main drawback because limited UV light could be used, which significantly decreased the photocatalytic performance. Additionally, pure TiO2 usually did not have a high charge separation rate, and therefore illustrated relatively low photocatalytic activity. Thus, many research studies have been conducted to settle these inadequacies including tuning the particle size,7 crystallinity and morphologies (nanotubes8 in 1D, nanosheets9 in 2D and microspheres10 or nanoflowers11 in 3D), and constructing heterostructured materials,12–14 which have resulted in the promotion of the photocatalytic performance. Among these nanostructures, heterostructures like core–shell structures15 with a large specific surface area and matched energy levels have attracted great attention.
Moreover, it has been reported that TiO2-based photocatalysts doped with cocatalysts like noble metals,10 MoS2,16–18etc., could broaden the range of light harvesting from the UV to UV-vis, and improve the charge separation efficiency. Two-dimensional transition metal sulfides (2D MSx), such as the typical MoS2, were reported as platinum-like materials, which were beneficial for improving the photocatalytic performance as a cocatalyst because of great (photo) electronic and catalytic traits.19 In addition, a few-layered MoS2 with increased edges was beneficial for electron acceptance, as well as increased active sites, illustrating improved photocatalytic performance. Recently, MoS2 catalysts with a variety of nanostructures have been prepared using various approaches including chemical vapour deposition,20 thermolysis21 or hydrothermal and solvothermal methods.22 Irregular aggregates of nanoparticles or stacked multilayers of the as-fabricated MoS2, however, largely limited the photocatalytic performance. Therefore, preparing heterostructured MoS2-based photocatalysts with enhanced photocatalytic activity remains challenging. Recently, MoS2-based photocatalysts such as CdS/MoS2,23,24 graphene/MoS225,26 and TiO2/MoS2 heterostructures with various structures including particles27 (0D), belts28 and wires12 (1D), sheets29 (2D) and flowers30 (3D) have demonstrated enhanced photocatalytic activities. Meanwhile, constructing novel nanostructures of core-shelled TiO2/MoS2 is also an effective approach to develop photocatalysts with large specific surface areas and increased active sites.
In this work, we prepared H-TiO2/MoS2 through a two-step solvothermal approach. In the first step, TiO2 nanoparticles were prepared as an ellipsoidal core through a solvothermal approach; then MoS2 nanosheets were coated on the surface of the TiO2 precursor again using a solvothermal method. The MoS2 nanosheets which were coated on the surface of TiO2 nanoparticles could allow fast electron transfer between TiO2 and MoS2. Furthermore, H-TiO2/MoS2 illustrated good structural stability. MoS2 nanosheets could expose active edge sites maximally, allowing an enhanced adsorption ability and improved photocatalytic degradation performance of rhodamine B (RhB). The synergistic effect of the novel heterostructure between MoS2 nanosheets and TiO2 nanoparticles accounted for the outstanding photocatalytic degradation performance.
Experimental
Chemicals
Tetrabutyl titanate (TBT, Aladdin Biochemical Technology Co., Ltd) and glacial acetic acid (Macklin Co., Ltd) were used without further purification. Absolute ethanol and Na2MoO4·2H2O were purchased from Sinopharm Chemical Regent Co., Ltd. Cysteine was purchased from Beijing Xinjingke Biotechnology Co., Ltd. Ultrapure H2O was employed in all experiments.
Preparation of TiO2
In a typical synthesis process, the TiO2 precursor was synthesized by a hydrothermal strategy.7,31 In detail, TBT (1 mL) was dropped into glacial acetic acid (15 mL) at room temperature, followed by addition of ultrapure water (0.3 mL) to initiate the hydrolysis of TBT. The mixture was stirred for ∼10 minutes at room temperature, and then transferred into a 50 mL autoclave, which was heated at 150 °C for 12 h. The as-prepared precursor was obtained by centrifugation after cooling the autoclave to room temperature and washed thoroughly with absolute ethanol and water several times with the assistance of ultrasound treatment.
Synthesis of H-TiO2/MoS2
H-TiO2/MoS2 was prepared via a solvothermal method.22 In detail, 100 mg TiO2 was dissolved into C2H5OH (10 mL) and H2O (20 mL), then Na2MoO4·2H2O (0.3 g) and cysteine (1.25 g) were added under vigorous stirring in sequence. Then the mixed solution was transferred into an autoclave (50 mL) and heated at 200 °C for 24 h. The resultant sample was obtained by centrifugation after cooling the autoclave to room temperature. Finally, the obtained sample was washed thoroughly with absolute ethanol and water several times with the assistance of ultrasound treatment. The as-fabricated H-TiO2/MoS2 was heated at 800 °C (5 °C min−1) under an Ar (5% H2) atmosphere for 2 h before collection.
Characterization
X-Ray diffraction (Bruker D8 Advance) with Ni-filtered Cu Kα radiation was used to reveal the crystal structure of the as-prepared samples at 40 kV and 40 mA with a step size of 0.02° and scan speed of 0.1 s. Transmission electron microscopy (TEM, FEI Tecnai G2 F20) and scanning electron microscopy (SEM, Zeiss Merlin compact LE0 1530 VP) were used to explore the morphologies and elemental compositions of the as-prepared samples. The Raman spectrum of TiO2/MoS2 was recorded on an Invia Qontor. Fourier transform-infrared (FT-IR) spectroscopy was performed to confirm the spectrum of TiO2/MoS2 in the range of 400–4000 cm−1 using a PerkinElmer Spectrum One spectrometer. N2 adsorption and desorption curves were obtained for the analysis of pore structure by ASAP 2460. UV-Vis absorption spectra were recorded on a PerkinElmer (Lambda 1050 +) for revealing the light absorption of the as-prepared samples.
Photocatalytic degradation measurement
Photocatalytic activity was studied at room temperature by the degradation of RhB using a 300 W Xe lamp. For the photocatalytic test, 50 mL of an aqueous suspension of RhB (15 mg L−1) and 5 mg of the samples were placed in a Pyrex glass tube. Before irradiation, the suspension was sonicated and stirred for 30 min in the dark. The mixed solution was continuously stirred during the photocatalytic reaction. During the photocatalytic reaction process, 3 mL of the suspension were collected after irradiation for the following analysis after the filtration. The concentration of RhB was monitored by measuring the absorbance at 554 nm using a UV-vis spectrometer (Lambda 1050 +). Isopropanol, DMSO and t-BuOH were used as free radical scavengers for the scavenging experiments. The pH values of RhB solution were controlled by 0.1 M HCl and 0.1 M NaOH.
Results and discussion
Morphology and composition
As illustrated in Scheme 1, H-TiO2/MoS2 was fabricated via a simple two-step method. Initially, the porous TiO2 (rice-like) precursor was prepared via a facile solvothermal method;7,31 then the TiO2 precursor was seen as a hard core, which loaded MoS2 as a shell outside, resulting in the successful preparation of H-TiO2/MoS2. Firstly, the tetrabutyl titanate precursor was hydrolyzed and nucleated into TiO2 microcrystals, and then the microcrystals gradually grew as porous TiO2. In detail, the tetrabutyl titanate precursor was hydrolyzed into numerous winding chain bundles after 2 h of reaction (Fig. S1a, ESI†). As the reaction proceeds, some chain bundles are gradually rotated and twisted into ellipsoidal aggregates which can serve as crystal nuclei.31 Finally, after 12 h of reaction, all the ellipsoidal aggregates grew into ellipsoidal particles (Fig. S1b, ESI†). The H-TiO2/MoS2 heterostructure was formed by an L-cysteine-assisted method.22,32 For the self-assembly process of the H-TiO2/MoS2 heterostructure, the porous TiO2 served as a precursor core for the adsorption of MoO42− anions. When heated in the solution-phase reaction, L-cysteine can release H2S, meanwhile acting as a sulfide source and a reducing agent.32 As the reaction time increased, H2S in situ reacted with MoO42− anions to form a two-dimensional nano-plate-like structure, which is common in other MoS2-based composites.33–35 As illustrated in Fig. S2 (ESI†), MoS2 can be loaded onto the surface of TiO2 in a short time and gradually crystallize with time.
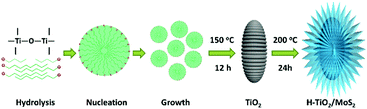 |
| Scheme 1 Scheme of the preparation process for as-prepared H-TiO2/MoS2. | |
As shown in X-ray diffraction patterns of Fig. 1, the crystal structure of the as-prepared precursor can be confirmed as TiO2 (anatase, Fig. S3a, ESI,† JCPDS No. 21-1272).7,31 Besides, the crystal structure of the resultant sample was confirmed to be H-TiO2/MoS2, which corresponds to TiO2 and MoS2 (2H, Fig. S3b, ESI,† JCPDS No. 37-1492)22 phases, indicating that H-TiO2/MoS2 was fabricated successfully.
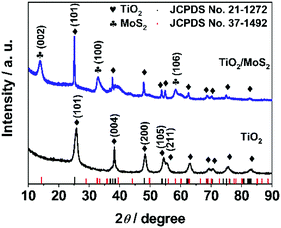 |
| Fig. 1 XRD patterns of as-prepared TiO2 and H-TiO2/MoS2. | |
To indicate the porous structure of TiO2, the characterization studies including magnified SEM and TEM images and N2 adsorption and desorption curves of a single TiO2 particle were performed. As illustrated in Fig. S4a and b (ESI†), the SEM and TEM images showed that the TiO2 particle had plenty of pores, and the N2 adsorption and desorption curves demonstrated a specific surface area of 103.07 m2 g−1 for TiO2 particles (Fig. S4c, ESI†).
The morphology of H-TiO2/MoS2 (∼240 nm) was confirmed by SEM and TEM. The SEM image in Fig. 2a illustrated that MoS2 nanosheets (∼20 nm thickness) were coated on the surface of TiO2 nanoparticles successfully, which matched well with the TEM image in Fig. 2b. Additionally, the single magnified particle in Fig. 2c clearly showed that a few layer MoS2 (∼10–30 layers) was loaded onto the surface of TiO2. What is more, as illustrated in the high-resolution TEM (HRTEM) image of Fig. 2d, the lattice fringe of 0.35 nm corresponded to the (101) plane of TiO2, and a lattice fringe of 0.6 nm corresponded to the (002) facet of MoS2.36 In order to explore the element dispersion of H-TiO2/MoS2, scanning transmission electron microscopy energy dispersive spectroscopy (STEM EDS) was employed. As shown in Fig. 2e–i, Ti and O were distributed evenly inside as a core, while Mo and S were located outside as a shell, demonstrating that MoS2 encapsulated on the surface of TiO2 successfully, which was consistent with SEM and TEM results.
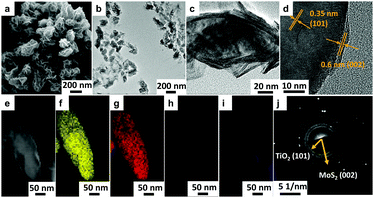 |
| Fig. 2 (a) SEM, (b) low- and (c) high-magnification TEM image, (d) HRTEM image, (e–i) STEM EDS mapping images and (j) SAED image of H-TiO2/MoS2. | |
Furthermore, the selected area electron diffraction (SAED) pattern in Fig. 2j pointed to the TiO2 (101) facet and MoS2 (002) facet, and corresponds well with the HRTEM image in Fig. 2d.
The rice-like morphology of the as-fabricated TiO2 is clearly shown in Fig. 3a with ∼200 nm in length and ∼70 nm in width. Meanwhile, MoS2 flowers were prepared and are shown in the SEM image in Fig. 3b.
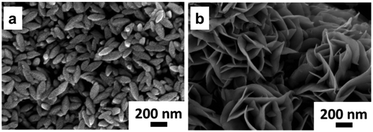 |
| Fig. 3 SEM images of (a) TiO2 nanoparticles and (b) MoS2 flowers. | |
The Raman scattering spectrum in Fig. 4a illustrated a series of Raman peaks of the as-prepared H-TiO2/MoS2, which corresponded to the typical peaks of MoS2 and TiO2. The peak located at 379 cm−1 was attributed to the in-plane E12g mode, while the peak located at 404 cm−1 was ascribed to the out-of-plane A1g mode of MoS2.37 Meanwhile, the Raman peak located at 144 cm−1 corresponded to the E1g mode of TiO2. The FT-IR spectrum of the as-prepared H-TiO2/MoS2 is shown in Fig. 4b. There are broad bands of H-TiO2/MoS2 at 486 cm−1, 903 cm−1, 1122 cm−1, and 1640 cm−1.38 The band which was located at 486 cm−1 corresponded to the Mo–S bond, while the band situated at 903 cm−1 was assigned to the S–S bond. The bands between 1122 cm−1 and 1640 cm−1 were ascribed to the stretching vibrations of –OH and Mo–O.
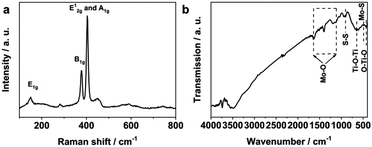 |
| Fig. 4 Raman and FTIR spectra of as-prepared H-TiO2/MoS2. | |
Photo absorption and photocatalytic degradation of RhB
UV-Vis absorption spectra were obtained to understand the optical properties. As illustrated in Fig. S5 (ESI†), H-TiO2/MoS2 showed enhanced light absorption in the UV to Vis range when compared to TiO2 nanoparticles, which is slightly lower than that of MoS2. The photocatalytic degradation of RhB for H-TiO2/MoS2 was evaluated under a 300 W Xe lamp (Fig. 5a). Before light irradiation, the photocatalyst went through an adsorption process in RhB solution in the dark for 30 min. Interestingly, it is found that H-TiO2/MoS2 illustrated a stronger adsorption ability towards RhB than TiO2 nanoparticles and MoS2 flowers, which was reported have an efficient photocatalytic performance. In addition, the RhB photodegradation efficiency of H-TiO2/MoS2 was greater than that of TiO2 nanoparticles and slightly higher than that of MoS2 flowers, suggesting the advantages of H-TiO2/MoS2 nanostructures. Specifically, the concentrations of RhB were decreased by 32.6%, 36.2%, and 29.6% after irradiation with catalyst of H-TiO2/MoS2, TiO2 nanoparticles, and MoS2 flowers, respectively. It is remarkable that the concentration of RhB decreased by 99.4% using H-TiO2/MoS2, which was beneficial for RhB adsorption and degradation. As shown in Fig. 5b, the recycling stability of H-TiO2/MoS2 was tested for 5 cycles, and illustrated no evident decay, which demonstrated a good stability.
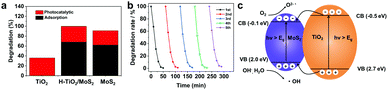 |
| Fig. 5 (a) Adsorption of RhB in dark (30 min) and photocatalytic degradation of RhB under the light irradiation (40 min) with TiO2 nanoparticles, H-TiO2/MoS2 and MoS2 flowers. (b) The recycling stability and (c) schematic photocatalytic degradation principle of H-TiO2/MoS2. | |
Additionally, as illustrated in Fig. S6 (ESI†), the consumed time for degradation decreased as the pH value increased. Specifically, it only took 20 min for RhB degradation at pH = 3, while it took ∼50 min for RhB degradation at pH = 6.9 and 8.9. This indicated that the degradation of RhB was easier in acid solution.
The schematic diagram (Fig. 5c) illustrates the energy band structure of H-TiO2/MoS2 and the process of electron transfer and the formation process of reactive oxygen species. Generally, the band gap of TiO2 (anatase) was relatively wide (∼3.2 eV), while the band gap of MoS2 was narrower (∼1.8 eV).39 Upon light illumination, electrons could be excited from the valence band (VB) of MoS2 to the conduction band (CB), leaving holes in the VB. Compared with TiO2, it was easy to induce photogenerated electrons in MoS2 with a relatively lower CB, and the photo-induced electrons (CB, MoS2) could rapidly transport to TiO2 nanoparticles (CB). The Mott–Schottky test was carried out to determine the flat-band potential of H-TiO2/MoS2.40 As illustrated in Fig. S7 (ESI†), the potential can be confirmed to be ∼−0.47 V (vs. SCE). The corresponding potential was converted to 0.18 V (vs. RHE) according to the equation E (vs. RHE) = E (vs. SCE) + 0.0591 pH + 0.244 V. Owing to dissolved oxygen in solution, photo-induced electrons could form superoxide radical anions from trapped O2 (O2 + e− → O2−˙), O2−˙, as a high activity intermediate, usually used to degrade organic pollutants. On the valence band, the leaving holes were transferred from TiO2 to MoS2 due to the higher VB, H2O was oxidized into hydroxyl radicals by holes with strong reduction (H2O + h+ → ˙OH), which could oxidize organic dye into CO2 and H2O, etc.41 The active species generated in the process of photodegradation were h+, radical O2˙ and radical ˙OH.42 In order to reveal the main active species that played the significant role in the photodegradation of RhB, the free radical scavenging experiments were conducted. In detail, free radical scavengers of isopropanol, dimethylsulfoxide (DMSO) and t-BuOH were added to the photodegradation system as a h+ trapping agent, a radical O2˙− trapping agent and a radical OH˙ trapping agent, respectively. As illustrated in Fig. S8 (ESI†), the photodegradation efficiency of RhB without adding a trapping agent was 94.7%, and the degradation efficiencies after adding isopropanol, t-BuOH and DMSO were 97.0%, 78.5% and 25.4%, respectively. The photodegradation performance of RhB was inhibited notably after adding DMSO, which confirmed that radical O2˙− was the main active species used for oxidation and h+ played a synergistic role in the photocatalytic reaction,43 and radical ˙OH indicated no evident effect.44 H-TiO2/MoS2 was just tapping into its strong charge separation ability and weak charge–hole recombination ability for enhancing the photocatalytic degradation performance.
Conclusions
H-TiO2/MoS2 consisting of TiO2 as a hard core and MoS2 as a shell was prepared through a facile two-step solvothermal approach. H-TiO2/MoS2 was beneficial for fast electron transfer between TiO2 and MoS2 due to maximally exposed active edge sites of MoS2 and illustrated high structural stability. Moreover, H-TiO2/MoS2 indicated enhanced light absorption and improved performance in the photocatalytic degradation of RhB (99.4%). The synergistic effect between MoS2 nanosheets and TiO2 nanoparticles accounted for the outstanding photocatalytic degradation performance. Therefore, this novel photocatalyst is promising for preparing elaborate heterostructures and applications in various fields, such as sewage-treatment, dye degradation, etc.
Author contributions
Ping Li: data curation, writing – original draft, methodology, investigation. Mengyou Gao: supervision, writing – review and editing. Lei Sun: investigation, data curation. Huizhong Xu: data curation. Xiaochen Dong: methodology, investigation. Jianjian Lin: methodology, conceptualization, supervision, writing – review and editing.
Conflicts of interest
The authors declare that there are no conflicts to declare.
Acknowledgements
This work was partly supported by QUSTHX201919. J. Lin is supported by the Young Taishan Scholarship Project of Shandong Province (tsqn201909115).
Notes and references
- A.-Y. Zhang, W.-Y. Wang, J.-J. Chen, C. Liu, Q.-X. Li, X. Zhang, W.-W. Li, Y. Si and H.-Q. Yu, Epitaxial Facet Junctions on TiO2 Single Crystals for Efficient Photocatalytic Water Splitting, Energy Environ. Sci., 2018, 11, 1444–1448 RSC.
- W. Zhang, H. He, Y. Tian, K. Lan, Q. Liu, C. Wang, Y. Liu, A. Elzatahry, R. Che, W. Li and D. Zhao, Synthesis of Uniform Ordered Mesoporous TiO2 Microspheres with Controllable Phase Junctions for Efficient Solar Water Splitting, Chem. Sci., 2019, 10, 1664–1670 RSC.
- L. Mu, Y. Zhao, A. Li, S. Wang, Z. Wang, J. Yang, Y. Wang, T. Liu, R. Chen, J. Zhu, F. Fan, R. Li and C. Li, Enhancing Charge Separation on High Symmetry SrTiO3 Exposed with Anisotropic Facets for Photocatalytic Water Splitting, Energy Environ. Sci., 2016, 9, 2463–2469 RSC.
- L. Ai, R. Shi, J. Yang, K. Zhang, T. Zhang and S. Lu, Efficient Combination of g-C3N4 and CDs for Enhanced Photocatalytic Performance: A Review of Synthesis, Strategies, and Applications, Small, 2021, 2007523 CrossRef CAS PubMed.
- L. Wang, J. Wan, Y. Zhao, N. Yang and D. Wang, Hollow Multi-Shelled Structures of Co3O4 Dodecahedron with Unique Crystal Orientation for Enhanced Photocatalytic CO2 Reduction, J. Am. Chem. Soc., 2019, 141, 2238–2241 CrossRef CAS PubMed.
- R. Shen, D. Ren, Y. Ding, Y. Guan, Y. H. Ng, P. Zhang and X. Li, Nanostructured CdS for Efficient Photocatalytic H2 Evolution: A Review, Sci. China Mater., 2020, 63, 2153–2188 CrossRef CAS.
- J. Lin, P. Li, H. Xu, Y. Kim, Z. Jing and D. Zheng, Controlled Synthesis of Mesoporous Single-Crystalline TiO2 Nanoparticles for Efficient Photocatalytic H2 Evolution, J. Hazard. Mater., 2020, 391, 122530 CrossRef CAS PubMed.
- P. Petrisková, O. Monfort, L. Satrapinskyy, E. Dobročka, T. Plecenik, G. Plesch, R. Papšík, R. Bermejo and Z. Lenčéš, Preparation and Photocatalytic Activity of TiO2 Nanotube Arrays Prepared on Transparent Spinel Substrate, Ceram. Int., 2021, 47, 12970–12980 CrossRef.
- X. Tao, P. Ruan, X. Zhang, H. Sun and X. Zhou, Microsphere Assembly of TiO2 Mesoporous Nanosheets with Highly Exposed (101) Facets and Application in a Light-Trapping Quasi-Solid-State Dye-Sensitized Solar Cell, Nanoscale, 2015, 7, 3539–3547 RSC.
- Z. Jiang, W. Wei, D. Mao, C. Chen, Y. Shi, X. Lv and J. Xie, Silver-Loaded Nitrogen-Doped Yolk-Shell Mesoporous TiO2 Hollow Microspheres with Enhanced Visible Light Photocatalytic Activity, Nanoscale, 2015, 7, 784–797 RSC.
- Y. Liu, K. Lan, S. Li, Y. Liu, B. Kong, G. Wang, P. Zhang, R. Wang, H. He, Y. Ling, A. M. Al-Enizi, A. A. Elzatahry, Y. Cao, G. Chen and D. Zhao, Constructing Three-Dimensional Mesoporous Bouquet-Posy-like TiO2 Superstructures with Radially Oriented Mesochannels and Single-Crystal Walls, J. Am. Chem. Soc., 2017, 139, 517–526 CrossRef CAS PubMed.
- X. Li, W. Li, M. Li, P. Cui, D. Chen, T. Gengenbach, L. Chu, H. Liu and G. Song, Glucose-Assisted Synthesis of the Hierarchical TiO2 Nanowire@MoS2 Nanosheet Nanocomposite and Its Synergistic Lithium Storage Performance, J. Mater. Chem. A, 2015, 3, 2762–2769 RSC.
- Y. Sun, H. Lin, C. Wang, Q. Wu, X. Wang and M. Yang, Morphology-Controlled Synthesis of TiO2/MoS2 Nanocomposites with Enhanced Visible-Light Photocatalytic Activity, Inorg. Chem. Front., 2018, 5, 145–152 RSC.
- Q. Wang, P. Yu, L. Bai, R. Bao, N. Wang, C. Cheng, Z. Liu, M. Yang, W. Yang and Z. Guo, Self-Assembled Nano-Leaf/Vein Bionic Structure of TiO2/MoS2 Composites for Photoelectric Sensors, Nanoscale, 2017, 9, 18194–18201 RSC.
- R. Dai, A. Zhang, Z. Pan, A. M. Al-Enizi, A. A. Elzatahry, L. Hu and G. Zheng, Epitaxial Growth of Lattice-Mismatched Core-Shell TiO2@MoS2 for Enhanced Lithium-Ion Storage, Small, 2016, 12, 2792–2799 CrossRef CAS PubMed.
- J. Liang, C. Wang, P. Zhao, Y. Wang, L. Ma, G. Zhu, Y. Hu, Z. Lu, Z. Xu, Y. Ma, T. Chen, Z. Tie, J. Liu and Z. Jin, Interface Engineering of Anchored Ultrathin TiO2/MoS2 Heterolayers for Highly-Efficient Electrochemical Hydrogen Production, ACS Appl. Mater. Interfaces, 2018, 10, 6084–6089 CrossRef CAS PubMed.
- Q. Xiang, J. Yu and M. Jaroniec, Synergetic Effect of MoS2 and Graphene as Cocatalysts for Enhanced Photocatalytic H2 Production Activity of TiO2 Nanoparticles, J. Am. Chem. Soc., 2012, 134, 6575–6578 CrossRef CAS PubMed.
- L. Zheng, S. Han, H. Liu, P. Yu and X. Fang, Hierarchical MoS2 Nanosheet@TiO2 Nanotube Array Composites with Enhanced Photocatalytic and Photocurrent Performances, Small, 2016, 12, 1527–1536 CrossRef CAS PubMed.
- B. Chen, Y. Meng, J. Sha, C. Zhong, W. Hu and N. Zhao, Preparation of MoS2/TiO2 based Nanocomposites for Photocatalysis and Rechargeable Batteries: Progress, Challenges, and Perspective, Nanoscale, 2017, 10, 34–68 RSC.
- J. Zheng, X. Yan, Z. Lu, H. Qiu, G. Xu, X. Zhou, P. Wang, X. Pan, K. Liu and L. Jiao, High-Mobility Multilayered MoS2 Flakes with Low Contact Resistance Grown by Chemical Vapor Deposition, Adv. Mater., 2017, 29, 1604540 CrossRef PubMed.
- T.-Y. Chen, Y.-H. Chang, C.-L. Hsu, K.-H. Wei, C.-Y. Chiang and L.-J. Li, Comparative Study on MoS2 and WS2 for Electrocatalytic Water Splitting, Int. J. Hydrogen Energy, 2013, 38, 12302–12309 CrossRef CAS.
- Q. Pang, Y. Zhao, X. Bian, Y. Ju, X. Wang, Y. Wei, B. Liu, F. Du, C. Wang and G. Chen, Hybrid Graphene@MoS2@TiO2 Microspheres for Use as a High Performance Negative Electrode Material for Lithium Ion Batteries, J. Mater. Chem. A, 2017, 5, 3667–3674 RSC.
- Y. Liu, H. Niu, W. Gu, X. Cai, B. Mao, D. Li and W. Shi, In situ Construction of Hierarchical CdS/MoS2 Microboxes for Enhanced Visible-Light Photocatalytic H2 Production, Chem. Eng. J., 2018, 339, 117–124 CrossRef CAS.
- L. Zhao, T. Dong, J. Du, H. Liu, H. Yuan, Y. Wang, J. Jia, H. Liu and W. Zhou, Synthesis of CdS/MoS2 Nanooctahedrons Heterostructure with a Tight Interface for Enhanced Photocatalytic H2 Evolution and Biomass Upgrading, Sol. RRL, 2021, 5, 2000415 CrossRef CAS.
- M. Yang, L. Wang, G. Hu, X. Chen, P. L. Gong, X. Cong, Y. Liu, Y. Yang, X. Li, X. Zhao and X. Liu, Optical Identification of Interlayer Coupling of Graphene/MoS2 van der Waals Heterostructures, Nano Res., 2021, 14, 2241–2246 CrossRef CAS.
- W. Zhou, K. Zhou, D. Hou, X. Liu, G. Li, Y. Sang, H. Liu, L. Li and S. Chen, Three-Dimensional Hierarchical Frameworks Based on MoS2 Nanosheets Self-Assembled on Graphene Oxide for Efficient Electrocatalytic Hydrogen Evolution, ACS Appl. Mater. Interfaces, 2014, 6, 21534–21540 CrossRef CAS PubMed.
- X. Liu, Z. Xing, H. Zhang, W. Wang, Y. Zhang, Z. Li, X. Wu, X. Yu and W. Zhou, Fabrication of 3D Mesoporous Black TiO2/MoS2/TiO2 Nanosheets for Visible-Light-Driven Photocatalysis, ChemSusChem, 2016, 9, 1118–1124 CrossRef CAS PubMed.
- W. Zhou, Z. Yin, Y. Du, X. Huang, Z. Zeng, Z. Fan, H. Liu, J. Wang and H. Zhang, Synthesis of Few-Layer MoS2 Nanosheet-Coated TiO2 Nanobelt Heterostructures for Enhanced Photocatalytic Activities, Small, 2013, 9, 140–147 CrossRef CAS PubMed.
- Y. Yuan, Z. Ye, H. Lu, B. Hu, Y. Li, D. Chen, J. Zhong, Z. Yu and Z. Zou, Constructing Anatase TiO2 Nanosheets with Exposed (001) Facets/Layered MoS2 Two-Dimensional Nanojunctions for Enhanced Solar Hydrogen Generation, ACS Catal., 2015, 6, 532–541 CrossRef.
- K. He, Q. Wen, C. Wang, B. Wang, S. Yu, C. Hao and K. Chen, A Facile Synthesis of Hierarchical Flower-Like TiO2 Wrapped with MoS2 Sheets Nanostructure for Enhanced Electrorheological Activity, Chem. Eng. J., 2018, 349, 416–427 CrossRef CAS.
- J. Lin, L. Zhao, Y. Heo, L. Wang, F. Bijarbooneh, A. Mozer, A. Nattestad, Y. Yamauchi, S. Dou and J. Kim, Mesoporous Anatase Single Crystals for Efficient Co(2+/3+)-Based Dye-Sensitized Solar Cells, Nano Energy, 2015, 11, 557–567 CrossRef CAS.
- K. Chang and W. Chen, L-Cysteine-Assisted Synthesis of Layered MoS2/Graphene Composites with Excellent Electrochemical Performances for Lithium Ion Batteries, ACS Nano, 2011, 5, 4720–4728 CrossRef CAS PubMed.
- Q. Zhou, W. Li, M. Gao, H. Xu, Y. Guo, L. Sun, D. Zheng and J. Lin, A. Truncated Octahedron Metal-Organic Framework Derived TiO2@C@MoS2 Composite with Superior Lithium-Ion Storage Properties, J. Power Sources, 2022, 518, 230746 CrossRef CAS.
- J. Yang, L. Yu, B. Zheng, N. Li, J. Xi and X. Qiu, Carbon Microtube Textile with MoS2 Nanosheets Grown on Both Outer and Inner Walls as Multifunctional Interlayer for Lithium–Sulfur Batteries, Adv. Sci., 2020, 7, 1903260 CrossRef CAS PubMed.
- S. Wang, B. Guan, L. Yu and X. (David), Lou, Rational Design of Three-Layered TiO2@Carbon@MoS2 Hierarchical Nanotubes for Enhanced Lithium Storage, Adv. Mater., 2017, 29, 1702724 CrossRef PubMed.
- J. Pei, H. Geng, E. H. Ang, L. Zhang, X. Cao, J. Zheng and H. Gu, Controlled Synthesis of Hollow C@TiO2@MoS2 Hierarchical Nanospheres for High-Performance Lithium-Ion Batteries, Nanoscale, 2018, 10, 17327–17334 RSC.
- B. Guo, K. Yu, H. Fu, Q. Hua, R. Qi, H. Li, H. Song, S. Guo and Z. Zhu, Firework-Shaped TiO2 Microspheres Embedded with Few-Layer MoS2 as an Anode Material for Excellent Performance Lithium-Ion Batteries, J. Mater. Chem. A, 2015, 3, 6392–6401 RSC.
- S. V. P. Vattikuti and C. Byon, Synthesis and Characterization of Molybdenum Disulfide Nanoflowers and Nanosheets: Nanotribology, J. Nanomater., 2015, 2015, 1–11 Search PubMed.
- X. Hu, H. Zhao, J. Tian, J. Gao, Y. Li and H. Cui, Synthesis of Few-Layer MoS2 Nanosheets-Coated TiO2 Nanosheets on Graphite Fibers for Enhanced Photocatalytic Properties, Sol. Energy Mater. Sol. Cells, 2017, 172, 108–116 CrossRef CAS.
- Q. Zhang, Y. Wang, X. Zhu, X. Liu and H. Li, 1T and 2H Mixed Phase MoS2 Nanobelts Coupled with Ti3+ Self-Doped TiO2 Nanosheets for Enhanced Photocatalytic Degradation of RhB under Visible Light, Appl. Surf. Sci., 2021, 556, 149768 CrossRef CAS.
- H. Yan, L. Liu, R. Wang, W. Zhu, X. Ren, L. Luo, X. Zhang, S. Luo, X. Ai and J. Wang, Binary Composite MoS2/TiO2 Nanotube Arrays as a Recyclable and Efficient Photocatalyst for Solar Water Disinfection, Chem. Eng. J., 2020, 401, 126052 CrossRef CAS.
- Z. Li, F. Cao, L. Wang, Z. Chen and X. Ji, A Novel Ternary MoS2/MoO3/TiO2 Composite for Fast Photocatalytic Degradation of Rhodamine B under Visible-Light Irradiation, New J. Chem., 2020, 44, 537–542 RSC.
- D. Cao, Q. Wang, S. Zhu, X. Zhang, Y. Li, Y. Cui, Z. Xue and S. Gao, Hydrothermal Construction of Flower-Like MoS2 on TiO2 NTs for Highly Efficient Environmental Remediation and Photocatalytic Hydrogen Evolution, Sep. Purif. Technol., 2021, 265, 118463 CrossRef CAS.
- M. Mohamed and N. Karunakaran, One-Step Solvothermal Synthesis of Carbon Doped TiO2–MoS2 Heterostructure Composites with Improved Visible Light Catalytic Activity, New J. Chem., 2016, 40, 8123–8130 RSC.
Footnotes |
† Electronic supplementary information (ESI) available. See DOI: 10.1039/d1ma01050f |
‡ These authors equally contributed to this work |
|
This journal is © The Royal Society of Chemistry 2022 |
Click here to see how this site uses Cookies. View our privacy policy here.