DOI:
10.1039/D1MA01195B
(Paper)
Mater. Adv., 2022,
3, 2393-2404
Transition metal ion-coordinated porous organic polymer to enhance the peroxidase mimic activity for detection of ascorbic acid and dopamine†
Received
17th December 2021
, Accepted 14th January 2022
First published on 19th January 2022
Abstract
Porous organic polymers (POPs) have been fabricated with intriguing physicochemical properties, which can be used in a wide range of emerging applications. However, they show less activity in nanozymes. Attempts have been made to boost nanozyme activity with incorporation of nanoparticles into POPs. The importance of M2+ incorporation has been highlighted, which prevents leaching and agglomeration. In this study, we prepared transition metal ion-coordinated porous organic polymers (Cu2+@POP, Ni2+@POP and Co2+@POP) by a mechanochemical method. As-prepared Cu2+@POP, Ni2+@POP and Co2+@POP exhibited excellent 3,3′,5,5′-tetramethylbenzidine (TMB) oxidation reaction in the presence of H2O2. The oxidation reaction occurred by single electron transfer, to convert colorless TMB to blue colored ox-TMB. Furthermore, the peroxidase like activity of Cu2+@POP has been shown to potentially prevent TMB oxidation in the presence of ascorbic acid (AA) and dopamine (DA). Based on the inhibition effect, we achieved low detection limits of 1.4 mM and 1 mM for AA and DA through colorimetric method. To the best of our knowledge, for the first time we investigate colorimetric-based detection of both AA and DA. With these findings, Cu2+@POP has excellent catalytic activity, providing a promising prospect for biosensors and clinical fields.
Introduction
Natural enzymes are exclusive biologically active catalysts that have been utilized for comprehensive applications. Indeed, several types of mimic activities exist such as peroxidase-mimic, oxidase-mimic, catalyst-mimic, and superoxide-like activities. Among them, peroxidase-mimic activity has gained great attention due to promising properties. Peroxidases are kinds of enzymes, capable of catalyzing the oxidation of hydrogen peroxide into reactive oxygen species. Moreover, they can give good responsivity for visual detection. However, natural enzymes have certain limitations such as hydrolysis, denaturation, less stability, non-reusability, and stimuli sensitivity.1 In order to overcome the limitations of natural enzymes, nanomaterials have been shown to be preferable candidates. Previously, nanomaterials, functionalized nanomaterials, graphene oxide, graphitic carbon nitrile, carbon dots, metal–organic frameworks (MOFs), and covalent organic frameworks (COFs) were demonstrated as an alternative nanozymes.2–5 However, those are remarkable materials for peroxidase-like activity. Many researchers have attempted to enhance nanozyme activity with external stimuli like size, shape and surface charges and modification and compositions. Even though, they showed some limitations including less activity and stability, and high cost.6–8 Generally, incorporation of nanoparticles into various substrate materials sufficiently enhances the peroxidase-like activity. We aim to incorporate nanoparticles into substrate materials for enhancing the peroxidase-mimic activity. In the abovementioned reports, solvothermal method, ionothermal method, and wet impregnation method were used for Cu2+ incorporation in the porous materials. However, this required harsh reaction conditions and toxic solvents and was expensive. For example, reduced graphene oxide (rGO) and graphitic carbon nitrile (g-C3N4) have been used to efficiently enhance the peroxidase-like activity.9,10 For example, Willner et al. reported Cu2+-modified graphene oxide (Cu2+–GO) and Cu2+-modified g-C3N4 (Cu2+–g-C3N4), showing superior catalytic activity which was analyzed by chemiluminescence.11 Wang et al. described Cu2+-incorporated g-C3N4 having effective peroxidase-like activity, which was analyzed calorimetrically.12 Generally, porous materials have higher catalytic activity than non-porous materials due to the rich metal binding sites and high porosity.
Porous materials have multifocal advantages including affordability, stable lattice, easy storage, and multiple functionalities.13–16 However, they have some limitations including acid sensitivity, blockage of active sites by axial coordination in MOFs and active positions being inaccessible and diffusions in COFs.17,18 In order to overcome these limitations, copper-modified porous materials have been investigated, having enhanced peroxidase-like activity compared with unmodified porous materials.19 For instance, Li found that Cu2+-incorporated MOF (UiO-67-Cu2+) had remarkable lactase activity.20 Willner et al. have reported Cu2+-modified NMOF (Cu2+–NMOF) that exhibited sufficient peroxidase-mimic activity. Hence, they detected dopamine through chemiluminescence.21 Following that, Fanggui and coworkers documented a Cu2+-incorporated covalent triazine framework which oxidized 3,3′,5,5′-tetramethylbenzidine (TMB).22 According to pervious reports, Cu2+ incorporation in porous materials is an attractive way to enhance the catalytic activity.
Porous organic polymers (POPs) are well known for their unique physiochemical properties such as structural adaptively, predictability, good hydrothermal stability, large surface area, low density, and so on.23–26 POPs are promising materials for a wide range of applications including gas storage/separation, optoelectronics, organocatalysis, sensors, drug delivery, photocatalysis/photovoltaics.27–31 Owing to the aforementioned properties, POPs are suitable for oxidation reactions but show less peroxidase-like activity. To enhance the peroxidase-like activity of POPs, we intended to incorporate transition metals. In the abovementioned reports, solvothermal method, ionothermal method, and wet impregnation method were used for Cu2+ incorporation in porous materials. However, this required harsh reaction conditions and toxic solvents and was expensive. Recently, mechanochemical synthesis of POPs has received much attention because of it being solvent free and of short reaction duration. We envisioned the incorporation of transition metal ions in POPs by mechanochemical method.
Ascorbic acid (AA) is one of the essential vitamins for human health that also is regularly used as an artificial antioxidant for food ingredients in food industries.32 Large doses of AA function as pro-anticancer agent and specifically kill colorectal cancer cells.33 Thus, AA detection is of significance. Also, dopamine (DA) is one of the essential nutrients for the human bodies. Disordered levels of DA in the human body can lead to numerous diseases.34,35 Therefore, detection and removal of excess DA in living systems is essential. Up to now, detection of AA and DA has been successfully carried out with instrumentation techniques including fluorescence method, electrochemical method, colorimetric method, surface plasma resonance, electro- and chemiluminescence, high-performance liquid chromatography, gas chromatography, and mass spectrometry.36,37 Among them, the colorimetric method is of great interest due to its simplicity and specificity.
Herein, we fabricated transition metal-coordinated POPs (Ni2+@POP, Co2+@POP, and Cu2+@POP) through mechanochemical method. As-synthesized functional materials exhibited marked peroxidase-like activity in the presence of H2O2 and TMB. Cu2+@POP shows excellent catalytic activity compared with Ni2+@POP and Co2+@POP. In the presence of H2O2, TMB exhibited a strong absorption band at 652 nm as well as blue colour that corresponds to ox-TMB. The presence of AA and DA can inhibit oxidation of TMB and limit the colour change. This remarkable inhibition effect has been used to achieve low detection limits of 1.3 mM and 1 mM for AA and DA. However, pristine POP did not exhibit any catalyst activity without transition metal coordination. These phenomena allow facile colorimetric detection for AA and DA. To the best of our knowledge, for the first time we applied transition metal-coordinated POP for the dual detection of biomolecules.
Materials
Benzene-1,3,5-tricarbonyl trichloride (C9H3O3Cl3), benzene-1,4-diamine, triethylamine (TEA), methanol (CH3OH), sodium borohydride (NaBH4), hydrogen peroxide (H2O2), TMB, DA, glucose (G), fructose (Fr), cysteine (Cy), uric acid (UA), AA, p-benzoquinone (PBQ), isopropyl alcohol (IPA). All chemicals were purchased from Sigma Aldrich, SRL Chemicals and Avra Chemicals and used without further purification.
Characterizations
The morphology and crystalline structure were evaluated using scanning electron microscopy (SEM, FEI Quanta FEG 200) and high-resolution transmission electron microscopy (HRTEM, JEOL, JEM, Fb-2000). Powder X-ray diffraction (PXRD) was performed using a PANalytical X’pert Pro X-ray diffractometer with CuKα radiation. For bonding information studies, an Agilent Technologies FTIR spectrometer (USA) was used to record the Fourier transform infrared (FT-IR) spectra with the ATR mode. Thermal stability was investigated by thermogravimetric analysis (STA 2500 Thermoanalyzer).
Synthesis of POP
POP was synthesized with minor modifications according to an earlier report.38 Benzene-1,4-diamine (0.7332 g) and TEA (1.9057 g) were dissolved in 30 ml of DCM in a 100 ml round bottom flask. Benzene-1,3,5-tricarbonyl trichloride (1.0 g) dissolved in 30 ml of DCM was added under ice-cold conditions to the reaction mixture. It is interesting to note that with a few drops of C9H3O3Cl3 added into reaction mixture, immediately a semi-solid was formed. The reaction was continued for a further 12 hours for complete conversion of POP. The obtained solid was washed with distilled water followed by methanol and acetone several times. The obtained material was dried overnight at room temperature and, finally, olive-colored POP was collected.
Synthesis of transition metal-coordinated POP (M2+@POP)
The M2+@POP systematic synthetic scheme is depicted in Scheme 1. Typically, 100 mg of prepared POP was placed in a mortar. Subsequently, 5 mg of metal precursor (Ni(OAc)2, or Co(OAc)2, or Cu(OAc)2) with a drop of TEA was added. The mixture was ground for 15 minutes and the olive color changed to purple color for Cu2+ ions. Moreover, the obtained solid was washed with distilled water several times. Further, the product was dried at 60 °C in a hot-air oven for 12 hours. Finally, the transition metal-coordinated POPs (Ni2+@POP, Co2+@POP and Cu2+@POP) were collected.
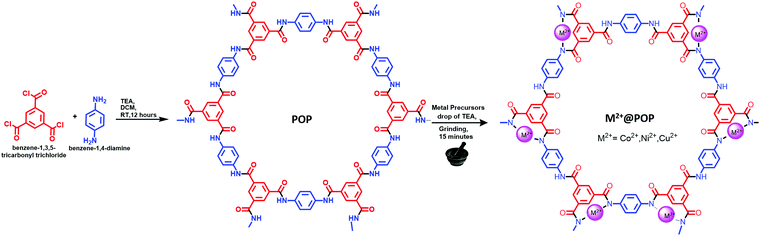 |
| Scheme 1 Schematic illustration for synthesis of M2+@POP. | |
Results and discussion
Herein, M2+@POPs were synthesized by a mechanochemical method. The M2+@POP synthetic route is systematically depicted in Scheme 1. For metal ion incorporation, microwave, solvothermal, and ion-thermal methods were developed.39,40 Typically, benzene-1,3,5-tricarbonyl trichloride and benzene-1,4-diamine have been coordinated in the presence of TEA at room temperature. Hence, POP was formed through nucleophile addition/elimination reaction. Subsequently, Ni2+, Co2+, Cu2+ and POP were ground mechanochemically. Thereby, transition metal ions were coordinated to POP, and thus Cu2+@POP, Ni2+@POP and Co2+@POP were synthesized.
Initially, the synthesized Ni2+@POP, Co2+@POP, and Cu2+@POP morphologies were ascertained by SEM and TEM imaging and the results are shown in Fig. 1. As seen in Fig. 1a, the obtained POPs have sphere-like morphology and are agglomerated. Also, SEM mapping and EDS analysis confirmed the existence of C, N, and O elements (Fig. S2 and S7a, ESI†).41 Moreover, TEM images also clearly indicate the as-prepared POPs have a spherical shape and polymeric nature, as displayed in Fig. 1b and Fig. S1 (ESI†). SEM images of the Cu2+-coordinated POP (Cu2+@POP) exhibited a slightly collapsed sphere-like morphology (Fig. 1c and d). It is interesting to note that the collapsed spherical shape is due to coordination with Cu2+ ions. As well, SEM mapping and EDS undoubtedly confirmed C, N, O, and Cu2+ elements were present (Fig. S3 and S7b, ESI†). Moreover, TEM images also ascertained a collapse in spherical shape, mainly due to incorporation Cu2+ in the POP. The HRTEM images show small particles spread over the POP as displayed in Fig. 1e and f. Similarly, SEM images of Ni2+@POP and Co2+@POP are shown in Fig. S4a and S53b (ESI†). EDS and SEM mapping undoubtedly confirmed the existence of C, N, O, and Ni2+ elements for Ni2+@POP, and C, N, O, and Co2+ elements for Co2+@POP (Fig. S4, S5 and S7, ESI†).
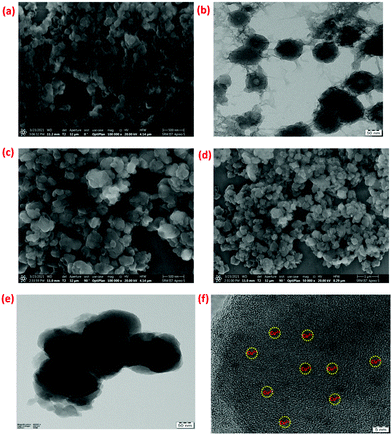 |
| Fig. 1 (a) SEM image of POP; (b) TEM image of POP; (c and d) SEM images of Cu2+@POP; and (e and f) TEM images of Cu2+@POP. | |
Following the morphological study, prepared POP, Ni2+@POP, Co2+@POP, and Cu2+@POP were analyzed by FT-IR spectroscopy, and the results are displayed in Fig. 2. Typically, absorption bands appeared at 1645 cm−1 and 1661 cm−1 associated with amide C
O stretching frequency (amide-I band). The N–H band was observed at 1511 cm−1 corresponding to bending frequency of amide-II band; at the same time N–H and C–N bonding interaction bands presented at 1252 and 1312 cm−1. Notably, a broad band appeared at 3307 cm−1 associated with N–H stretching frequency, this result revealing POP had been formed. Notably, 701 cm−1 peak was not observed that corresponds to acid chloride, which emphasizes that acid chloride was completely converted to POP and this well matched with an earlier report.38 After confirming the formation of POP, transition metal ions (M = Ni2+, Co2+, Cu2+) were coordinated with POP through a mechanochemical method. As a result, the N–H bending band was drastically reduced, that reduction being due to the coordination between Ni2+, Co2+ and Cu2+ and electron-rich nitrogen group of POP. The FT-IR results clearly indicate the formation of POP and Ni2+@POP, Co2+@POP, and Cu2+@POP.
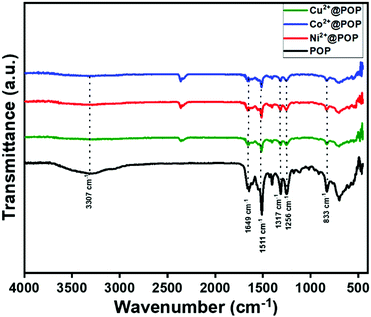 |
| Fig. 2 FT-IR spectra of POP, Ni2+@POP, Co2+@POP, and Cu2+@POP. | |
Following the FT-IR analysis, the as-synthesized materials (POP, Ni2+@POP, Co2+@POP, and Cu2+@POP) were investigated by PXRD as displayed in Fig. 3. As depicted in Fig. 3, 2θ peaks were located at 28° and 43°, which are associated with repeating units and π–π interaction. This result indicates that amorphous POP was formed. Moreover, with transition metal ion incorporation into POP, discrete diffraction peaks at 44.3° corresponding to 111 plane of metallic peaks for Ni2+@POP, Co2+@POP, and Cu2+@POP were not detected. Interestingly, repeating units were prominent due to the coordination with metal ions. This result reveals successful coordination between amine groups of POP and metal ions. In addition, note that Cu0 and CuxO are not observed by this method; also, Cu2+ is dominant in Cu2+@POP.22 Additionally, Cu2+ presence was clarified by SEM mapping and UV-visible spectra, as we discuss in more detail below. According to the diffraction result, the formation of amide-functionalized POP and Cu2+@POP, Ni2+@POP, and Co2+@POP was confirmed.
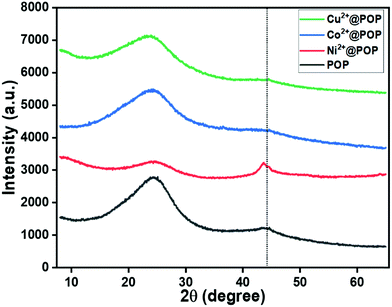 |
| Fig. 3 PXRD patterns of POP, Ni2+@POP, Co2+@POP, and Cu2+@POP. | |
UV-visible spectra were examined to explore the optical absorption properties of prepared POP, Ni2+@POP, Co2+@POP, and Cu2+@POP. As shown in Fig. 4a, the spectrum of pristine POP had a characteristic absorption band positioned at 350 nm due to the n–π* transition of amide group of POP. However, the n–π* transition band shifted to 371, 372 and 379 nm for Ni2+@POP, Co2+@POP, and Cu2+@POP, respectively. The shift reveals coordination between POP and Co2+, Ni2+, and Cu2+. These results confirmed that Co2+, Ni2+, and Cu2+ had been successfully coordinated with POP. Subsequently, the thermal stability of obtained POP, Ni2+@POP, Co2+@POP, and Cu2+@POP was appraised by TGA measurement, the results being shown in Fig. 4b. Initially, loss of surface-adsorbed H2O showed a weight decrease up to 7%, 5%, 3% and 5% for POP, Ni2+@POP, Co2+@POP, and Cu2+@POP, respectively. As-prepared POP had sustainable thermal stability up to 300 °C; coordination with corresponding metal ions increased the stability to more than 350 °C for Ni2+@POP, Co2+@POP, and Cu2+@POP. The TGA results also clearly indicated that transition metal ions successfully coordinated with POP and the resulting materials have excellent thermal stability.
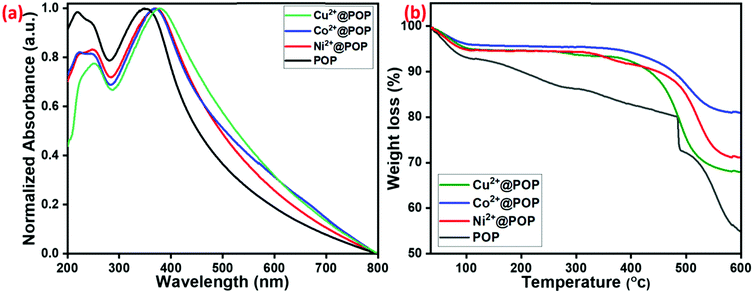 |
| Fig. 4 (a) UV-visible spectra and (b) TGA curves of POP, Ni2+@POP, Co2+@POP, and Cu2+@POP. | |
Furthermore, chemical composition and oxidation state of POP and Cu2+@POP were extensively studied by X-ray photoemission spectroscopy (XPS). The wide range spectra clearly indicate C, N, and O for POP22 and Cu, C, N, and O for Cu2+@POP, as shown in Fig. 5a. As seen in Fig. 5b, showing the high-resolution spectrum of Cu2+ with deconvolution, Cu2+ shows exquisite major characteristic peaks at 933.5, 935.5 and 953.6 eV.42 These results confirmed that Cu2+ was successfully coordinated into POP via mechanochemical method. Furthermore, the high-resolution spectra of N 1s and O 1s clearly indicate coordination between Cu2+ and amine group of POP.43 In the N 1s deconvoluted spectrum of POP, peaks appeared at 399.7 and 400.2 eV corresponding to C–N and free N–H as shown in Fig. 5c. It is interesting to note that for POP after coordination with Cu2+, a new peak appeared at 405.5 eV associated with N–Cu coordination. From this observation, we confirmed the N atom coordinated with Cu2+ to form a Cu2+-incorporated POP. Likewise, O 1s spectra indicate non-covalent interaction. In the high-resolution O 1s spectrum, peaks appeared at 531.3, 532.4, and 533.2 eV which are associated with C
O, C–O and adsorbed O2. Subsequently, after coordination with Cu2+, these slightly shifted to 531.6, 532.7 and 533.5 eV, emphasizing oxygen atom was involved though non-covalent interaction (Fig. 5d). The C 1s spectra are shown in Fig. S3 (ESI†). According to these XPS spectra, we concluded that Cu2+ was coordinated with POP.
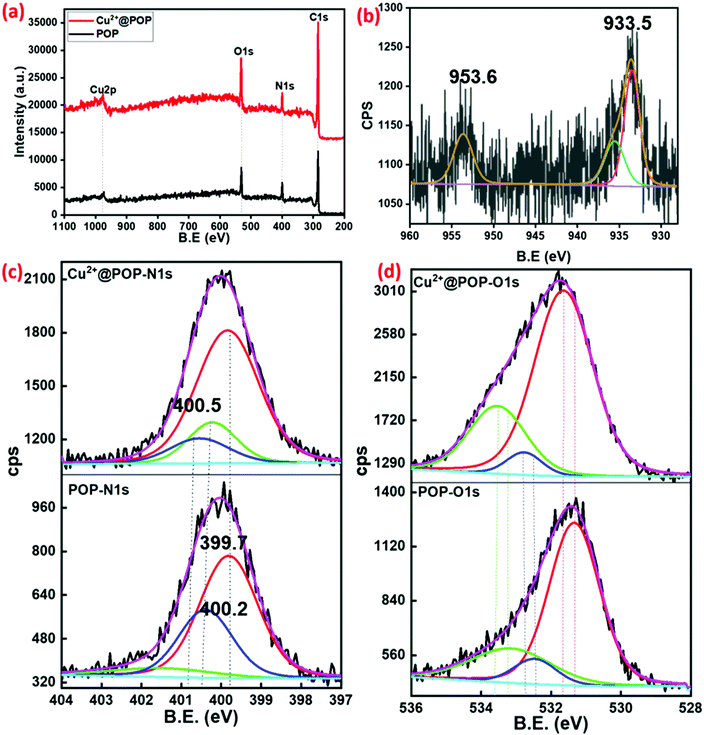 |
| Fig. 5 (a) Survey spectra of prepared POP and Cu2+@POP. (b) High-resolution spectrum of Cu2p of Cu2+@POP. (c and d) Deconvoluted N 1s and O 1s spectra before and after coordination with Cu2+. | |
Investigation of a peroxidase-like activity of M2+@POP
Horseradish peroxidase (HRP)-like activity is naturally found for enzymes extracted from plants, which are widely used in various applications such as biosensors (detection of H2O2, glucose, DNA, and RNA), biocatalysis and cancer treatment, even though HRP has some limitations. To replace HRP, prepared POP, Cu2+@POP, Ni2+@POP and Co2+@POP have been employed for their peroxidase-like activity. The oxidation reaction analyzed was that of TMB to ox-TMB in the presence of H2O2, as illustrated in Fig. 6a. The oxidation of TMB is mainly of three types: one-electron transfer, two-electron transfer and mixture of one- and two-electron transfer process. Consequently, one can obtain a blue, green and yellow color, respectively. For example, NPs catalyze the oxidation reaction of TMB in the presence of H2O2. Thus, POP, Cu2+@POP, Ni2+@POP and Co2+@POP were investigated for their peroxidase-mimicking function. As seen in Fig. 6a, the mixture of TMB, H2O2 and Cu2+@POP gives intense blue color as well as a characteristic absorption peak appearing at 652 nm, which corresponds to oxidation of TMB (ox-TMB). In contrast, TMB/H2O2, Cu2+@POP/TMB and Cu2+@POP/H2O2 do not exhibit any color change or absorption peak. When the concentration of H2O2 is increased, the absorption peak is intensified. According to the result, Cu2+@POP operated thorough the one-electron transfer process. Moreover, the optical photographs too clearly displayed color appearing for Cu2+@POP in the presence of TMB and H2O2 solution (inset of Fig. 6a). According to the XPS result, Cu2+@POP has a trinuclear copper center that contains Cu2+ and Cu+ in two oxidation states. This particular type of copper center is similar to that of Cu2+–Cu2+ analogues. During the oxidation of TMB, Cu2+–Cu2+ was reduced to Cu+–Cu+, so that Cu2+@POP oxidized TMB in the presence of H2O2. Moreover, Cu2+ atoms were uniformly dispersed in Cu2+@POP, which was not the case for Cu0 and CuxO. Impressively, Cu2+@POP showed a high peroxidase-mimic activity compared to POP, Ni2+@POP and Co2+@POP. The oxidation of TMB by Cu2+@POP can produce a blue color corresponding to the single-electron transfer process. According to that, Cu2+@POP shows higher peroxidase-mimic activity compared with POP, Ni2+@POP and Co2+@POP (Fig. 6b). Among them, Cu2+@POP displayed a more intense color than Ni2+@POP and Co2+@POP (optical images shown in the inset of Fig. 6b). From this result, we confirmed the prepared Cu2+@POP has superior catalytic activity.
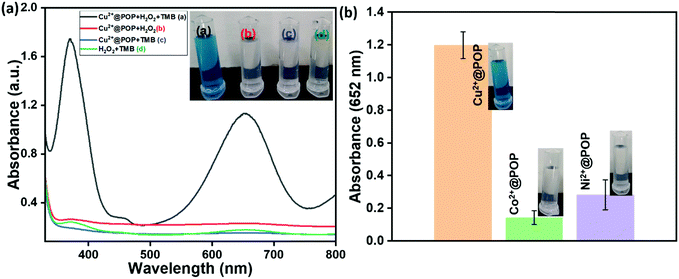 |
| Fig. 6 (a) UV-visible spectra of Cu2+@POP for oxidation of TMB in the presence of H2O2 and optical image shown as inset. (b) Oxidation efficiency of TMB with Cu2+@POP, Co2+@POP and Ni2+@POP, with color changes shown in inset optical images. | |
Optimization conditions
Peroxidase-like activity of Cu2+@POP was exhibited as characteristic absorption peaks as well as naked-eye color change. Moreover, some external factors affected catalytic activity, including pH, time, and concentration of TMB/Cu2+@POP. Hence, optimized pH was found via use of constant quantity of H2O2, TMB and Cu2+@POP at different pH (3 to 10), from which respective absorption peaks with intense blue color appeared. Among them, pH = 4 gave the best catalytic activity for oxidation of TMB (Fig. 7a) and also a digital image clearly shows the color change depending on the pH (inset of Fig. 7a). Then, the time-dependent oxidation process was investigated, as displayed in Fig. 7b. We also estimated the concentration of Cu2+@POP for oxidation of TMB at optimized pH and time. As seen in Fig. 7c, 10–100 μg of Cu2+@POP was used for the oxidation of TMB, other parameters kept constant. The time-dependent absorption intensity was recorded at 652 nm. The intensity of the 652 nm peak gradually increased up to 100 μg of Cu2+@POP. Hence, 100 μg of Cu2+@POP showed the best catalytic activity. The same procedure as mentioned above was followed for TMB optimization with various concentrations; according to this result, 90 mM TMB exhibited the highest activity (Fig. 7d).
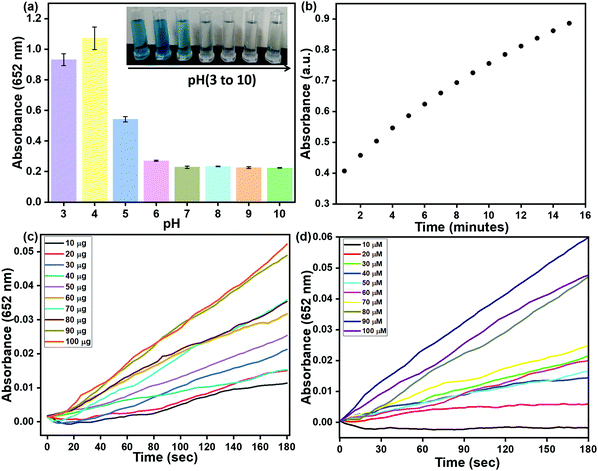 |
| Fig. 7 Optimization experiments: (a) pH; (b) time-dependent absorption intensity; (c) different concentrations of TMB; (d) various quantities of Cu2+@POP. | |
Kinetics study
To quantify the peroxidase-like activity of Cu2+@POP, a steady-state kinetic study via catalytic behavior with TMB and H2O2 was employed. The Michaelis–Menten curves obtained concentration of TMB and H2O2 as a substrate. Moreover, Michaelis–Menten curves (Fig. S7a and c, ESI†) and Lineweaver–Burk plots (Fig. S7b and d, ESI†) could be obtained for TMB and H2O2 within certain ranges of concentration, respectively. Then, we fitted the Lineweaver–Burk plot to calculate the Michaelis–Menten constant (Km) and maximal initial velocity (V) of the catalytic reaction involving Cu–HMT. The Km value of Cu2+@POP for the substrate H2O2 and TMB was 1.1343 mM and 0.79 mM. Moreover, the calculated Vmax of these two systems were 214.8 × 108 M−1 s and 3.5 × 108 M−1 s, respectively. According to the results, we conclude that as-prepared Cu–HMT has superior binding affinity towards H2O2 and TMB that enhanced the peroxidase-like activity.
To further investigate the catalytic mechanism of Cu2+@POP-induced peroxidase-like activity, a series of scavengers were used to scavenge the possible reactive oxygen species (ROS) during the catalytic reaction, including hydroxyl radicals (˙OH), superoxide anions (˙O2−), and holes (h+). By adding IPA that scavenges hydroxyl radicals (˙OH), no obvious color change or characteristic absorption band at 652 nm was seen, which reveals that ˙OH is not a major contributing ROS for oxidation of TMB. The result is shown in Fig. 8a. The catalytic activity was not obviously inhibited by EDTA, indicating that holes (h+) also are not major contributors. Addition of p-benzoquinone to ox-TMB solution exhibits an apparent inhibition effect on oxidation of TMB, confirming that superoxide anions (˙O2−) are ROS responsible for the oxidation TMB. The NBT analysis, which have absorbance band exhibited at 550 nm during the in the presence of riboflavin and light (control). Our case addition of Cu2+@POP and H2O2 existence of absorption band at 550 nm in the presence of NBT presence. Bare NBT exhibits an absorption band with low intensity. After incubation of Cu2+@POP and H2O2, the absorption band at 550 nm has increased because of production of superoxide ions (˙O2−). According to Fig. 8b, superoxide ions (˙O2−) are confirmed as the major ROS for oxidation of TMB. There appeared no reduction peak for a bare glass carbon electrode. After modifying with Cu2+@POP, the electrode shows a strong reduction peak at −0.5 eV, which verified the reduction of H2O2, similar to a previous report. This result emphasizes the peroxidase-mimic reaction through superoxide. The results reveal the ability of Cu2+@POP to transfer electrons between electron donor and acceptor, referring to electrode surface and H2O2, respectively (Fig. S10, ESI†).
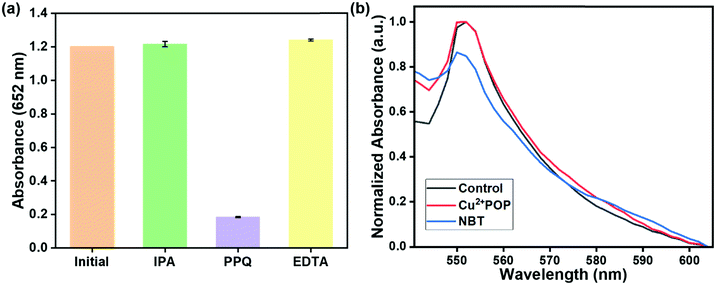 |
| Fig. 8 (a) Scavenging experiment for ROS. (b) NBT for SOD analysis, which has an absorption band at 550 nm in the presence of riboflavin and light (control). Bare NBT has an absorption band with low intensity. NBT has a strong absorption band at 550 nm in the presence of Cu2+@POP and H2O2 (Cu2+@POP). The absorption band at 550 nm has increased because of production of superoxide ions (˙O2−). | |
Detection of H2O2 and AA
The determination of H2O2 was analyzed by oxidation of TMB with synthesized Cu2+@POP, as shown in Fig. S8 (ESI†). As mentioned above, the optimum reaction conditions for H2O2 analysis were found from the above mentioned experiments. With increasing concentration of Cu2+@POP and TMB in the presence of different concentrations of H2O2, there is a dramatically increased absorbance at 652 nm; hence absorbance was directly proportional to the concentration. The ROS produced can oxidize TMB which turns into a blue color. Moreover, the absorption band and color emphasize the one-electron transfer process. The intensity of the absorption band at 652 nm changes relative to the concentration of H2O2 (Fig. S8 (ESI†) insert shows optical images of different concentrations of H2O2 in the reaction system). Besides, the peroxidase-like activity of Cu2+@POP and the inhibition of peroxidase-like activity in the presence of AA and DA were investigated. Fig. 9a schematically illustrates Cu2+@POP catalyzing the conversion of AA to dehydroascorbic acid with inhibition effect. Remarkably, strong blue-colored ox-TMB is evidenced with an absorption band at 652 nm without AA. Meanwhile, with the addition of AA into the system, immediately the deep color disappeared. Such a color fade indicates the inhibition of oxidation of TMB. Hence, varying the concentration of AA (1–17.5 mM) gradually decreased the absorption spectrum intensity as well as weakening the color. As illustrated in Fig. 9b, the strong absorption band at 652 nm exhibits a hypochromic shift with increasing concentration of AA. After 8 mM of AA, the absorption peak showed steady-state equilibrium. In Fig. 9c, the relative absorption intensity is plotted versus concentration of AA. The inset digital photographic images show that color disappeared, which confirms the inhibition effect of AA. A linear calibration curve was obtained for absorbance versus concentration of AA with a range of 1–17.5 mM, which exhibited excellent linear correlation, R2 = 0.992, and with low detection limit (Fig. 9d).
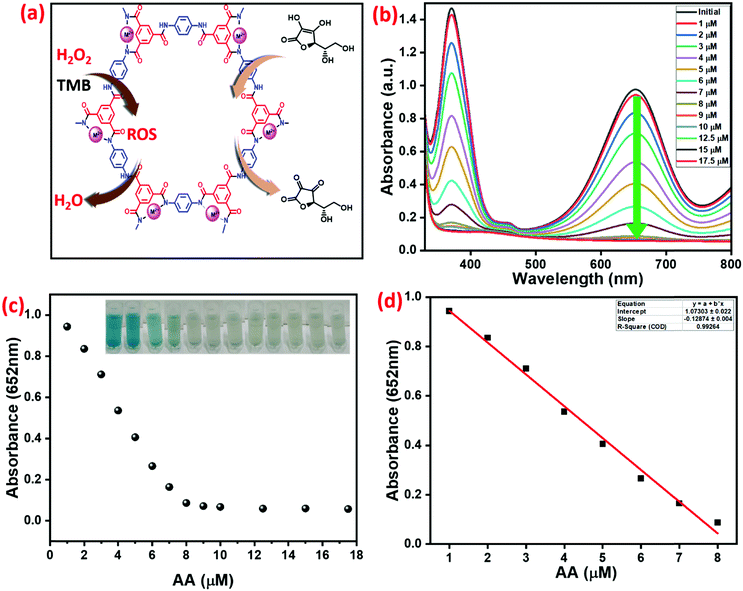 |
| Fig. 9 (a) Schematic illustration of AA to hydroascorbic acid conversion. (b) UV-visible spectra with different concentrations of AA. (c) Relative intensity plot for absorbance versus various concentrations of AA (inset shows optical images of different concentrations of AA in the reaction system). (d) Linear calibration plot for different concentrations of AA. | |
DA detection
Simultaneously, DA also inhibits the peroxidase-like activity of Cu2+@POP, as extensively investigated. DA converts to aminopurine due to the inhibition of oxidation reaction, as shown in Fig. 10a.
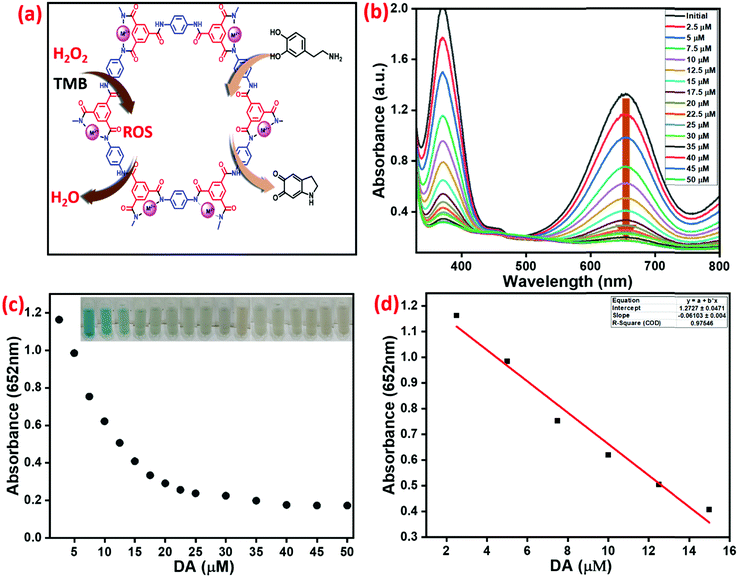 |
| Fig. 10 (a) Schematic illustration of dopamine to aminopurine conversion. (b) UV-visible spectra of different concentrations of DA in the oxidized TMB product. (c) Absorption suppression plotted as the absorbance of the band at 652 nm versus various concentrations of DA (inset optical images show different concentrations of DA in the reaction system). (d) The linear calibration plot for different concentrations of DA in the reaction system. | |
Specifically, in the absence of DA, a significant absorption peak at 652 nm corresponds to ox-TMB. Moreover, addition of DA into the system inhibits oxidation of TMB. When 2.5 μM to 50 μM of DA was introduced into the high-peroxidase-active system, apparent color was steadily eliminated to a colorless solution, as shown in Fig. 10b. Up to 20 μM, DA had an inhibition effect. On further increasing the concentration of DA, the absorption peak obtained a steady state. As shown in Fig. 10c, the relative absorption intensity was plotted versus various concentrations of DA. Inset digital photographic images display color fading, which confirms the inhibition effect and the possibility of naked-eye analysis. The linear calibration curve was plotted of absorbance versus concentration of DA with a range of 2.5–15 μM (R2 = 0.946) and detection limit was achieved, the result being depicted in Fig. 10d.
Selectivity of AA and DA detection
In order to analyze the selectivity of prepared Cu2+@POP, we conducted a series of experiments with DA, AA, Cy, Fr, G, and cystine (CysC). The results are illustrated in Fig. 11a. According to naked-eye observation, AA and DA showed selective inhibition of peroxidase-like activity with color change as shown in Fig. 11. Further, this was evidently proved from the absorption spectra, and visual color change as displayed in Fig. 11c. The absorption band at 652 nm is drastically changed in the presence of AA and DA. Other than AA and DA, there was no obvious inhibition of oxidation of TMB. From this result, we confirmed the high selectivity of Cu2+@POP to both AA and DA. We compare this with various nanozymes mentioned in the literature49–53 and listed in Table 1.
Table 1 Comparison table for related copper-based nanozymes
No. |
Materials |
Method |
Analytes |
Linear range |
LOD |
Ref. |
C, colorimetric; CL, chemiluminescence; L, laccase; DA, dopamine; AP, acid phosphatase; Ph, phenols; MB, methylene blue. |
1 |
Cu2+ |
C |
DA |
1–50 μM |
1 mM |
44
|
2 |
CuS/rGO |
C |
DA |
2–100 μM |
0.48 mM |
45
|
3 |
Cu–MOXs |
C |
DA |
0.5–20 μM |
8.6 mM |
46
|
4 |
Cu(PDA)(DMF) |
C |
DA |
1–10 mM |
— |
47
|
5 |
Cu–HMT |
C |
DA |
0.5–3.5 mM |
4.2 mM |
48
|
6 |
Cu2+–g-C3N4 |
CL |
DA |
— |
— |
16
|
7 |
Cu2+–C-dots |
CL |
DA |
— |
— |
16
|
8 |
Cu2+–rGO |
CL |
DA |
— |
— |
17
|
9 |
Cu2+–g-C3N4 |
C |
AP |
0.05 to 40 U L−1 |
8.8 mM |
18
|
10 |
Cu2+–MOF |
L |
Ph |
0.1–200 μM |
61 nM |
20
|
11 |
Cu2+–NMOFs |
CL |
DA |
— |
— |
21
|
12 |
Cu2+–COF |
C |
MB |
2.5–140 μM |
— |
22
|
13 |
Cu2+–POP |
C |
DA & AA |
2.5–15 μM and 1–17.5 mM |
1.4 mM and 1 mM |
This work |
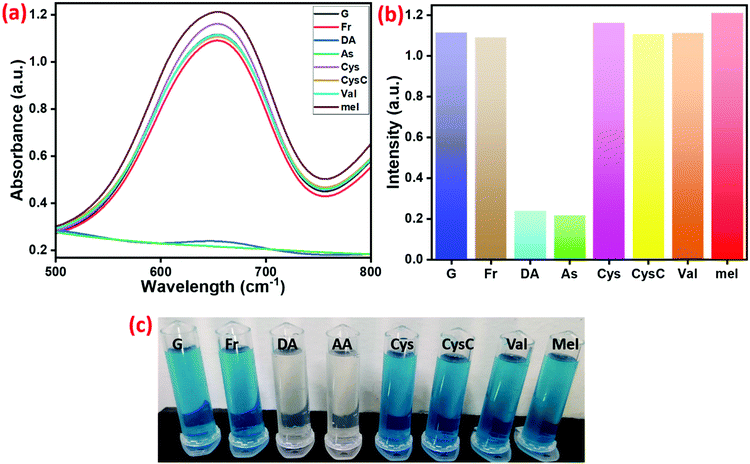 |
| Fig. 11 (a) Selectivity of Cu2+@POP towards DA detection in comparison to other interfering substances. (b) Relative detection efficiency bar diagram showing absorbance at 652 nm. (c) Optical images of decolorization in the presence of DA. The standard values are used for selectivity study: TMB (100 μM), H2O2 (100 μM), catalyst solution (0.7 mg ml−1) with DA (50 μM) or other interfering substances (100 μM). | |
Conclusion
In summary, the present work has introduced mechanochemically synthesized M2+-coordinated POPs. Interestingly, the as-obtained materials exhibited excellent peroxidase-like activity. Moreover, Cu2+ atoms were uniformly dispersed in Cu2+@POP, which is not the case for Cu0 and CuxO. Impressively, Cu2+@POP showed a high peroxidase mimic activity compared to POP, Ni2+@POP and Co2+@POP. The oxidation of TMB catalyzed by Cu2+@POP can produce blue color corresponding to a single-electron transfer process. Subsequently, the peroxidase-like activity was inhibited in the presence of AA and DA. Based on the inhibition effect, dual detections were realized with low detection limits at 1.3 mM and 1 mM for DA and AA. As-obtained Cu2+@POP has unique characteristics with enhanced peroxidase mimic activity and successfully detected AA and DA via a colorimetric method.
Conflicts of interest
There are no conflicts to declare.
Acknowledgements
The authors acknowledge the “Selective Excellence Research Initiative Fund SRMIST-2021” and Department of Chemistry, SRM Institute of Science and Technology, Tamil Nadu – 603 203, India.
References
- H. Wei and E. Wang, Chem. Soc. Rev., 2013, 42, 6060–6093 RSC.
- J. Wu, X. Wang, Q. Wang, Z. Lou, S. Li, Y. Zhu, L. Qin and H. Wei, Chem. Soc. Rev., 2019, 48, 1004–1076 RSC.
- L. Gao, J. Zhuang, L. Nie, J. Zhang, Y. Zhang, N. Gu, T. Wang, J. Feng, D. Yang, S. Perrett and X. Yan, Nat. Nanotechnol., 2007, 2, 577–583 CrossRef CAS PubMed.
- W. W. He, W. G. Wamer, Q. Xia, J. J. Yin and P. P. Fu, J. Environ. Sci. Health, Part C: Environ. Carcinog. Ecotoxicol. Rev., 2014, 32, 186–211 CrossRef CAS PubMed.
- A. AnandBabuChristus, P. Panneerselvamand and A. Ravikumar, Anal. Methods, 2018, 10, 4378–4386 RSC.
- S. Wang, D. Xu and L. Ma, Anal. Bioanal. Chem., 2018, 410, 7145–7152 CrossRef CAS PubMed.
- L. Ai, L. Li, C. Zhang, J. Fu and J. Jiang, Chem. – Eur. J., 2013, 19, 5105–15108 CrossRef PubMed.
- X. Qi, H. Tian, X. Dang, Y. Fan, Y. Zhang and H. Zhao, Anal. Methods, 2019, 11, 1111–1124 RSC.
- M. Muppidathi, P. Perumal, R. Ayyanu and S. Subramanian, Analyst, 2019, 144(9), 3111–3118 RSC.
- T. Zhang, Y. Song, Y. Xing, Y. Gu, X. Yan, H. Liu, N. Lu, H. Xu, Z. Xu, Z. Zhang and M. Yang, Nanoscale, 2019, 11, 20221–20227 RSC.
- L. Zhou, X. Luo, J. Gao, G. Liu, L. Ma, Y. He, Z. Huang and Y. Jiang, Nanoscale Adv., 2020, 2, 1036–1039 RSC.
- T. Zhang, Y. Xing, Y. Song, Y. Gu, X. Yan, N. Lu, H. Liu, Z. Xu, H. Xu, Z. Zhang and M. Yang, Anal. Chem., 2019, 91(16), 10589–10595 CrossRef CAS PubMed.
- M. Marieeswaran and P. Panneerselvam, RSC Adv., 2020, 10, 3705–3714 RSC.
- H. Xu, X. Chen, J. Gao, J. Lin, M. Addicoat, S. Irle and D. Jiang, Chem. Commun., 2014, 50, 1292–1294 RSC.
- E. Y. Choi, P. M. Barron, R. W. Novotny, H. T. Son, C. Hu and W. Choez, Inorg. Chem., 2009, 48(2), 426–428 CrossRef CAS PubMed.
- M. Vázquez-González, W. C. Liao, R. Cazelles, S. Wang, X. Yu, V. Gutkin and I. Willner, ACS Nano, 2017, 11, 3247–3253 CrossRef PubMed.
- S. Wang, R. Cazelles, W. C. Liao, M. Vázquez-González, A. Zoabi, R. Abu-Reziq and I. Willner, Nano Lett., 2017, 17, 2043–2048 CrossRef CAS PubMed.
- Y. Guo, X. Li, Y. Dong and G.-L. Wang, ACS Sustainable Chem. Eng., 2019, 7(8), 7572–7579 CrossRef CAS.
- W. Li, Y. Li, H. L. Qian, X. Zhao, C. X. Yang and X. P. Yan, Talanta, 2019, 204, 224–228 CrossRef CAS PubMed.
- C. Y. Hu, Z. W. Jiang, C. Z. Huang and Y. F. Li, Microchim. Acta, 2021, 188(8), 1–8 CrossRef PubMed.
- C. H. Chen, M. Vázquez-González, A. Kozell, A. Cecconello and I. Willner, Small, 2018, 14(5), 1703149 CrossRef PubMed.
- Y. H. Xiong, Y. M. Qin, L. J. Su and F. G. Ye, Chem. – Eur. J., 2017, 23, 11037–11045 CrossRef CAS PubMed.
- A. Trewin and A. I. Cooper, Angew. Chem., Int. Ed., 2012, 49(9), 1533–1535 CrossRef PubMed.
- P. Kaur, J. T. Hupp and S. T. Nguyen, ACS Catal., 2011, 1, 819–835 CrossRef CAS.
- Y. Zhang and S. N. Riduan, Chem. Soc. Rev., 2012, 41, 2083–2094 RSC.
- N. B. McKeown and P. M. Budd, Chem. Soc. Rev., 2006, 35, 675–683 RSC.
- T. Ben, C. Pei, D. Zhang, J. Xu, F. Deng, X. Jinga and S. Qiu, Energy Environ. Sci., 2011, 4, 3991–3999 RSC.
- A. Bhunia, V. Vasylyeva and C. Janiak, Chem. Commun., 2013, 49, 3961–3963 RSC.
- P. M. Budd, B. S. Ghanem, S. Makhseed, N. B. McKeown, K. J. Msayib and C. E. Tattershall, Chem. Commun., 2004, 230–231 RSC.
- H. A. Patel, S. H. Je, J. Park, D. P. Chen, Y. Jung, C. T. Yavuz and A. Coskun, Nat. Commun., 2013, 4, 1357–1363 CrossRef PubMed.
- P. Kaur, J. T. Hupp and S. T. Nguyen, ACS Catal., 2011, 1, 819–835 CrossRef CAS.
- D. Wu, Y. Li, Y. Zhang, P. Wang, Q. Wei and B. Du, Electrochim. Acta, 2014, 116, 244–249 CrossRef CAS.
- H. Cheng, X. Wang and H. Wei, Anal. Chem., 2015, 87, 8889–8895 CrossRef CAS PubMed.
- J. S. Aguilar, I. Paris, P. Muñoz, E. Ferrari, L. Zecca and F. A. Zucca, J. Neurochem., 2014, 129, 898–915 CrossRef PubMed.
- P. Belujon and A. A. Grace, Int. J. Neuropsychopharmacol., 2017, 20, 1036–1046 CrossRef CAS PubMed.
- X. Wei, Z. Zhang and Z. Wang, Microchem. J., 2019, 145, 55–58 CrossRef CAS.
- H. Tang, P. Lin, H. L. Chan and F. Yan, Biosens. Bioelectron., 2011, 26, 4559–4563 CrossRef CAS PubMed.
- L. Rajput and R. Banerjee, Cryst. Growth Des., 2014, 14(6), 2729–2732 CrossRef CAS.
- H. Wei, S. Chai, N. Hu, Z. Yang, L. Wei and L. Wang, Chem. Commun., 2015, 51, 12178–12181 RSC.
- L. H. Li, X. Feng, X. Cui, Y. Ma, S. Ding and W. Wang, J. Am. Chem. Soc., 2017, 139, 6042–6045 CrossRef CAS PubMed.
- G. Li, J. Ye, Q. Fang and F. Liu, Chem. Eng. J., 2019, 370, 822–830 CrossRef CAS.
- Z. Xiaojuan, S. Xifeng, M. A. Abdullah, L. Yonglan and S. Xuping, Inorg. Chem. Front., 2018, 5, 1188–1192 RSC.
- J. W. Rong, G. Z. Hou, Y. Z. Zhi, H. N. Guo and L. Dan, CCS Chem., 2020, 2, 2045–2053 Search PubMed.
- H. B. Wang, Y. Li, G.-L. Dong, T. Gan and Y.-M. Liu, New J. Chem., 2017, 41, 14364–14369 RSC.
- S. Dutta, C. Ray, S. Mallick, S. Sarkar, R. Sahoo, Y. Negishi and T. Pal, J. Phys. Chem. C, 2015, 119, 23790–23800 CrossRef CAS.
- M. X. Guo and Y. F. Li, Spectrochim. Acta, Part A, 2019, 207, 236–241 CrossRef CAS PubMed.
- F. Liu, J. He, M. Zeng, J. Hao, Q. Guo, Y. Song and L. Wang, J. Nanopart. Res., 2016, 18, 1–9 CrossRef.
- M. Marieeswaran and P. Panneerselvam, Mater. Adv., 2021, 2, 7024–7035 RSC.
- B. Liu, Y. Wang, Y. Chen, L. Guo and G. Wei, J. Mater. Chem. B, 2020, 8, 10065–10086 RSC.
- D. Zhu, B. Liu and G. Wei, Biosensors, 2021, 11(8), 259 CrossRef CAS PubMed.
- N. S. Vallabani, S. Singh and A. S. Karakoti, Appl. Surf. Sci., 2019, 492, 337–348 CrossRef CAS.
- N. S. Vallabani, A. S. Karakoti and S. Singh, Colloids Surf., B, 2017, 153, 52–60 CrossRef CAS PubMed.
- J. Shah, R. Purohit, R. Singh, A. S. Karakoti and S. Singh, J. Colloid Interface Sci., 2015, 456, 100–107 CrossRef CAS PubMed.
Footnote |
† Electronic supplementary information (ESI) available. See DOI: 10.1039/d1ma01195b |
|
This journal is © The Royal Society of Chemistry 2022 |
Click here to see how this site uses Cookies. View our privacy policy here.