DOI:
10.1039/D2NA00420H
(Review Article)
Nanoscale Adv., 2022,
4, 3462-3478
Recent advances in mechanical force-responsive drug delivery systems
Received
29th June 2022
, Accepted 15th July 2022
First published on 18th July 2022
Abstract
Mechanical force responsive drug delivery systems (in terms of mechanical force induced chemical bond breakage or physical structure destabilization) have been recently explored to exhibit a controllable pharmaceutical release behaviour at a molecular level. In comparison with chemical or biological stimulus triggers, mechanical force is not only an external but also an internal stimulus which is closely related to the physiological status of patients. However, although this mechanical force stimulus might be one of the most promising and feasible sources to achieve on-demand pharmaceutical release, current research in this field is still limited. Hence, this tutorial review aims to comprehensively evaluate the recent advances in mechanical force-responsive drug delivery systems based on different types of mechanical force, in terms of direct stimulation by compressive, tensile, and shear force, or indirect/remote stimulation by ultrasound and a magnetic field. Furthermore, the exciting developments and current challenges in this field will also be discussed to provide a blueprint for potential clinical translational research of mechanical force-responsive drug delivery systems.
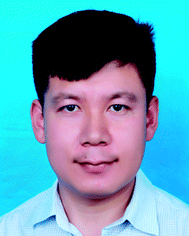 Panqin Ma | Panqin Ma received his Master's degree from Shenyang Pharmaceutical University (China) in 2015. Currently, he is a PhD candidate at the School of Pharmaceutical Sciences, Xiamen University, Xiamen (China). His main research focus is functional polymers and their applications in novel pharmaceutics delivery. |
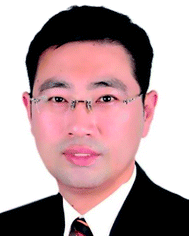 Zibiao Li | Dr. Zibiao Li is currently the director of the Sustainable Polymers division at the Institute of Sustainability for Chemicals, Energy and Environment (ISCE2), A*STAR. He has a joint appointment as a Senior Scientist at the Institute of Materials Research and Engineering (IMRE), A*STAR and an adjunct associate professor position at the National University of Singapore (NUS). Zibiao obtained his PhD in biomedical engineering from the NUS. His research interests focus on materials sustainability and MedTech polymers for healthcare applications. |
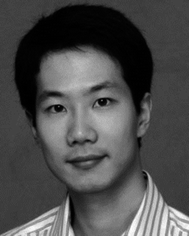 Yun-Long Wu | Yun-Long Wu is currently a professor at the School of Pharmaceutical Sciences, Xiamen University (China). He received his B. S. degree from the University of Science and Technology of China in 2005 and PhD degree from the National University of Singapore in 2011. After his postdoctoral fellow training at Nanyang Technological University (Singapore), he joined Xiamen University in 2012. His research is focused on polymeric pharmaceutical delivery system designs. |
1. Introduction
In recent years, smart drug delivery systems (DDSs) with the ability to control drug release precisely in time, location, and dosage, or with the ability to respond to physiological or external stimuli, have been extensively studied.1 As a typical example, endogenous disease-specific environments, in terms of pH,2,3 redox,4,5 hypoxia6,7 and enzymes,8,9 or external signals, in terms of light,10,11 temperature,12,13 ultrasound,14,15 and magnetic fields16,17 have been widely explored to design intelligent or safe targeted drug delivery systems.18 Among them, a DDS that responds to mechanical force stimuli, which has a wide range of sources in organisms and is of great significance in life activities, has recently attracted a lot of interest.19 For example, the compressive force of articular cartilage during movement,20 the tensile force of muscles during exercise,21 and the high shear force in the case of vascular disease22 can be utilized to trigger the release of drugs in the DDS, as shown in Fig. 1. Even with a very small mechanical force stimulus (nanonewtons) upon cytotoxic T cell receptor activation by a homologous antigen, controllable drug release can be triggered to achieve more effective and precise treatment.23 Besides the internal mechanical stimulation in organisms, mechanical force could also be generated by using external ultrasound or magnetic fields.24,25 In detail, ultrasound could generate mechanical force stimuli similar to “push”, “pull”, shear force, or radiation force through acoustic radiation.26 In the case of a magnetic field, it can be utilized to guide drug delivery and release by interacting with magnetic particles to generate physical forces.27
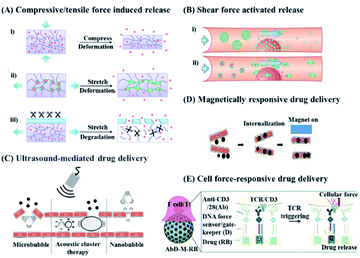 |
| Fig. 1 Schematic diagram of a drug delivery system triggered by different mechanical forces. (A and B) Drug release in response to endogenous mechanical force. Reproduced with permission.1 Copyright 2016, American Chemical Society. (A) Drug release in response to compressive or tensile force. (i) The deformation of the carrier under compression causes the loaded drug to be released. (ii) Tension-responsive particle deformation causes the release of the encapsulated drug. (iii) The material will degrade when exposed to relevant enzymes under tension strain. (B) Drug release can be triggered by (i) aggregate dissociation or (ii) shear force caused by particle deformation. (C and D) Drug release in response to exogenous mechanical force generated by ultrasound and magnetic field. Reproduced with permission.28 Copyright 2020, Elsevier BV. (C) Ultrasound produces a variety of mechanical effects, including push/pull, acoustic radiation force, and microjets, which can cause the short opening of blood vessels and control the release of drugs. (D) The magnetic field can destroy the endothelial cell–cell connection to achieve controlled drug release. (E) Drug release in response to cellular forces. Reproduced with permission.23 Copyright 2020, Royal Society of Chemistry. | |
Compared with other traditional responsive drug delivery systems (for example, a pH-responsive drug delivery system29 or enzyme-responsive drug delivery system30), mechanical force-responsive drug delivery systems have high probability for clinical translation by endogenous stimulus (i.e., compressions, tension, and shear) and external stimulus.31–36 More specifically, a DDS with ability to response to a compression stimulus could deliver insulin to reduce the glucose levels in diabetics and deliver VEGF (vascular endothelial growth factor) for induced vascular proliferation by using a calcium-crosslinked hydrogel as a carrier. Furthermore, a DDS with tension responsibility could deliver drugs and proteins via stretchable hydrogels or cross-linked micelles to treat diabetes and cancer. And microparticles or nanofibers might release drugs via shear force-activated triggers for cardiovascular use. Furthermore, external mechanical responsiveness has compelling applications, particularly ultrasonic triggers, which induce mechanochemical activation to release drugs via polymer chain extension. In this case, mechanically stimulated responsive drug delivery has great potential for translational clinical applications, including adjuvant therapy for cancer, cardiovascular disease, and diabetes.37,38
In short, a mechanical force-responsive DDS could correspondingly change mechanical properties (stiffness, viscoelasticity, geometric constraints, etc.) or chemical bonds (covalent bonds, hydrogen bonds, and ionic bonds) upon endogenous or exogenous mechanical stimulation, while these changes could serve as the source for controllable drug release.18,39 And according to the stimulation sources, mechanical force-responsive DDSs are divided into endogenous DDSs that respond to mechanical forces from the body's internal compression, tension, or shear forces,32,33,40–42 and exogenous DDSs that respond to external magnetism or ultrasound. Thanks to widespread and easily accessible mechanical stimuli, a mechanical force-responsive DDS is expected to achieve high precision and deep penetration level drug delivery at the desired location and time.28 Actually, compared with chemical or biological response systems, mechanical force-responsive DDSs can more easily predict the direction of drug delivery and manage the drug concentration range.39 Thanks to these advantages, mechanical force responsive DDSs have been utilized in the treatments of cardiovascular disease,43 wound healing,44 bone regeneration,20 or diabetes.45 For example, ultrasound could serve as an efficient and non-invasive trigger to generate exogenous forces through effects such as cavitation and radiation, so as to achieve positioning and controllable on-demand drug delivery or release.46 On the other hand, the magnetic force induced by a magnetic field can guide the magnetically responsive particles to be transported to deep tissues, and achieve the on-demand release of drugs upon dissociation or morphological changes.47 Furthermore, ultrasound and magnetism can also control the release of drugs through thermal effects, and are used as triggers for cancer treatment.48–51 In this case, recent development in the field of a mechanical force responsive DDS is worth summarizing, in terms of endogenous mechanical or exogenous mechanical stimuli (Scheme 1), which might be helpful for the future design of DDSs towards more safe and precise disease treatments.
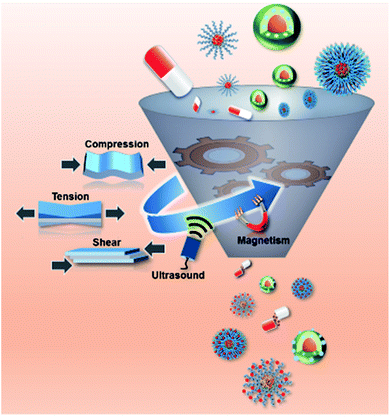 |
| Scheme 1 Schematic illustration of a mechanical force responsive DDS, upon the stimulation of endogenous mechanical force (compression, tension, and shear force) or exogenous mechanical force (ultrasound and magnetism). | |
2 Endogenous mechanical force responsive DDS
The human body can generate endogenous mechanical forces including compression, tension, and shearing force as a stimulus to control the release of drugs in a DDS. In detail, tension can be produced by muscle stretching (i.e., heart muscle, smooth muscle, and skeletal muscle), skin, tendons, and bone joints, while compression force can be observed in the stomach and bones. Shear forces are mostly generated in the cardiovascular system in vivo.39 At the cellular level, these mechanical forces regulate growth, differentiation, shape changes, and death by affecting the opening of ion channels, morphology of matrix proteins, unfolding of cytoplasmic proteins, changes in enzyme dynamics, and formation of chemical bonds.19,52,53 In short, mechanical force exists extensively in the human body and has a significant effect on physiological behaviors. Therefore, mechanical force might be worthy to be utilized as stimuli resource to control the performance of DDS. More importantly, when these mechanical forces change due to pathological factors, this trigger could be designed to achieve on-demand drug release for feedback therapy. In the following section, a representative DDS that responds to these endogenous mechanical forces is discussed in detail.
2.1 Compression force responsive DDS
Currently, the compression force responsive DDS developed is based on hydrogels or microcapsules, which can be deformed by stretching,20,44,54,55 with high biocompatibility or with high similarity to the extracellular matrix, and with mechanical responsiveness.56 As a typical example, Fang et al. designed a tough and zwitterionic hydrogel, made of Pluronic F127 diacrylate micelles and N,N′-methylenebis (acrylamide) as crosslinking agents, with mechanical responsibility.44 Upon the application of external pressure, the polymer chain hydrogel will deform, resulting in a decrease in the strength of the hydrophobic interaction within the hydrogel core as well as the release of encapsulated drugs. Furthermore, due to the reversible characteristics of electrostatic interaction and hydrophobic association, this hydrogel could quickly restore its original shape without pressure stimulation. In order to control drug release in a precise manner, the drug release of the hydrogel under various mechanical strain stimuli and the penetration of the drug into the skin tissue were characterized. The experimental results showed that the drug release rate was 9.1%, under a strain pressure close to that of human finger joints as well as 250 stimulations at a rate of 6 s per cycle. More importantly, upon the application of compression force stimulation, the released drug achieved deeper penetration into the tissue. Therefore, this hydrogel might be useful in dynamic mechanical environments such as acute wounds, which are frequently deformed by various mechanical forces, to promote wound healing.
As another example, microcapsules, which are self-assembly core–shell composites,57,58 are also sensitive to external pressure.59 Microcapsules can be designed to show a response to enzymes, heat, pH, or even compression forces for controllable drug release.50 For example, in a recent study Mohanraj et al. have developed a mechanically activatable microcapsule (MAMC) (Fig. 2A),20 whose thickness-to-diameter ratio (t/D) within the shell can be adjusted by controlling the concentration of poly (D,L-lactide-co-glycolide) acid (PLGA) in the MAMC and the flow rate of the fluid phase in the preparation device. The t/D of the microcapsule significantly affected the response rate of the microcapsule to pressure stimulation. In detail, at a higher t/D, the MAMC will only break under a higher compression force. However, under physiological conditions, an MAMC with a higher concentration of PLGA can maintain the structural integrity of the shell for up to 70 d, and can still maintain the characteristics of pressure activation (as shown in Fig. 2B). More interestingly, programmable drug release can be established by combining microcapsules with different pressure thresholds (as shown in Fig. 2C), which might be useful for activatable drug release in the synovial space or embedding in the repair site for on-demand tissue regeneration.20 In short, this work introduced an MAMC as a programmable drug delivery system and explored the relationship between the mechanical properties of the MAMC and drug release, which could be of great significance for the subsequent design of programmable mechanical response drug delivery systems. In another study, Hansen et al. designed polyelectrolyte multilayer (PEM) microcapsules, which were made of dextran, poly-l-lysine (PLL), poly-l-glutamic acid (PLG), and fibrinogen (fbn) and utilized to encapsulate drugs of pro-clotting biotherapeutic factor VIII (fVIII).60 These PEM microcapsules could bind and hybridize with platelets, while the platelets targeted the vascular injury site. Upon platelet adhesion and activation, the platelets might immediately produce contraction force, which created a hole on the PEM capsule and accelerated the encapsulated drug release. In comparison with systemic administration of the same drug formulation, the use of drug-loaded PEM microcapsules could achieve faster (18 min faster) clot formation, because of the fast response of this DDS immediately after platelet activation and release of local but high-dose drugs to enhance hemostasis. Hence, there is no doubt that this mechanically responsive DDS is a more aggressive method of targeted drug delivery than other targeted systems relying on diffusion release, chemical or enzymatic dissolution, or using external equipment in the case of hemostasis.
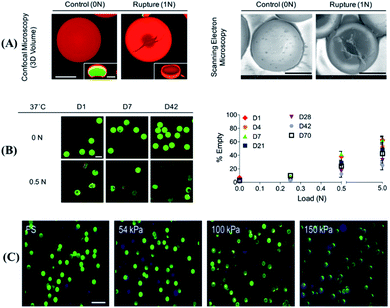 |
| Fig. 2 Mechanical activity and stability of a mechanically activatable microcapsule (MAMC). (A) The volume (confocal, scale bar = 50 μm) and shape (SEM, scale bar = 50 μm) of fast-degrading MAMCs containing dextran (green) with labeled shells (red) and illustration of the rupture mechanism. (B) The stability and the mechanical activation response at low pressure of slow degrading MAMCs from day 1 (D1) to incubation for more than 70 days (D70). Fluorescent images scale bar = 100 μm. (C) Typical confocal images of MAMCs in different stiffness matrix hydrogels (matrix: blue, MAMCs: green, ruptured MAMCs with loss of internal signal). Scale bar = 200 μm. Reproduced with permission.20 Copyright 2019, John Wiley & Sons. | |
In short, current reports have shown that it is promising to achieve on-demand controlled drug release through endogenous compression forces. However, most of the current research is conceptual verification, and further verification on animal models might be necessary and a number of issues including the preparation process or safety concerns might also need to be resolved before clinical application.
2.2 Tension responsive DDS
Since a large amount of strain and stretch is usually required in daily exercise, tension-responsive DDSs are considered to be a design with an easy stimulus source.61 Currently, most of the tension response systems are based on elastic materials or composite materials that can produce cracks.57,62,63 These DDSs could generally crack under the pulling force from tissues such as bones or muscles, and the enclosed drug could be released.64–66 Although these systems can be smartly designed, it is still difficult to precisely control the amount of drug released, which might affect the therapeutic effect. In order to solve this issue, Jeon et al. reported a more precise dose control DDS based on a film composed of a polyurethane polymer layer coated with a biocompatible titanium metal layer, which generated microcracks under tensile strain.67 The manufacturing process of this drug delivery system is simple, with possible mass production potentials. More importantly, there is a good linear correlation between the amount of drug released and the physically exposed crack area of the metal layer generated under different tensions (as shown in Fig. 3), which showed an accurate control of drug release by this DDS. By controlling the amount of tension, the crack area exposed by the film can be controlled, and the amount of drug released could be tuned. However, this system had the problem of drug leakage when there is no stretching force stimulation, and no corresponding disease treatment model has been established.
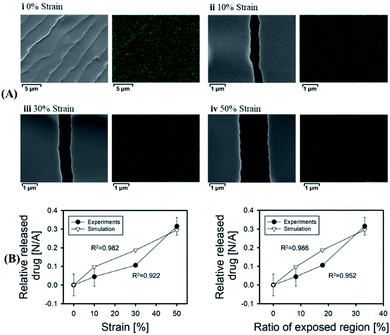 |
| Fig. 3 Correlation between the amount of drug released and exposed region of the stretched film. (A)Scanning electron microscope (SEM) images and energy dispersive X-ray spectroscopy images of the stretched membranes at various tensile strains. (B) Profiles of the relative released drug versus strain and the ‘ratio of the exposed region’ under experimental and theoretical conditions. Reproduced with permission.67 Copyright 2018, Elsevier BV. | |
In another study, Wei et al. designed a three-layer composite DDS that can respond to tension and strain.65 In this system, the outermost layer is a cera flava coating used to isolate external solutions, and the middle layer is an elastic polycaprolactone (PCL) electrospinning tub, while the innermost layer is an electrospinning film of PCL that wraps around the drug. Upon stimulation by tensile force, the outermost layer might produce a micro-mechanical door, which increased the wettability of the electrospinning tube of the middle layer. In this case, the drug could diffuse outward along the concentration gradient. In case the external stretching disappeared, the micro-mechanical door could be closed to stop drug release. Furthermore, in vitro experiments and animal models were utilized to verify the effectiveness of this composite structure. However, the tensile response range of this composite structure and the drug release amount is small. In order to solve this issue, a material with a superhydrophobic structure was promising for tension-responsive drug delivery.66,68 Generally speaking, such a system could maintain the stability of trapped air for several weeks to several months, and might effectively prevent drug release without specific mechanical stimulation.69 For example, Wang et al. recently designed a hydrophilic material containing the drug as the core, and used superhydrophobic biomaterials as coatings to prepare a multi-layer tension-responsive DDS.70 In detail, this super-hydrophobic coating could shield the core from the surrounding environment, and might form cracks on the super-hydrophobic coating when subjected to tensile strain. The crack formation could promote system wetting, and lead to drug release. From experimental results, the release rate of the small molecule drug cisplatin in the hydrophilic core was positively correlated to the thicknesses of superhydrophobic coatings, upon 10% increase of the mesh's original length. Furthermore, because this superhydrophobic coating might minimize the exposure of the contents, this DDS could even be utilized to achieve controllable release of macromolecular active therapeutic proteins, within 50% strain under tension.
In short, current reports have shown that it is feasible to control the release of drugs through endogenous tension, although it is still under exploration that the size and duration of tension stimulation should be controlled by reasonable designs to control the drug dosage and to meet the clinical medication needs.
2.3 Shear force responsive DDS
Shear force responsive drug delivery generally uses high shear of blood vessels under pathological conditions as stimulating conditions.71 It is worth mentioning that the magnitude of the shear force is very different in a healthy arterial system and a pathologically narrowed blood vessel. In detail, the shear stress in a healthy arterial system is around 15 dynes cm−2 and is quite stable, while the narrowed blood vessels might exhibit a shear stress ranging from 150 to 1000 dyne cm−2.72 In the case of the lipid bilayer of liposomes with a high degree of flexibility, the flowing fluid with low shear stress might come into contact with the lipid bilayer of the liposome, and the liposome might still be able to maintain its structure integrity. However, the structure of the liposome might not remain intact if it comes in contact with a fluid environment with high shear stress, and its payload could be released, which is the prototype of first generation shear-responsive liposomes.73–75 However, liposomal drugs are generally considered foreign particles. In this case, they might be attacked by the immune system, which caused a complement activation-related pseudo-allergy (CARPA).76 In order to explore this issue, Buscema et al. prepared 1,3-palmitoylamido-1,3-deoxy-sn-glycero-2-phosphatidylcholine (Pad-PC-Pad) liposomes with shear responsiveness and focused on this liposome CARPA risk.77 They found that the Pad-PC-Pad liposomes might cause weak complement activation, but Pad-PC-Pad exhibited fewer or similar CARPA risks in comparison with Doxil (an Food and Drug Administration or FDA-approved liposomal drug). In other words, Pad-PC-Pad liposomes might have sufficient safety and are a promising targeted mechanical response drug delivery system. Furthermore, inspired by dinoflagellates that emit light due to the movement of the surrounding water, Rifaie-Graham et al. designed a polymer vesicle, which was composed of two types of amphiphilic block copolymers with adenine or thymine embedded in the hydrophobic region.22 This vesicle contained nucleobases. Upon nucleobase pairing, the water-soluble compounds contained in the vesicle are impermeable.
On the other hand, when the nucleobases are cleaved by mechanical stimulation, the drugs within the vesicles might be released osmotically (as shown in Fig. 4A). In order to verify this concept, fluorescein sodium was packed in the vesicles and the vesicles were placed in microfluidic channels of different widths to simulate different intensities of shearing (as shown in Fig. 4C). More interestingly, fluorescence can be detected under high shear stress. With the increase in intensity, 45% of the drug was released at a flow rate of 70 mL min−1 and a detection system width of 0.65 mm (as shown in Fig. 4B). Furthermore, the shear force threshold range for drug penetration was at a flow rate of around 15 mL min−1 through a detector with a diameter of 0.65 mm. In this case, vesicles with a radius of 100–300 nm were subjected to a shear force, which exceeded the shear force in normal tissues but was within the shear force in the pathological range. However, the number of nucleobases in such vesicles can not only affect the threshold of the shear force response, but also the self-assembly of vesicles. Therefore, further study could be conducted on more nucleobases which were suitable for shear-responsive DDS designs.
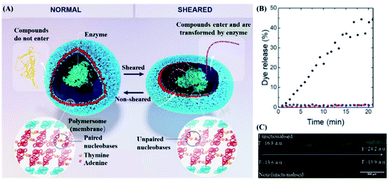 |
| Fig. 4 Structure and drug release of shear-reactive polymer vesicles. (A) Schematic diagram of the inner cavity of polymer vesicles with or without shear stress. (B) Fluorescence microscope images of polymer vesicles filled with sodium fluorescein with or without shear stress obtained using a microfluidic device with multiple contractions from left to right. (C) Fluorescein sodium is released from the shear-responsive polymer vesicles with a shear flow (black) or in the absence of a shear flow (red), and non-shear-responsive polymer vesicles with a shear flow (blue). Reproduced with permission.22 Copyright 2021, John Wiley & Sons. | |
As another example, platelets will be blocked at the injury site upon occurrence of vascular injury, indicating that local high shear stress is an important factor in platelet adhesion and activation.79,80 In this case, platelets might be pathologically activated, leading to uncontrolled growth of thrombus, thereby blocking blood vessels or embolism, and leading to downstream ischemic events. However, it might also create a possibility for novel shear-sensitive drug delivery platforms.43 For example, Yang et al. coated poly (DL-lactide-glycolic acid copolymer) (PLGA) nanoparticles (PNPs) with a complete platelet membrane to simulate platelet adhesion under high shear stress.81 In the in vitro experiments, under different shear stresses, PNPs showed stronger binding to platelet adhesion-related proteins than the NP group only. Furthermore, PNPs had the ability to bind to multiple sites of the sclerosing aortic valve, which might be enriched in the sclerosing aortic valve under high shear stress and increase the local drug concentration. Therefore, PNPs are promising as a platform for the treatment of cardiovascular diseases such as aortic stenosis. However, this platform required natural platelet membranes, which might be difficult to prepare and had large differences between batches. In this case, more reliable production technology is required for clinical transformation. Similarly, inspired by platelet shear activation, shear-activated mechanically sensitive nanoparticle aggregates have also been developed.72 For example, Zukerman et al. designed functionalized carboxylated polystyrene nanoparticles (VTC) with one or a combination of the E-selectin binding peptide (Esbp) and anti-ICAM-1 antibody with different densities to target endothelial cells (as shown in Fig. 5).78 Esbp and the anti-ICAM-1 antibody are two common inflammation ligands that can adhere to vascular endothelial cells during inflammation. It was found that nanoparticles functionalized with Esbp or the anti-ICAM-1 antibody might enhance adhesion to vascular endothelial cells. More importantly, the adhesion ability of functionalized nanoparticles might increase with shear force, indicating that the targeting function of nanoparticles is related to the density of functionalized substances and the strength of shearing force.
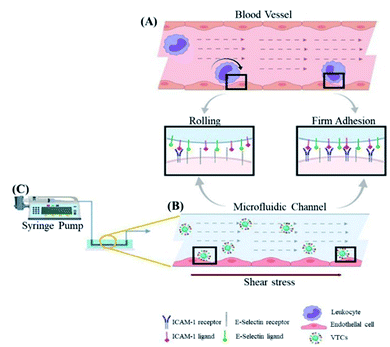 |
| Fig. 5 Schematic illustration of the interaction between functionalized polystryrene nanoparticles (as a vector targeting endothelial cells or VCT) and endothelial cells under high shear stress. Schematic diagram of (A) leukocytes and (B) VTC that mimics white blood cells activating endothelial cells under the blood flow mediated by overexpression of ICAM 1 and E-selectin. (C) In microfluidics, the channel controls the flow of injection pump with ability to apply shear stress on endothelial cells. Reproduced with permission.78 Copyright 2020, Wiley VCH. | |
In short, a shear force-responsive DDS could achieve targeted and controllable drug release in a non-invasive manner. However, shear-sensitive drug carriers remain largely unexplored.72 Many challenges such as no universally recognized measurement method to characterize the shear activation performance of drug carriers and the relatively short in vivo circulation time of drug carriers still need to be solved.
3. Exogenous ultrasound responsive DDS
Ultrasound (US) not only has a direct therapeutic effect on diseases,82 but also the ability to serve as a physical trigger. On the other hand, ultrasonic waves could produce thermal effects and mechanical effects such as cavitation, which might also help enhance drug delivery and release.28 In detail, recent advances in ultrasound-responsive DDSs have been summarized in Table 1. Herein, drug delivery in response to mechanical effects was focused on “push” or “pull”, radiation force, and shear-related acoustic current induced by ultrasound.
Table 1 Typical examples of the delivery of different types of drugs mediated by ultrasonic mechanical effects
Validation model; types of drug delivery systems |
Mechanical effects of ultrasound; US parameters |
Effect |
In vitro release; poly (methyl acrylate) (PMA) contains a furan-maleimide mechanical group as the chain center14 |
Elongational forces; frequency: 20 kHz; intensity: 8.2 W cm−2 |
US stimulation can successfully release chemically bound small molecules. The highest release is 87%, which can effectively predict the release amount |
Microfluidic platform; microbubbles83 |
Acoustic radiation force; frequency: 107 kHz, acoustic pressure: 41.7 kPa |
Enhance the permeability of cell membranes; reduce cell mortality |
In vitro and in vivo experiments in mice; fluorous nanoemulsions72,84 |
Vaporization; acoustic pressure; intensity: 0.1–2.0 W cm−2 |
Encapsulate the protein into the fluorine emulsion core and transport it into the cell |
In vitro cell experiment; polyelectrolyte multilayer microcapsules85 |
Acoustic pressure; frequency: 2.25 MHz, Intensity: 115 mW cm−2 |
Release the drug only under ultrasound induction |
In vitro and in vivo experiments in mice; polymeric micelles74,86 |
Cavitation; acoustic radiation force; frequency: 1.90 MHz, intensity: 0.4 W cm−2 |
Significantly inhibited tumor growth |
In vitro release; microcapsules based on multiple emulsions87 |
Interface shear force; frequency: 1 MHz, intensity: 1.9 W cm−2 |
Realize the coordinated delivery of hydrophilic and hydrophobic payloads |
Human bladder organoid model; microbubbles88 |
Cavitation; acoustic radiation force; frequency: 1.1 MHz acoustic pressure: 2.5 MPa |
Drug delivery increased 16-fold compared to the control; no cell damage detected |
3.1 Microbubble induced drug release
Not only serving as a useful drug carrier that can enhance the permeability of drugs in cells and tissues, microbubbles have high compressibility, which allows them to scatter ultrasound with unique echoes which can be monitored by diagnostic imaging.89 On the other hand, ultrasound can not only release drugs from microbubbles through effects such as cavitation, but also increase the permeability of the vascular system or cell membranes through acoustic pore effects.90 Based on these characteristics, a number of studies have reported smart microbubble drug delivery systems based on the ultrasound response.91–96 In addition, microbubbles can lower the cavitation threshold by increasing the local permeability, thus controlling the release of the drug.38 As a typical example, Horsley et al. prepared sulfur hexafluoride-filled bubbles (a popular clinical contrast agent) consisting of biocompatible lipid shells for gentamicin encapsulation.88 By using a human bladder organoid model, this microbubble was validated to have comparable cytotoxicity with that of conventional antibacterial therapy. More importantly, the microbubbles activated by ultrasound could increase the intracellular drug concentration which was two times higher than that in the case of unstimulated microbubbles, and more than 16 times in comparison with the free drug. In this case, the gentamicin concentration for treatment was much lower than the minimum inhibitory concentration in case of direct administration, with a satisfactory inhibition effect to prevent the recurrence of urinary tract infections. In another work, Chen et al. designed a polymer microcapsule composed of tannic acid and poly (N-vinylpyrrolidone) (TA/PVPON).85 In detail, TA/PVPON capsules had good echo characteristics, and could be imaged and positioned under a low-intensity ultrasound of 0.1 W cm−1. Under high-intensity ultrasound stimulation that exceeded the threshold, the drug might be released from the capsule. More interestingly, without ultrasound stimulation, the drug release could also be stopped (Fig. 6A). Furthermore, the thickness of the capsule shell, the intensity of the ultrasound, the duration of the ultrasound, and the pH of the solution could serve as factors to control the drug release behavior (Fig. 6B and D). And in vitro studies showed that the TA/PVPON capsules could effectively release drugs upon ultrasonic stimulation to achieve on-demand administration (Fig. 6C). It is worth mentioning that the ratio of the capsule shell thickness to the size might affect ultrasound sensitivity, while the composition of the capsule might also affect drug release and safety issues. Furthermore, Batchelor et al. also developed nested nanobubbles (nested-NBs) as an ultrasound-responsive drug delivery system.97 Interestingly, when high-intensity focused ultrasound (HIFU) in pulse mode was used to trigger the drug release of nested-NBs, it was found that the structure of the liposomes was still intact, although the liposome-encapsulated NBs were destroyed. In this case, the drug could not be released. When HIFU in continuous wave (CW) mode was used as the trigger, NBs could undergo a phase change from liquid to gas. In this case, the particle size became large enough to break the liposomes, which was able to release the encapsulated drugs, indicating the promising controllable drug delivery application of microbubbles. More importantly, microbubbles could also enable synergistic chemotherapy and photodynamic therapy (PDT) by co-loading chemotherapeutics and photosensitizers.
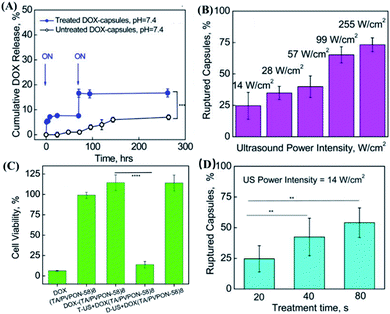 |
| Fig. 6
In vitro drug release experiment of US-responsive DOX–(TA/PVPON-58)8 capsules. (A) On-off controllable drug release of ultrasound-responsive capsules of DOX–(TA/PVPON-58)8 capsules. Arrows indicate the point at which the US was applied (ON). (B) The dependence of capsule rupture (%) on the US power intensity (W cm−2) under the condition of 20 seconds of US duration time, pH = 7.4. (C) In vitro anticancer activity of ultrasound-responsive DOX–(TA/PVPON-58)8 capsules. (D) The dependence of capsule rupture (%) on the time of ultrasonic treatment under the condition of a US intensity of 14 W cm−1, pH = 7.4. Reproduced with permission.85 Copyright 2017, American Chemical Society. | |
3.2 Nanoemulsion based on perfluorocarbons
Perfluorocarbon (PFC) nanoemulsion is a type of carrier, which has been extensively studied in the delivery of drugs in response to ultrasound stimulation.98,99 Due to the weak intermolecular forces caused by the low polarizability of fluorine and strong intramolecular C–F bonds, PFC could respond to ultrasound for a liquid–gas phase transition.100 There are a number of studies on the mechanism of the liquid–gas phase transition of PFC caused by ultrasound.98 For example, the shear force101 and high excitation pressure102 generated by ultrasound might cause the liquid–gas phase transition of PFC. Thanks to this unique feature, ultrasound-responsive drug delivery systems based on PFC nanoemulsions could be realized. As a typical example, Cao et al. constructed a programmable chemotherapeutic drug delivery nanoemulsion with phase change ability by using a low-intensity focused ultrasound (3 W and 8 W ultrasonic power) trigger.103 The in vitro release experiment showed that, when the ultrasonic power reaches the threshold, sudden drug release could be achieved. The in vivo experimental results showed that low-intensity focused ultrasound radiation might improve the efficiency of passive targeting of nano-droplets to tumor tissues. It is also important that the bubble oscillation produced by the phase transition of this nanoemulsion and micro-shearing might cause microvascular system destruction, which further improved tumor growth inhibition efficacy. However, this system had drug leakage after only 4 h, indicating that its stability needs might need to be further improved.
In another study, Zhong et al. developed a US-responsive polymeric perfluoropentane nanoemulsion for clinical transformation (Fig. 7A).104 Interestingly, this nanoemulsion could maintain good stability after as many as five freeze–thaw cycles, and the monodispersity difference of each batch was quite small (Fig. 7B and C). More importantly, by encapsulating drugs with different molecular weights and hydrophobicity, it was found that this nanoemulsion could widely encapsulate small-molecule hydrophobic drugs (Fig. 7D). The mechanism study showed that non-mechanical effects such as heating generated by ultrasound were unlikely to have a significant impact on drug release, while the release behaviour was closely related to the ultrasonic effect. Furthermore, this nanoemulsion had not been found to cause obvious toxicity in up to 100 mice experiments. The in vivo experiment had also shown that the release of drugs from the perfluorocarbon nanoemulsion under the stimulation of ultrasound could increase the local drug concentration and produce substantial drug effects. However, this nanoemulsion might only deliver small-molecule hydrophobic drugs, which limited its application. In a study by Sloand et al., another emulsion formulation, made of fluoro-amphiphilic chemical tags (FTags) with fluorine masking ability, was capable of delivering macromolecular proteins.84 In detail, this design could adjust the number of fluorine molecules in perfluorocarbon and the polarity, while the macromolecular protein can be dispersed in the perfluorocarbon emulsion. At the same time, due to the fluorine–fluorine interaction, the biological activity of protein molecules could be maintained. In order to prove this concept, FTags were combined with green fluorescent protein (GFP) and were successfully dispersed in a perfluorocarbon solvent, where a peptide emulsifier was further used to improve cell targeting ability as the emulsion shell for successful preparation of a protein-loaded fluorine nanoemulsion. More interestingly, an ultrasonic power of 0.1 W cm−1 could cause the fluorine nanoemulsion to form bubbles or cavitation, which in turn caused the cell membrane to form transient pores. In this case, GFP could more easily enter the cell and exhibit a 150 time intracellular concentration increase in comparison with the control group without ultrasound. In vivo experiments had also proved that this system could deliver 7 times more antibodies to tumour cells compared to the control group without ultrasound. Zhao et al. developed an enzyme activity regulation system triggered by ultrasound, describing the release and activation of enzymes by gold nanoparticles (AuNPs) through ultrasound disassembly.35 In addition, Zhou et al. designed a switch to control protein activity by using the mechanical force of ultrasonic waves to stretch and break polymer chains, and in turn remotely controlling the bioactive molecule.36 In short, an emulsion is a very attractive DDS for large molecules such as proteins, but the stability and safety of this system might also need further exploration.
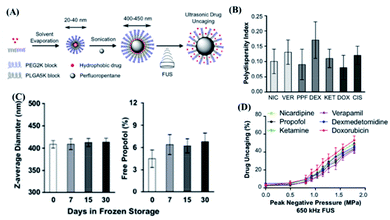 |
| Fig. 7 Polymer perfluoropentane nanoemulsion as an ultrasound-responsive delivery system for encapsulating hydrophobic drugs. (A) Schematic of polymer perfluoropentane nanoemulsion production and ultrasonic drug uncaging. (B) Polydispersity index of perfluoropentane nanoemulsion loaded with propofol (PPF), nicardipine (NIC), verapamil (VER), dexmedetomidine (DEX), ketamine (KET), doxorubicin (DOX) or cisplatin. (C) Stability of perfluoropentane nanoemulsion under frozen storage and thawing. (D) In vitro drug release under ultrasound stimulation of perfluoropentane nanoemulsion loaded with different hydrophobic drugs (650 kHz ultrasound treatment, 50 ms pulse repetition 60 times). Reproduced with permission.104 Copyright 2019, Elsevier Ltd. | |
3.3 Other ultrasound responsive DDSs
Recently, liposome and hydrogel delivery systems that respond to ultrasound have also been reported.46,105,106 Furthermore, a number of vesicles with ultrasound response have also been encapsulated in liposomes.107 For example, Awad et al. synthesized a PEGylated liposome and modified the liposome with different targeting proteins.108 This liposome could be stimulated by low-frequency ultrasound (20 kHz) with a power density of 12 W cm−1.2 It was found that 57.5% of the drug was released, while only 22% of the drug was released from the non-PEGylated liposome, indicating the importance of the amount of polyethylene glycol (PEG) in this design. On the other hand, hydrogels could also respond to power from external mechanical stimuli, indicating its broad prospects as smart DDSs.15 For example, Huang et al. designed a hydrogel in which dopamine functionalized PEG was bonded to Fe3+.109 By using intramolecular hydrogen bonds or Fe3+ coordination bonds with catechol, this hydrogel had an energy dissipation mechanism.110 In this case, this hydrogel had mechanical stimulation ability, and could restore its original state after stimulation. The in vitro experimental results showed that, at an ultrasonic intensity of 43 W cm−2, the hydrogel showed close to 50% disintegration. More interestingly, upon the disappearance of ultrasonic stimulation, the generation of coordination bonds could be re-observed. More importantly, by changing the amount or ratio of catechol and Fe3+, the self-healing ability of the hydrogel and the sensitivity to the ultrasound response might be tuned. Therefore, it is expected to further improve the performance of this water coagulation so that it might be applied to embed an ultrasonic responsive DDS.
Recent endeavors have also shown that prodrug designs could also be activated by ultrasound. As a typical case, Huo et al. constructed a representative prodrug molecular framework that can be activated by ultrasound in three ways.33 First, the drug molecule was attached to the carbonate linker at the β-position of the disulfide bond within the polymer. Upon stimulation by ultrasound, the disulfide bond breaks and a series of chemical reactions occur to release the drug from the carbonate linker. The second method is to rely on the strong combination between the RNA (ribonucleic acid) aptamer and drug molecule, for inactive prodrug design. Upon ultrasound stimulation, the non-covalent interaction (such as a hydrogen bond or electrostatic interaction) was destroyed. When the additional depolymerization of the phosphodiester RNA backbone and the breaking of the covalent bond occurred, the drug could be re-activated. The third method is based on the supramolecular binding between the drug molecule and its H-bond complementary peptide target sequence. Under ultrasound activation, the hydrogen bond in multivalent hydrogen bond mechanical group broke, thereby activating the drug, indicating the feasibility of prodrug designs triggered by the mechanochemical effect of ultrasound.
In short, as a non-invasive method, ultrasound could effectively control drug release at a pre-determined location, time, and dose by changing the exposure time and frequency. However, current ultrasound-responsive DDSs are still mainly limited to cell culture experiments and a small number of in vivo studies in mice models. Before clinical transformation, large animal models and further research on optimizing ultrasound parameters or the treatment process might be required.
4. Exogenous magnetic force responsive DDS
Magnetically responsive nanocarriers have a satisfactory side effect111 and highly controllable behavior.112 Usually, by grafting biocompatible polymers with target groups onto the surface of magnetically responsive nanoparticles, the composite system could not only show low toxicity, but also had the function of magnetic targeting. Currently, a variety of DDSs such as liposomes,113 vesicles,114 hydrogel beads115 and dendritic polymers116 with magnetic response designs have been widely reported. Most magnetically responsive DDSs used magnetically guided targeting or magnetically generated heat for therapy or controllable drug release.117 Herein, a DDS with magnetic force was chosen as the main factor to control its drug release behavior, although magnetic hyperthermia could also be a useful approach.118 In the following section, recent reports on magnetic response DDSs from the perspective of the external magnetic field and the magnet guidance system of the magnetic implant will be discussed.
4.1 External magnetic field responsive DDS
Generally, magnetic drug targeting can be guided by using external electromagnetic coils or various types of permanent magnets.112 A neodymium iron boron (NdFeB) magnet is one of the most used magnetic navigation systems for magnetic targeted DDSs, because it could provide a strong magnetic field per unit volume.119–121 For example, Qiu et al. designed a magnetic nano DDS with significant uptake increase within endothelial cells, through the magnetic force produced by the NdFeB magnet, which is similar to the intracellular magnetic force.27 First, in microfluidics, the induction of magnetic force was verified to reversibly change the distribution of VE-cadherin in endothelial cells and the cytoskeleton of actin, thereby destroying the adhesion between cells and making the cells more permeable. The cellular experimental results showed that the nanoparticle uptake amount by magnetically guided cells was 2.3 times more, in comparison with that in non-magnetically induced cells. Furthermore, in vivo mice experiments also proved that the location of particles could be controlled by magnetic guidance, with enhanced permeability of blood vessels.
Besides the ability to effectively control drug release, magnetic force could also guide the designated location of a DDS. For example, Li et al. prepared platelets coated with L-arginine and γ-Fe2O3 magnetic nanoparticles (PAMNs), with the aim to precisely deliver PAMNs to the lesion site through both active platelet transport and magnetic-guided targeting (as shown in Fig. 8A).122 This hypothesis has been verified in a mice model of cerebral cortex ischemic stroke. By placing a magnet that generates a static magnetic field above the lesion, the PAMN amount at the lesion could be twice as high as that without the guidance of a magnetic field (as shown in Fig. 8B). The analysis of immunohistochemical slices proved that PAMNs could better reach the brain injury site under the action of the magnetic field, thereby significantly reducing the symptoms (as shown in Fig. 8C). More importantly, when guided by a magnetic field, PAMNs could effectively reproduce the dynamic blood flow of the diseased part and increase the revascularization rate and blood flow. However, it is difficult to locate areas below 5 cm under the skin by this kind of static magnetic field controlled drug release and targeting.123 Therefore, the dynamic control of the magnet is required to deliver the drug to a deeper site. For example, Shamsi et al. used a theoretical model to explore the influence of the magnetic strength, tumor–magnet distance, and magnetic particle size on the dynamics of drugs reaching peritoneal tumors.124 By using a simulation model, a strong enough magnetic force (2.5 T) can transport nanoparticles to medium (R = 5 mm) and large (R = 10 mm) tumors, via the capillary approach.125 Although it might be difficult to deliver drugs to deep tissues by magnetic targeting alone, it could be combined with other methods to deliver drugs to any part of the body in multiple targeted directions. For example, Qiao et al. developed a dual-targeted DDS guided by the ability to bind bacteria and magnetic field responsibility.126 By taking advantage of precise bacterial capture ability and magnetic targeting, drugs can be effectively transported to deep bone marrow tissues, indicating the potential to completely eradicate methicillin resistant Staphylococcus aureus infected osteomyelitis.
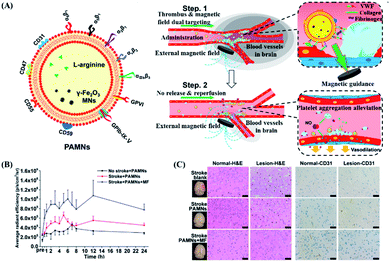 |
| Fig. 8 Magnetic response PAMN for in vivo verification of early ischemic stroke. (A) Schematic diagram of the structure of PAMN and the effect of in vivo magnetic targeted drug release. (B) Quantitative analysis of the average DiR fluorescence signal intensity shows that the magnetic field can increase the distribution of the drug in the lesion area. (C) H&E staining and CD31 staining of normal brain (left) tissue and ischemic lesion (right) tissue under different treatment conditions (scale bar: 20 μm). Reproduced with permission.122 Copyright 2020, American Chemical Society. | |
Micromotors and nanomotors are also considered to be a new generation of targeted and personalized DDSs, which have significant advantages over passive (non-motion) DDSs.127 In terms of power to control the targeted propulsion of micro/nano motors, magnetic force is a very promising candidate because it does not require fuel and can make the motor adapt to different environments due to its high controllability.128,129 For example, Sun et al. developed a pine pollen-based micromotor (PPBM) based on pine pollen encapsulated with magnetic particles and drugs.130 Due to the uniform packaging of a large number of magnetic nanoparticles, PPBM has superparamagnetism. Upon the application of different external magnetic fields, PPBM exhibited three motion modes: rolling, tumbling, and rotating. The propulsion ability of PPBM was further evaluated, and it was found that the translation speed of PPBM was proportional to the frequency of the magnetic field. Furthermore, PPBM was experimentally proved to navigate to a specific destination effortlessly, along a predetermined route by adjusting the frequency and direction of the external magnetic field. The above results showed that PPBM could be used as a magnetically controllable and effective delivery system. Under a high-intensity magnetic field, the magnetic nanoparticles in PPBM converged into a magnetic block, which acted as a magnetic rotor, generated a fluid field and released the encapsulated drug. In another work, Ceylan et al. designed a hydrogel-based magnetically driven micromotor.131 By using two-photon polymerization technology and applying a continuous magnetic field, a micromotor with uniform distribution of magnetic particles and a size similar to that of mammalian cells was successfully fabricated. A rotating magnetic field was realized by a six-coil electromagnetic design, and it was found that the moving speed of this micromotor increased linearly with the excitation frequency of the magnetic field. When driving the micromotor for long-distance motion at a frequency of 5 Hz, their average moving speed could even reach 3.36 ± 0.71 μm s−1. More importantly, through magnetic steering control, a small group of micromotors could be controlled for directional movement. The targeted drug release was achieved by the swelling of micromotor due to the appearance of matrix metalloproteinases highly expressed at the tumor site.
4.2 Internal magnetic implant responsive DDS
Magnetic targeted drug delivery usually requires an external magnetic field. However, applying an external magnetic field during a long period of treatment is cumbersome and expensive.132 The magnetic field generated by the internal magnetic implant might be able to solve this problem, and it is also one of the effective ways to transport drugs to deep tissues.133 For example, Lee et al. developed an implantable magnetic pump loaded with insulin that controlled the insulin release through an external magnetic field.134 As a next step, the magnetic pump was further improved by incorporating an actuator as the driver and reservoir with loading of exenatide.135 Each plunger and barrel of the actuator was equipped with a magnet for actuation. The plunger and barrel were embedded with multiple magnets of different shapes and polarities like a keyhole. By using a magnet with the same shape but opposite polarity as a key, the drug release could be realized. More importantly, this pump showed high stability, even after immersion in PBS (phosphate buffer saline) buffer for more than 28 d. The dose per actuation was still reproducible, while no drug leakage was observed within the detection limit of the experiment (as shown in Fig. 9A). H&E (hematoxylin & eosin) and MT (Masson Trichrome) staining were utilized to evaluate the formation of tissue fibrotic sacs (Fig. 9B). And the in vivo experimental results showed that, although it will cause mild inflammation at the implantation site, the inflammatory markers in blood did not change, indicating that it might not cause systemic adverse reactions. Furthermore, compared with an injection, this systemic administration approach had similar areas under the drug–time curves and basically the same therapeutic effect (as shown in Fig. 9C). It is also worth mentioning that this design was a semi-permanent and implantable magnetic switch pump, which could be continuously driven up to 300 times after implantation, while the replenishment of drugs and repeated use might be realized. Therefore, this system might serve as a representative strategy for on-demand drug delivery, with minimized needle injections. In another work, Wang et al. developed an intravitreal implantable DDS, mainly consisting of a micro check valve and magnetically actuated flexible membrane component.136 Upon driven by an external magnetic force, the micro check valve could be opened, which lead to drug release. By changing the intensity of the applied magnetic field and stimulation number, the precise release of the drug at the nanoscale could be adjusted. Furthermore, after implanting this magnetic micropump in rabbit vitreous, the drug could effectively reach the macular area of rabbit eyes by the induction of a magnetic field.
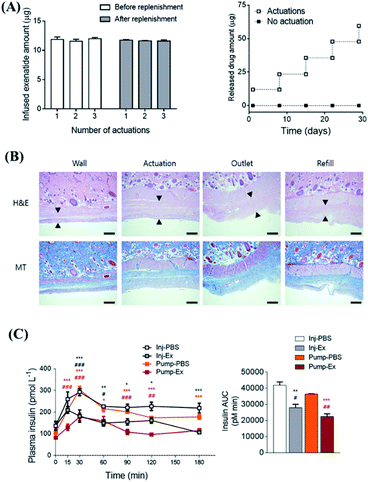 |
| Fig. 9 Implantable, battery-free magnetically responsive pump as a validation of a drug delivery system. (A) In vitro performance test of drug delivery stability of the magnetic response pump. (B) Testing the biocompatibility of the magnetic response pump in different parts by H&E and MT staining. The scale bars are 100 μm. (C) In vivo pharmacodynamic experiment of insulin changes caused by in vivo administration of exenatide-loaded magnetic response pump infusion per actuation with up to 300 consecutive actuations. Reproduced with permission.135 Copyright 2020, Elsevier. | |
In short, the application of an external magnetic force to control the navigation of a DDS and on-demand release of drugs is a promising strategy. However, most of the current magnetically responsive DDS research remains in its infant state, while it remains very difficult to design an effective magnetic field-controlled delivery system for precise delivery which is suitable for clinical applications.
5. Conclusion and perspective
In this review, the current reports on mechanical force responsive DDSs are summarized, mainly by considering the source of force stimulus in terms of endogenous biomechanical force and indirect mechanical force from in vitro sources. In the DDS that responds to endogenous biomechanics, a DDS mediated by endogenous compression force, tension, and shear force has been discussed, with focus on the structural design or biomimetic DDS. It is worth mentioning that a number of exciting mechanical response DDSs have been reported, with unique behavior such as long-term stability inside the body,20 controllable release of drugs,67 high safety without allergic reactions,77 and targeting ability.126 However, most systems still require further research before clinical practice, as most studies only involve animal model studies or even an in vitro drug release study only. Secondly, most of the current endogenous mechanical response drug delivery systems are mainly focused on mechanical forces, which are very important in the various life activities of cells.18 However, due to the unclear specific mechanism of mechanical forces affecting cell life activities and the lack of methods to detect cellular level mechanical forces in vivo,137–139 currently only one work from Lei and Tang have verified this concept. In details, upon activation by homologous antigens and T cell receptors (TCR), the binding force exerted by T cells could be utilized to control the drug release from mesoporous silica particles in situ.23 In this case, the exploration of this small but precise endogenous biomechanical force source might be a key issue for the design of the next generation of mechanically responsive DDSs. On the other hand, exogenously responsive mechanically DDSs are more practical and promising to achieve precise delivery of drugs to deep parts of the human body and accurately control drug release. One of the effective methods is to implant the DDS into the lesion, while external magnetic force or ultrasound could be designed to control the on-demand release of the drug. However, this method requires surgical intervention, and also requires high safety, tissue compliance, or stability of the DDS. Furthermore, by rational design of a magnetic navigation system through the first electromagnetic theory, it might be possible to accurately guide the movement of the system and achieve precise positioning or drug delivery.118 For more precise positioning, researchers have combined ultrasound and magnetic imaging to combine ultrasound positioning, magnetic navigation, and appropriate stimulation to achieve precise positioning and release of drugs.140–142 More interestingly, on-demand drug release could be controlled by changing the stimulus intensity in a linear manner. Furthermore, a recent design of a micromotor could also serve as a new generation DDS, which could be driven by external ultrasound or magnetic force to control its autonomous movement. This DDS could be externally driven or autonomously pushed for local drug delivery, at a pre-determined site through directional movement (i.e. Paul Ehrlich idea of a “magic bullet”),143 rather than relying on local fluid gradients or blood circulation.144,145 More interestingly, the micromotor could be designed to have board stimulation sources in terms of ultrasound or magnetic force,17,119,146,147 indicating its promising future as a next-generation drug delivery system.
In short, in the past few years, mechanically responsive DDSs have been greatly expanded due to the introduction of novel designs or biomaterials. The dynamic characteristics of the human body are constantly subjected to forces, and these mechanical forces are of great significance to the development, activation, migration and other life activities of tissues or cells. Therefore, mechanically responsive drug delivery systems are more widely used than other stimulus-responsive drug delivery systems, while it has been validated in various disease models such as cancer, diabetes, cardiovascular disease, wound healing, and urinary tract infection. As a typical example, a mechanical force-responsive drug delivery system has great potential in clinical translational applications, such as the hybrid hydrogel construct with bacterial cellulose nanofibers (BCNFs) which are triggered by compressive stress to release drugs on-demand and to control the dosage of drugs in clinical applications.34 Therefore, although more and more efforts should be made to use mechanical force to trigger the design of a DDS both preclinically and clinically, using mechanical stimulation as a drug delivery response trigger is a very promising approach.
Author contributions
Conceptualization: Z. L., C. W. and Y.-L. W.; literature review and writing—original draft preparation: P. M., X. L., Z. L., E. Y. and Y. C.; writing—review and editing and supervision: Z. L., C. W., X. J. L., and Y.-L. W. P. M. and X. L. contributed equally to this work. All authors have read and agreed to the current version of the manuscript.
Conflicts of interest
There are no conflicts to declare.
Acknowledgements
The authors would like to thank the National Key R&D Program of China (Grant No. 2020YFA0908100), and the Natural Science Foundation of China (81971724 and 82173750) for the support of this project. Z. L. would also like to express gratitude to the financial support from A*STAR, Science and Engineering Research Council (SERC) Central Research Fund (Use-inspired Basic Research) for this work.
References
- Y. Zhang, J. Yu, H. N. Bomba, Y. Zhu and Z. Gu, Mechanical Force-Triggered Drug Delivery, Chem. Rev., 2016, 116(19), 12536–12563 CrossRef CAS PubMed.
- R. T. Rozenbaum, O. C. J. Andrén, H. C. van der Mei, W. Woudstra, H. J. Busscher, M. Malkoch and P. K. Sharma, Penetration and Accumulation of Dendrons with Different Peripheral Composition in Pseudomonas aeruginosa Biofilms, Nano Lett., 2019, 19(7), 4327–4333 CrossRef CAS PubMed.
- L. J. C. Albuquerque, V. Sincari, A. Jäger, J. Kucka, J. Humajova, J. Pankrac, P. Paral, T. Heizer, O. Janouškova, I. Davidovich, Y. Talmon, P. Pouckova, P. Štěpánek, L. Sefc, M. Hruby, F. C. Giacomelli and E. Jäger, pH-responsive polymersome-mediated delivery of doxorubicin into tumor sites enhances the therapeutic efficacy and reduces cardiotoxic effects, J. Controlled Release, 2021, 332, 529–538 CrossRef CAS PubMed.
- W. Zhang, Y. Wen, D.-X. He, Y.-F. Wang, X.-L. Liu, C. Li and X.-J. Liang, Near-infrared AIEgens as transformers to enhance tumor treatment efficacy with controllable self-assembled redox-responsive carrier-free nanodrug, Biomaterials, 2019, 193, 12–21 CrossRef CAS PubMed.
- J. Yang, S. Pan, S. Gao, Y. Dai and H. Xu, Anti-recurrence/metastasis and chemosensitization therapy with thioredoxin reductase-interfering drug delivery system, Biomaterials, 2020, 249, 120054 CrossRef CAS PubMed.
- T. X. Zhang, Z. Z. Zhang, Y. X. Yue, X. Y. Hu, F. Huang, L. Shi, Y. Liu and D. S. Guo, A General Hypoxia-Responsive Molecular Container for Tumor-Targeted Therapy, Adv. Mater., 2020, 32(28), e1908435 CrossRef PubMed.
- Y. Li, J. Ding, X. Xu, R. Shi, P. E. Saw, J. Wang, S. Chung, W. Li, B. M. Aljaeid, R. J. Lee, W. Tao, L. Teng, O. C. Farokhzad and J. Shi, Dual Hypoxia-Targeting RNAi Nanomedicine for Precision Cancer Therapy, Nano Lett., 2020, 20(7), 4857–4863 CrossRef CAS PubMed.
- M. Han, M. Y. Huang-Fu, W. W. Guo, N. N. Guo, J. Chen, H. N. Liu, Z. Q. Xie, M. T. Lin, Q. C. Wei and J. Q. Gao, MMP-2-Sensitive HA End-Conjugated Poly (amidoamine) Dendrimers via Click Reaction To Enhance Drug Penetration into Solid Tumor, ACS Appl. Mater. Interfaces, 2017, 9(49), 42459–42470 CrossRef CAS PubMed.
- C. Wang, S. Chen, Y. Wang, X. Liu, F. Hu, J. Sun and H. Yuan, Lipase-Triggered Water-Responsive “Pandora's Box” for Cancer Therapy: Toward Induced Neighboring Effect and Enhanced Drug Penetration, Adv. Mater., 2018, 30(14), e1706407 CrossRef PubMed.
- H. P. Lee and A. K. Gaharwar, Light-Responsive Inorganic Biomaterials for Biomedical Applications, Adv. Sci., 2020, 7(17), 2000863 CrossRef CAS PubMed.
- W. Zhao, Y. Zhao, Q. Wang, T. Liu, J. Sun and R. Zhang, Remote Light-Responsive Nanocarriers for Controlled Drug Delivery: Advances and Perspectives, Small, 2019, 15(45), e1903060 CrossRef PubMed.
- M. Karimi, P. Sahandi Zangabad, A. Ghasemi, M. Amiri, M. Bahrami, H. Malekzad, H. Ghahramanzadeh Asl, Z. Mahdieh, M. Bozorgomid, A. Ghasemi, M. R. Rahmani Taji Boyuk and M. R. Hamblin, Temperature-Responsive Smart Nanocarriers for Delivery Of Therapeutic Agents: Applications and Recent Advances, ACS Appl. Mater. Interfaces, 2016, 8(33), 21107–21133 CrossRef CAS PubMed.
- D. Zhang, C. Yang, Z. Niu, C. Wang, S. Mukherjee, D. Wang, X. Li, R. Liu, J. Gao and Y. Chen, Construction of Crowning β-cyclodextrin with Temperature Response and Efficient Properties of Host-Guest Inclusion, Langmuir, 2018, 34(38), 11567–11574 CrossRef CAS PubMed.
- X. Hu, T. Zeng, C. C. Husic and M. J. Robb, Mechanically Triggered Small Molecule Release from a Masked Furfuryl Carbonate, J. Am. Chem. Soc., 2019, 141(38), 15018–15023 CrossRef CAS PubMed.
- W. Sun, H. Jiang, X. Wu, Z. Xu, C. Yao, J. Wang, M. Qin, Q. Jiang, W. Wang and D. Shi, Strong dual-crosslinked hydrogels for ultrasound-triggered drug delivery, Nano Res., 2019, 12(1), 115–119 CrossRef CAS.
- H. Xu, M. Medina-Sánchez, V. Magdanz, L. Schwarz, F. Hebenstreit and O. G. Schmidt, Sperm-Hybrid Micromotor for Targeted Drug Delivery, ACS Nano, 2018, 12(1), 327–337 CrossRef CAS PubMed.
- Y. Dong, L. Wang, J. Wang, S. Wang, Y. Wang, D. Jin, P. Chen, W. Du, L. Zhang and B. F. Liu, Graphene-Based Helical Micromotors Constructed by “Microscale Liquid Rope-Coil Effect” with Microfluidics, ACS Nano, 2020, 14, 16600–16613 CrossRef CAS PubMed.
- J. Wang, J. A. Kaplan, Y. L. Colson and M. W. Grinstaff, Mechanoresponsive materials for drug delivery: Harnessing forces for controlled release, Adv. Drug Delivery Rev., 2017, 108, 68–82 CrossRef CAS PubMed.
- V. Vogel and M. Sheetz, Local force and geometry sensing regulate cell functions, Nat. Rev. Mol. Cell Biol., 2006, 7(4), 265–275 CrossRef CAS PubMed.
- B. Mohanraj, G. Duan, A. Peredo, M. Kim, F. Tu, D. Lee, G. R. Dodge and R. L. Mauck, Mechanically-Activated Microcapsules for 'On-Demand' Drug Delivery in Dynamically Loaded Musculoskeletal Tissues, Adv. Funct. Mater., 2019, 29(15), 1807909 CrossRef PubMed.
- C. Chen, S. Li, C. Hu, W. Cao, Q. Fu, J. Li, L. Zheng and J. Huang, Protective Effects of Puerarin on Premature Ovarian Failure via Regulation of Wnt/β-catenin Signaling Pathway and Oxidative Stress, Reprod. Sci., 2021, 28(4), 982–990 CrossRef CAS PubMed.
- O. Rifaie-Graham, N. F. B. Galensowske, C. Dean, J. Pollard, S. Balog, M. G. Gouveia, M. Chami, A. Vian, E. Amstad, M. Lattuada and N. Bruns, Shear Stress-Responsive Polymersome Nanoreactors Inspired by the Marine Bioluminescence of Dinoflagellates, Angew. Chem., Int. Ed. Engl., 2021, 60(2), 904–909 CrossRef CAS PubMed.
- K. Lei and L. Tang, T cell force-responsive delivery of anticancer drugs using mesoporous silica microparticles, Mater. Horiz., 2020, 7(12), 3196–3200 RSC.
- S. R. Sirsi and M. A. Borden, State-of-the-art materials for ultrasound-triggered drug delivery, Adv. Drug Delivery Rev., 2014, 72, 3–14 CrossRef CAS PubMed.
- J. Thévenot, H. Oliveira, O. Sandre and S. Lecommandoux, Magnetic responsive polymer composite materials, Chem. Soc. Rev., 2013, 42(17), 7099–7116 RSC.
- T. Boissenot, A. Bordat, E. Fattal and N. Tsapis, Ultrasound-triggered drug delivery for cancer treatment using drug delivery systems: From theoretical considerations to practical applications, J. Controlled Release, 2016, 241, 144–163 CrossRef CAS PubMed.
- Y. Qiu, S. Tong, L. Zhang, Y. Sakurai, D. R. Myers, L. Hong, W. A. Lam and G. Bao, Magnetic forces enable controlled drug delivery by disrupting endothelial
cell-cell junctions, Nat. Commun., 2017, 8, 15594 CrossRef CAS PubMed.
- T. Sun, A. Dasgupta, Z. Zhao, M. Nurunnabi and S. Mitragotri, Physical triggering strategies for drug delivery, Adv. Drug Delivery Rev., 2020, 158, 36–62 CrossRef CAS PubMed.
- Y. J. Zhu and F. Chen, pH-responsive drug-delivery systems, Chem. – Asian J., 2015, 10(2), 284–305 CrossRef CAS PubMed.
- M. Shahriari, M. Zahiri, K. Abnous, S. M. Taghdisi, M. Ramezani and M. Alibolandi, Enzyme responsive drug delivery systems in cancer treatment, J. Controlled Release, 2019, 308, 172–189 CrossRef CAS PubMed.
- K. Chida, T. Yoshii, K. Takahashi, M. Yamamoto, K. Kanamaru, M. Ohwada, V. Deerattrakul, J. Maruyama, K. Kamiya, Y. Hayasaka, M. Inoue, F. Tani and H. Nishihara, Force-responsive ordered carbonaceous frameworks synthesized from Ni-porphyrin, Chem. Commun., 2021, 57(49), 6007–6010 RSC.
- A. B. Cook and P. Decuzzi, Harnessing Endogenous Stimuli for Responsive Materials in Theranostics, ACS Nano, 2021, 15(2), 2068–2098 CrossRef CAS PubMed.
- S. Huo, P. Zhao, Z. Shi, M. Zou, X. Yang, E. Warszawik, M. Loznik, R. Göstl and A. Herrmann, Mechanochemical bond scission for the activation of drugs, Nat. Chem., 2021, 13(2), 131–139 CrossRef CAS PubMed.
- D. Park, J. W. Kim, K. Shin and J. W. Kim, Bacterial cellulose nanofibrils-reinforced composite hydrogels for mechanical compression-responsive on-demand drug release, Carbohydr. Polym., 2021, 272, 118459 CrossRef CAS PubMed.
- P. Zhao, S. Huo, J. Fan, J. Chen, F. Kiessling, A. J. Boersma, R. Gostl and A. Herrmann, Activation of the Catalytic Activity of Thrombin for Fibrin Formation by Ultrasound, Angew. Chem., Int. Ed. Engl., 2021, 60(26), 14707–14714 CrossRef CAS PubMed.
- Y. Zhou, S. Huo, M. Loznik, R. Gostl, A. J. Boersma and A. Herrmann, Controlling Optical and Catalytic Activity of Genetically Engineered Proteins by Ultrasound, Angew. Chem., Int. Ed., 2021, 60(3), 1493–1497 CrossRef CAS PubMed.
- Y. Sun, W. J. Neary, Z. P. Burke, H. Qian, L. Zhu and J. S. Moore, Mechanically Triggered Carbon Monoxide Release with Turn-On Aggregation-Induced Emission, J. Am. Chem. Soc., 2022, 144(3), 1125–1129 CrossRef CAS PubMed.
- L. Tu, Z. Liao, Z. Luo, Y. L. Wu, A. Herrmann and S. Huo, Ultrasound-controlled drug release and drug activation for cancer therapy, Exploration, 2021, 1(3), 20210023 CrossRef.
- P. Cai, B. Hu, W. R. Leow, X. Wang, X. J. Loh, Y. L. Wu and X. Chen, Biomechano-Interactive Materials and Interfaces, Adv. Mater., 2018, 30(31), e1800572 CrossRef PubMed.
- N. Arora, J. P. Hazra and S. Rakshit, Anisotropy in mechanical unfolding of protein upon partner-assisted pulling and handle-assisted pulling, Commun. Biol., 2021, 4(1), 925 CrossRef CAS PubMed.
- C. C. Parkins, J. H. McAbee, L. Ruff, A. Wendler, R. Mair, R. J. Gilbertson, C. Watts and O. A. Scherman, Mechanically matching the rheological properties of brain tissue for drug-delivery in human glioblastoma models, Biomaterials, 2021, 276, 120919 CrossRef CAS PubMed.
- Y. Yang, J.-j. Tian, L. Wang, Z. Chen and S. Pu, D-π-A type carbazole and triphenylamine derivatives with different π-conjugated units: Tunable aggregation-induced emission (AIE) and mechanofluorochromic properties, J. Photochem. Photobiol., A, 2022, 429, 409–415 Search PubMed.
- A. Rana, E. Westein, B. Niego and C. E. Hagemeyer, Shear-Dependent Platelet Aggregation: Mechanisms and Therapeutic Opportunities, Front. Cardiovasc. Med., 2019, 6, 141 CrossRef CAS PubMed.
- K. Fang, R. Wang, H. Zhang, L. Zhou, T. Xu, Y. Xiao, Y. Zhou, G. Gao, J. Chen, D. Liu, F. Ai and J. Fu, Mechano-Responsive, Tough, and Antibacterial Zwitterionic Hydrogels with Controllable Drug Release for Wound Healing Applications, ACS Appl. Mater. Interfaces, 2020, 12(47), 52307–52318 CrossRef CAS PubMed.
- P. Y. Wang, Implantable reservoir for supplemental insulin delivery on demand by external compression, Biomaterials, 1989, 10(3), 197–201 CrossRef CAS PubMed.
- R. Chandan, S. Mehta and R. Banerjee, Ultrasound-Responsive Carriers for Therapeutic Applications, ACS Biomater. Sci. Eng., 2020, 6(9), 4731–4747 CrossRef CAS PubMed.
- F. Li, Y. Qin, J. Lee, H. Liao, N. Wang, T. P. Davis, R. Qiao and D. Ling, Stimuli-responsive nano-assemblies for remotely controlled drug delivery, J. Controlled Release, 2020, 322, 566–592 CrossRef CAS PubMed.
- K. Saravanakumar, X. Hu, D. M. Ali and M. H. Wang, Emerging Strategies in Stimuli-Responsive Nanocarriers as the Drug Delivery System for Enhanced Cancer Therapy, Curr. Pharm. Des., 2019, 25(24), 2609–2625 CrossRef CAS PubMed.
- A. Raza, T. Rasheed, F. Nabeel, U. Hayat, M. Bilal and H. M. N. Iqbal, Endogenous and Exogenous Stimuli-Responsive Drug Delivery Systems for Programmed Site-Specific Release, Molecules, 2019, 24(6), 1117 CrossRef CAS PubMed.
- Z. Li, Z. Wang, X. Du, C. Shi and X. Cui, Sonochemistry-Assembled Stimuli-Responsive Polymer Microcapsules for Drug Delivery, Adv. Healthcare Mater., 2018, 7(11), e1701326 CrossRef PubMed.
- L. Zhu, Z. Zhou, H. Mao and L. Yang, Magnetic nanoparticles for precision oncology: theranostic magnetic iron oxide nanoparticles for image-guided and targeted cancer therapy, Nanomedicine, 2017, 12(1), 73–87 CrossRef CAS PubMed.
- M. Maurer and J. Lammerding, The Driving Force: Nuclear Mechanotransduction in Cellular Function, Fate, and Disease, Annu. Rev. Biomed. Eng., 2019, 21, 443–468 CrossRef CAS PubMed.
- M. Valdivia, F. Vega-Macaya and P. Olguín, Mechanical Control of Myotendinous Junction Formation and Tendon Differentiation during Development, Frontiers in Cell and Developmental Biology, 2017, 5, 26 CrossRef PubMed.
- Y. Qiu and K. Park, Environment-sensitive hydrogels for drug delivery, Adv. Drug Delivery Rev., 2001, 53(3), 321–339 CrossRef CAS PubMed.
- K. M. Wiggins, J. N. Brantley and C. W. Bielawski, Methods for activating and characterizing mechanically responsive polymers, Chem. Soc. Rev., 2013, 42(17), 7130–7147 RSC.
- J. Chen, Q. Peng, X. Peng, L. Han, X. Wang, J. Wang and H. Zeng, Recent Advances in Mechano-Responsive Hydrogels for Biomedical Applications, ACS Appl. Polym. Mater., 2020, 2(3), 1092–1107 CrossRef CAS.
- J. Di, S. Yao, Y. Ye, Z. Cui, J. Yu, T. K. Ghosh, Y. Zhu and Z. Gu, Stretch-Triggered Drug Delivery from Wearable Elastomer Films Containing Therapeutic Depots, ACS Nano, 2015, 9(9), 9407–9415 CrossRef CAS PubMed.
- L. Wilde, M. Bock, G. Glöckl, G. Garbacz and W. Weitschies, Development of a pressure-sensitive glyceryl tristearate capsule filled with a drug-containing hydrogel, Int. J. Pharm., 2014, 461(1–2), 296–300 CrossRef CAS PubMed.
- M. P. Neubauer, M. Poehlmann and A. Fery, Microcapsule mechanics: from stability to function, Adv. Colloid Interface Sci., 2014, 207, 65–80 CrossRef CAS PubMed.
- C. E. Hansen, D. R. Myers, W. H. Baldwin, Y. Sakurai, S. L. Meeks, L. A. Lyon and W. A. Lam, Platelet-Microcapsule Hybrids Leverage Contractile Force for Targeted Delivery of Hemostatic Agents, ACS Nano, 2017, 11(6), 5579–5589 CrossRef CAS PubMed.
- B. Kim, S. Yoo, Y.-J. Kim, J. Park, B. Kang, S. Haam, S.-W. Kang, K. Kang and U. Jeong, A Strain-Regulated, Refillable Elastic Patch for Controlled Release, Adv. Mater. Interfaces, 2016, 3(9), 1500803 CrossRef.
- J. Wang, J. A. Kaplan, Y. L. Colson and M. W. Grinstaff, Stretch-Induced Drug Delivery from Superhydrophobic Polymer Composites: Use of Crack Propagation Failure Modes for Controlling Release Rates, Angew. Chem., Int. Ed., 2016, 55(8), 2796–2800 CrossRef CAS PubMed.
- D. C. Hyun, G. D. Moon, C. J. Park, B. S. Kim, Y. Xia and U. Jeong, Strain-Controlled Release of Molecules from Arrayed Microcapsules Supported on an Elastomer Substrate, Angew. Chem., Int. Ed., 2011, 50(3), 724–727 CrossRef CAS PubMed.
- Y. Zhang, Y. Gao, W. S. P. Carvalho, C. Fang and M. J. Serpe, Microgel-Based Stretchable Reservoir Devices for Elongation Enhanced Small Molecule Release Rate, ACS Appl. Mater. Interfaces, 2020, 12(16), 19062–19068 CrossRef CAS PubMed.
- L. Wei, L. Liu, C. Gao, C. Qian, K. Xi, H. Ruan, J. Yu, Z. P. Luo and W. Cui, Mechanical on-off gates for regulation of drug release in cutaneous or musculoskeletal tissue repairs, Mater. Sci. Eng., C, 2020, 115, 111048 CrossRef CAS PubMed.
- J. Wang, J. A. Kaplan, Y. L. Colson and M. W. Grinstaff, Stretch-Induced Drug Delivery from Superhydrophobic Polymer Composites: Use of Crack Propagation Failure Modes for Controlling Release Rates, Angew. Chem., Int. Ed. Engl., 2016, 55(8), 2796–2800 CrossRef CAS PubMed.
- H. Jeon, M. S. Kim, S. K. Hong, S. J. Cho and G. Lim, Tensile strain-controlled drug delivery system based on a cracked metal structure, Sens. Actuators, B, 2018, 270, 64–71 CrossRef CAS.
- K. Khanmohammadi Chenab, B. Sohrabi and A. Rahmanzadeh, Superhydrophobicity: advanced biological and biomedical applications, Biomater. Sci., 2019, 7(8), 3110–3137 RSC.
- E. J. Falde, S. T. Yohe, Y. L. Colson and M. W. Grinstaff, Superhydrophobic materials for biomedical applications, Biomaterials, 2016, 104, 87–103 CrossRef CAS PubMed.
- J. Wang, Y. L. Colson and M. W. Grinstaff, Tension-Activated Delivery of Small Molecules and Proteins from Superhydrophobic Composites, Adv. Healthcare Mater., 2018, 7(7), e1701096 CrossRef PubMed.
- M. Guvendiren, H. D. Lu and J. A. Burdick, Shear-thinning hydrogels for biomedical applications, Soft Matter, 2012, 8(2), 260–272 RSC.
- M. Epshtein and N. Korin, Shear targeted drug delivery to stenotic blood vessels, Journal of Biomechanics, 2017, 50, 217–221 CrossRef PubMed.
-
M. Buscema, H. Deyhle, T. Pfohl, S. E. Hieber, A. Zumbuehl and B. Müller in Characterization of Mechano-Sensitive Nano-Containers for Targeted Vasodilation, Bioinspiration, Biomimetics, and Bioreplication 2016, International Society for Optics and Photonics, 2016 Search PubMed.
- T. Natsume and M. Yoshimoto, Mechanosensitive liposomes as artificial chaperones for shear-driven acceleration of enzyme-catalyzed reaction, ACS Appl. Mater. Interfaces, 2014, 6(5), 3671–3679 CrossRef CAS PubMed.
- M. N. Holme, I. A. Fedotenko, D. Abegg, J. Althaus, L. Babel, F. Favarger, R. Reiter, R. Tanasescu, P. L. Zaffalon, A. Ziegler, B. Müller, T. Saxer and A. Zumbuehl, Shear-stress sensitive lenticular vesicles for targeted drug delivery, Nat. Nanotechnol., 2012, 7(8), 536–543 CrossRef CAS PubMed.
- J. Szebeni, Complement activation-related pseudoallergy: a stress reaction in blood triggered by nanomedicines and biologicals, Mol. Immunol., 2014, 61(2), 163–173 CrossRef CAS PubMed.
- M. Buscema, S. Matviykiv, T. Mészáros, G. Gerganova, A. Weinberger, U. Mettal, D. Mueller, F. Neuhaus, E. Stalder, T. Ishikawa, R. Urbanics, T. Saxer, T. Pfohl, J. Szebeni, A. Zumbuehl and B. Müller, Immunological response to nitroglycerin-loaded shear-responsive liposomes in vitro and in vivo, J. Controlled Release, 2017, 264, 14–23 CrossRef CAS PubMed.
- H. Zukerman, M. Khoury, Y. Shammay, J. Sznitman, N. Lotan and N. Korin, Targeting functionalized nanoparticles to activated endothelial cells under high wall shear stress, Bioeng. Transl. Med., 2020, 5(2), e10151 CAS.
- S. P. Jackson, W. S. Nesbitt and E. Westein, Dynamics of platelet thrombus formation, J. Thromb. Haemostasis, 2009, 7(Suppl 1), 17–20 CrossRef CAS PubMed.
- M. J. Maxwell, E. Westein, W. S. Nesbitt, S. Giuliano, S. M. Dopheide and S. P. Jackson, Identification of a 2-stage platelet aggregation process mediating shear-dependent thrombus formation, Blood, 2007, 109(2), 566–576 CrossRef CAS PubMed.
- H. Yang, Y. Song, J. Chen, Z. Pang, N. Zhang, J. Cao, Q. Wang, Q. Li, F. Zhang, Y. Dai, C. Li, Z. Huang, J. Qian and J. Ge, Platelet Membrane-Coated Nanoparticles Target Sclerotic Aortic Valves in ApoE(-/-) Mice by Multiple Binding Mechanisms Under Pathological Shear Stress, Int. J. Nanomed., 2020, 15, 901–912 CrossRef CAS PubMed.
- K.-H. Choi and J.-H. Kim, Therapeutic applications of ultrasound in neurological diseases, J. Neurosonol. Neuroimag., 2019, 11(1), 62–72 CrossRef.
- L. Meng, X. Liu, Y. Wang, W. Zhang, W. Zhou, F. Cai, F. Li, J. Wu, L. Xu, L. Niu and H. Zheng, Sonoporation of Cells by a Parallel Stable Cavitation Microbubble Array, Adv. Sci., 2019, 6(17), 1900557 CrossRef PubMed.
- J. N. Sloand, T. T. Nguyen, S. A. Zinck, E. C. Cook, T. J. Zimudzi, S. A. Showalter, A. B. Glick, J. C. Simon and S. H. Medina, Ultrasound-Guided Cytosolic Protein Delivery via Transient Fluorous Masks, ACS Nano, 2020, 14(4), 4061–4073 CrossRef CAS PubMed.
- J. Chen, S. Ratnayaka, A. Alford, V. Kozlovskaya, F. Liu, B. Xue, K. Hoyt and E. Kharlampieva, Theranostic Multilayer Capsules for Ultrasound Imaging and Guided Drug Delivery, ACS Nano, 2017, 11(3), 3135–3146 CrossRef CAS PubMed.
- P. Wu, Y. Jia, F. Qu, Y. Sun, P. Wang, K. Zhang, C. Xu, Q. Liu and X. Wang, Ultrasound-Responsive Polymeric Micelles for Sonoporation-Assisted Site-Specific Therapeutic Action, ACS Appl. Mater. Interfaces, 2017, 9(31), 25706–25716 CrossRef CAS PubMed.
- Z. Zhu, F. Huang, C. Yang, T. Si and R. X. Xu, On-Demand Generation of Double Emulsions Based on Interface Shearing for Controlled Ultrasound Activation, ACS Appl. Mater. Interfaces, 2019, 11(43), 40932–40943 CrossRef CAS PubMed.
- H. Horsley, J. Owen, R. Browning, D. Carugo, J. Malone-Lee, E. Stride and J. L. Rohn, Ultrasound-activated microbubbles as a novel intracellular drug delivery system for urinary tract infection, J. Controlled Release, 2019, 301, 166–175 CrossRef CAS PubMed.
- S. Roovers, T. Segers, G. Lajoinie, J. Deprez, M. Versluis, S. C. De Smedt and I. Lentacker, The Role of Ultrasound-Driven Microbubble Dynamics in Drug Delivery: From Microbubble Fundamentals to Clinical Translation, Langmuir, 2019, 35(31), 10173–10191 CrossRef CAS PubMed.
- S. Sun, P. Wang, S. Sun and X. Liang, Applications of Micro/Nanotechnology in Ultrasound-based Drug Delivery and Therapy for Tumor, Curr. Med. Chem., 2021, 28(3), 525–547 CrossRef CAS PubMed.
- D. Y. Zhang, C. Dmello, L. Chen, V. A. Arrieta, E. Gonzalez-Buendia, J. R. Kane, L. P. Magnusson, A. Baran, C. D. James, C. Horbinski, A. Carpentier, C. Desseaux, M. Canney, M. Muzzio, R. Stupp and A. M. Sonabend, Ultrasound-mediated Delivery of Paclitaxel for Glioma: A Comparative Study of Distribution, Toxicity, and Efficacy of Albumin-bound Versus Cremophor Formulations, Clin. Cancer Res., 2020, 26(2), 477–486 CrossRef CAS PubMed.
- A. H. Liao, C. H. Wang, P. Y. Weng, Y. C. Lin, H. Wang, H. K. Chen, H. L. Liu, H. C. Chuang and C. P. Shih, Ultrasound-induced microbubble cavitation via a transcanal or transcranial approach facilitates inner ear drug delivery, JCI Insight, 2020, 5(3), e132880 CrossRef PubMed.
- S. M. Chowdhury, L. Abou-Elkacem, T. Lee, J. Dahl and A. M. Lutz, Ultrasound and microbubble mediated therapeutic delivery: Underlying mechanisms and future outlook, J. Controlled Release, 2020, 326, 75–90 CrossRef CAS PubMed.
- W. Sun, Z. Li, X. Zhou, G. Yang and L. Yuan, Efficient exosome delivery in refractory tissues assisted by ultrasound-targeted microbubble destruction, Drug Delivery, 2019, 26(1), 45–50 CrossRef CAS PubMed.
- E. K. Juang, I. De Cock, C. Keravnou, M. K. Gallagher, S. B. Keller, Y. Zheng and M. Averkiou, Engineered 3D Microvascular Networks for the Study of Ultrasound-Microbubble-Mediated Drug Delivery, Langmuir, 2019, 35(31), 10128–10138 CrossRef CAS PubMed.
- J. Zhang, S. Wang, Z. Deng, L. Li, G. Tan, X. Liu, H. Zheng and F. Yan, Ultrasound-Triggered Drug Delivery for Breast Tumor Therapy Through iRGD-Targeted Paclitaxel-Loaded Liposome-Microbubble Complexes, J. Biomed. Nanotechnol., 2018, 14(8), 1384–1395 CrossRef CAS PubMed.
- D. V. B. Batchelor, R. H. Abou-Saleh, P. L. Coletta, J. R. McLaughlan, S. A. Peyman and S. D. Evans, Nested Nanobubbles for Ultrasound-Triggered Drug Release, ACS Appl. Mater. Interfaces, 2020, 12(26), 29085–29093 CAS.
- N. Rapoport, Drug-Loaded Perfluorocarbon Nanodroplets for Ultrasound-Mediated Drug Delivery, Adv. Exp. Med. Biol., 2016, 880, 221–241 CrossRef CAS PubMed.
- N. Rapoport, Phase-shift, stimuli-responsive perfluorocarbon nanodroplets for drug delivery to cancer, Wiley Interdiscip. Rev.: Nanomed. Nanobiotechnol., 2012, 4(5), 492–510 CAS.
- M. G. Freire, P. J. Carvalho, L. M. Santos, L. R. Gomes, I. M. Marrucho and J. A. Coutinho, Solubility of water in fluorocarbons: Experimental and COSMO-RS prediction results, J. Chem. Thermodyn., 2010, 42(2), 213–219 CrossRef CAS.
- N. Y. Rapoport, A. L. Efros, D. A. Christensen, A. M. Kennedy and K. H. Nam, Microbubble Generation in Phase-Shift Nanoemulsions used as Anticancer Drug Carriers, Bubble Sci., Eng., Technol., 2009, 1(1–2), 31–39 CrossRef CAS PubMed.
- N. Reznik, G. Lajoinie, O. Shpak, E. C. Gelderblom, R. Williams, N. de Jong, M. Versluis and P. N. Burns, On the acoustic properties of vaporized submicron perfluorocarbon droplets, Ultrasound in Medicine and Biology, 2014, 40(6), 1379–1384 CrossRef PubMed.
- Y. Cao, Y. Chen, T. Yu, Y. Guo, F. Liu, Y. Yao, P. Li, D. Wang, Z. Wang, Y. Chen and H. Ran, Drug Release from Phase-Changeable Nanodroplets Triggered by Low-Intensity Focused Ultrasound, Theranostics, 2018, 8(5), 1327–1339 CrossRef CAS PubMed.
- Q. Zhong, B. C. Yoon, M. Aryal, J. B. Wang, T. Ilovitsh, M. A. Baikoghli, N. Hosseini-Nassab, A. Karthik, R. H. Cheng, K. W. Ferrara and R. D. Airan, Polymeric perfluorocarbon nanoemulsions are ultrasound-activated wireless drug infusion catheters, Biomaterials, 2019, 206, 73–86 CrossRef CAS PubMed.
- A. Jain, A. Tiwari, A. Verma and S. K. Jain, Ultrasound-based triggered drug delivery to tumors, Drug Delivery Transl. Res., 2018, 8(1), 150–164 CrossRef CAS PubMed.
- S. Quader and K. Kataoka, Nanomaterial-Enabled Cancer Therapy, Mol. Ther., 2017, 25(7), 1501–1513 CrossRef CAS PubMed.
- U. Kauscher, M. N. Holme, M. Björnmalm and M. M. Stevens, Physical stimuli-responsive vesicles in drug delivery: Beyond liposomes and polymersomes, Adv. Drug Delivery Rev., 2019, 138, 259–275 CrossRef CAS PubMed.
- N. S. Awad, V. Paul, M. S. Mahmoud, N. M. Al Sawaftah, P. S. Kawak, M. H. Al Sayah and G. A. Husseini, Effect of Pegylation and Targeting Moieties on the Ultrasound-Mediated Drug Release from Liposomes, ACS Biomater. Sci. Eng., 2020, 6(1), 48–57 CrossRef CAS PubMed.
- W. C. Huang, F. Ali, J. Zhao, K. Rhee, C. Mou and C. J. Bettinger, Ultrasound-Mediated Self-Healing Hydrogels Based on Tunable Metal-Organic Bonding, Biomacromolecules, 2017, 18(4), 1162–1171 CrossRef CAS PubMed.
- J. Y. Sun, X. Zhao, W. R. Illeperuma, O. Chaudhuri, K. H. Oh, D. J. Mooney, J. J. Vlassak and Z. Suo, Highly stretchable and tough hydrogels, Nature, 2012, 489(7414), 133–136 CrossRef CAS PubMed.
- A. Gholami, S. M. Mousavi, S. A. Hashemi, Y. Ghasemi, W.-H. Chiang and N. Parvin, Current trends in chemical modifications of magnetic nanoparticles for targeted drug delivery in cancer chemotherapy, Drug Metab. Rev., 2020, 52(1), 205–224 CAS.
- P. M. Price, W. E. Mahmoud, A. A. Al-Ghamdi and L. M. Bronstein, Magnetic Drug Delivery: Where the Field Is Going, Front. Chem., 2018, 6, 619 CrossRef CAS PubMed.
- Y. Liu, J. Li, H. Chen, Y. Cai, T. Sheng, P. Wang, Z. Li, F. Yang and N. Gu, Magnet-activatable nanoliposomes as intracellular bubble microreactors to enhance drug delivery efficacy and burst cancer cells, Nanoscale, 2019, 11(40), 18854–18865 RSC.
- S. Liu, X. Chen, L. Bao, T. Liu, P. Yuan, X. Yang, X. Qiu, J. J. Gooding, Y. Bai, J. Xiao, F. Pu and Y. Jin, Treatment of infarcted heart tissue via the capture and local delivery of circulating exosomes through antibody-conjugated magnetic nanoparticles, Nat. Biomed. Eng., 2020, 4(11), 1063–1075 CrossRef CAS PubMed.
- J. Supramaniam, R. Adnan, N. H. M. Kaus and R. Bushra, Magnetic nanocellulose alginate hydrogel beads as potential drug delivery system, Int. J. Biol. Macromol., 2018, 118, 640–648 CrossRef CAS PubMed.
- M. Rahimi, K. D. Safa and R. Salehi, Co-delivery of doxorubicin and methotrexate by dendritic chitosan-g-mPEG as a magnetic nanocarrier for multi-drug delivery in combination chemotherapy, Polym. Chem., 2017, 8(47), 7333–7350 RSC.
- S. Persano, P. Das and T. Pellegrino, Magnetic Nanostructures as Emerging Therapeutic Tools to Boost Anti-Tumour Immunity, Cancers, 2021, 13(11), 2735 CrossRef CAS PubMed.
- Y. L. Liu, D. Chen, P. Shang and D. C. Yin, A review of magnet systems for targeted drug delivery, J. Controlled Release, 2019, 302, 90–104 CrossRef CAS PubMed.
- V. M. Kadiri, C. Bussi, A. W. Holle, K. Son, H. Kwon, G. Schütz, M. G. Gutierrez and P. Fischer, Biocompatible Magnetic Micro- and Nanodevices: Fabrication of FePt Nanopropellers and Cell Transfection, Adv. Mater., 2020, 32(25), e2001114 CrossRef PubMed.
- H. Jin, P. Afiuny, S. Dove, G. Furlan, M. Zakotnik, Y. Yih and J. W. Sutherland, Life Cycle Assessment of Neodymium-Iron-Boron Magnet-to-Magnet Recycling for Electric Vehicle Motors, Environ. Sci. Technol., 2018, 52(6), 3796–3802 CrossRef CAS PubMed.
- B. Chertok, A. E. David and V. C. Yang, Brain tumor targeting of magnetic nanoparticles for potential drug delivery: effect of administration route and magnetic field topography, J. Controlled Release, 2011, 155(3), 393–399 CrossRef CAS PubMed.
- M. Li, J. Li, J. Chen, Y. Liu, X. Cheng, F. Yang and N. Gu, Platelet Membrane Biomimetic Magnetic Nanocarriers for Targeted Delivery and in Situ Generation of Nitric Oxide in Early Ischemic Stroke, ACS Nano, 2020, 14(2), 2024–2035 CrossRef CAS PubMed.
- B. Shapiro, Towards dynamic control of magnetic fields to focus magnetic carriers to targets deep inside the body, J. Magn. Magn. Mater., 2009, 321(10), 1594 CrossRef CAS PubMed.
- M. Shamsi, A. Sedaghatkish, M. Dejam, M. Saghafian, M. Mohammadi and A. Sanati-Nezhad, Magnetically assisted intraperitoneal drug delivery for cancer chemotherapy, Drug Delivery, 2018, 25(1), 846–861 CrossRef CAS PubMed.
- P. Shojaee, H. Niroomand-Oscuii, M. Sefidgar and L. Alinezhad, Effect of nanoparticle size, magnetic intensity, and tumor distance on the distribution of the magnetic nanoparticles in a heterogeneous tumor microenvironment, J. Magn. Magn. Mater., 2020, 498, 166089 CrossRef CAS.
- Y. Qiao, X. Liu, B. Li, Y. Han, Y. Zheng, K. W. K. Yeung, C. Li, Z. Cui, Y. Liang, Z. Li, S. Zhu, X. Wang and S. Wu, Treatment of MRSA-infected osteomyelitis using bacterial capturing, magnetically targeted composites with microwave-assisted bacterial killing, Nat. Commun., 2020, 11(1), 4446 CrossRef CAS PubMed.
- M. Medina-Sánchez, H. Xu and O. G. Schmidt, Micro- and nano-motors: the new generation of drug carriers, Ther. Delivery, 2018, 9(4), 303–316 CrossRef PubMed.
- H. Choi, J. Yi, S. H. Cho and S. K. Hahn, Multifunctional micro/nanomotors as an emerging platform for smart healthcare applications, Biomaterials, 2021, 279, 121201 CrossRef CAS PubMed.
- S. H. Wang, K. Liu, F. Wang, F. Peng and Y. F. Tu, The Application of Micro- and Nanomotors in Classified Drug Delivery, Chem. - Asian J., 2019, 14(14), 2336–2347 CrossRef CAS PubMed.
- M. M. Sun, X. J. Fan, X. H. Meng, J. M. Song, W. N. Chen, L. N. Sun and H. Xie, Magnetic biohybrid micromotors with high maneuverability for efficient drug loading and targeted drug delivery, NANOSCALE, 2019, 11(39), 18382–18392 RSC.
- H. Ceylan, I. C. Yasa, O. Yasa, A. F. Tabak, J. Giltinan and M. Sitti, 3D-Printed Biodegradable Microswimmer for Theranostic Cargo Delivery and Release, ACS Nano, 2019, 13(3), 3353–3362 CrossRef CAS PubMed.
- M. Y. Hua, H. L. Liu, H. W. Yang, P. Y. Chen, R. Y. Tsai, C. Y. Huang, I. C. Tseng, L. A. Lyu, C. C. Ma, H. J. Tang, T. C. Yen and K. C. Wei, The effectiveness of a magnetic nanoparticle-based delivery system for BCNU in the treatment of gliomas, Biomaterials, 2011, 32(2), 516–527 CrossRef CAS PubMed.
- J. Ge, Y. Zhang, Z. Dong, J. Jia, J. Zhu, X. Miao and B. Yan, Initiation of Targeted Nanodrug Delivery in Vivo by a Multifunctional Magnetic Implant, ACS Appl. Mater. Interfaces, 2017, 9(24), 20771–20778 CrossRef CAS PubMed.
- S. H. Lee, Y. B. Lee, B. H. Kim, C. Lee, Y. M. Cho, S. N. Kim, C. G. Park, Y. C. Cho and Y. B. Choy, Implantable batteryless device for on-demand and pulsatile insulin administration, Nat. Commun., 2017, 8, 15032 CrossRef CAS PubMed.
- S. H. Lee, S. H. Min, Y. C. Cho, J. H. Han, M. N. Kim, C. R. Kim, C. H. Ahn, B. H. Kim, C. Lee, Y. M. Cho and Y. B. Choy, Magnetically-driven implantable pump for on-demand bolus infusion of short-acting glucagon-like peptide-1 receptor agonist, J. Controlled Release, 2020, 325, 111–120 CrossRef CAS PubMed.
- C. Wang, S. J. Seo, J. S. Kim, S. H. Lee, J. K. Jeon, J. W. Kim, K. H. Kim, J. K. Kim and J. Park, Intravitreal implantable magnetic micropump for on-demand VEGFR-targeted drug delivery, J. Controlled Release, 2018, 283, 105–112 CrossRef CAS PubMed.
- D. Vorselen, Y. Wang, M. M. de Jesus, P. K. Shah, M. J. Footer, M. Huse, W. Cai and J. A. Theriot, Microparticle traction force microscopy reveals subcellular force exertion patterns in immune cell-target interactions, Nat. Commun., 2020, 11(1), 20 CrossRef CAS PubMed.
- K. Lei, A. Kurum and L. Tang, Mechanical Immunoengineering of T cells for Therapeutic Applications, Acc. Chem. Res., 2020, 53(12), 2777–2790 CrossRef CAS PubMed.
- J. W. Hickey, Y. Dong, J. W. Chung, S. F. Salathe, H. C. Pruitt, X. Li, C. Chang, A. K. Fraser, C. A. Bessell, A. J. Ewald, S. Gerecht, H. Q. Mao and J. P. Schneck, Engineering an Artificial T-Cell Stimulating Matrix for Immunotherapy, Adv. Mater., 2019, 31(23), e1807359 CrossRef PubMed.
- Ş. Hamarat Şanlıer, G. Ak, H. Yılmaz, A. Ünal, F. Bozkaya Ü, G. Tanıyan, Y. Yıldırım and G. Yıldız Türkyılmaz, Development of Ultrasound-Triggered and Magnetic-Targeted Nanobubble System for Dual-Drug Delivery, J. Pharm. Sci., 2019, 108(3), 1272–1283 CrossRef PubMed.
- B. Chertok and R. Langer, Circulating Magnetic Microbubbles for Localized Real-Time Control of Drug Delivery by Ultrasonography-Guided Magnetic Targeting and Ultrasound, Theranostics, 2018, 8(2), 341–357 CrossRef CAS PubMed.
- N. Hijnen, E. Kneepkens, M. de Smet, S. Langereis, E. Heijman and H. Grüll, Thermal combination therapies for local drug delivery by magnetic resonance-guided high-intensity focused ultrasound, Proc. Natl. Acad. Sci. U. S. A., 2017, 114(24), E4802–e4811 CrossRef CAS PubMed.
- K. Strebhardt and A. Ullrich, Paul Ehrlich's magic bullet concept: 100 years of progress, Nat. Rev. Cancer, 2008, 8(6), 473–480 CrossRef CAS PubMed.
- K. Yuan, Z. Jiang, B. Jurado-Sánchez and A. Escarpa, Nano/Micromotors for Diagnosis and Therapy of Cancer and Infectious Diseases, Chemistry, 2020, 26(11), 2309–2326 CrossRef CAS PubMed.
- S. K. Srivastava, G. Clergeaud, T. L. Andresen and A. Boisen, Micromotors for drug delivery in vivo: The road ahead, Adv. Drug Delivery Rev., 2019, 138, 41–55 CrossRef CAS PubMed.
- J. Wang, X. Liu, Y. Qi, Z. Liu, Y. Cai and R. Dong, Ultrasound-propelled nanomotors for improving antigens cross-presentation and cellular immunity, Chem. Eng. J., 2021, 416, 129091 CrossRef CAS.
- A. V. Singh, M. H. Dad Ansari, C. B. Dayan, J. Giltinan, S. Wang, Y. Yu, V. Kishore, P. Laux, A. Luch and M. Sitti, Multifunctional magnetic hairbot for untethered osteogenesis, ultrasound contrast imaging and drug delivery, Biomaterials, 2019, 219, 119394 CrossRef CAS PubMed.
|
This journal is © The Royal Society of Chemistry 2022 |
Click here to see how this site uses Cookies. View our privacy policy here.