DOI:
10.1039/D2NJ00816E
(Perspective)
New J. Chem., 2022,
46, 8939-8966
From truxenes to heterotruxenes: playing with heteroatoms and the symmetry of molecules
Received
17th February 2022
, Accepted 22nd March 2022
First published on 24th March 2022
Abstract
The truxene molecule is more versatile and widespread than ever imagined. At the end of the last century, this star-shaped structure was recognized mostly in liquid crystals. However, the development of optoelectronics resulted in a revolution in truxenes. Stable, fast, easily processed and cheap optoelectronic devices are desirable in many areas of industry and science. Consequently, the contribution of truxenes to these fields is manifold, ranging from D–A emitters, semiconductors, and complexes to sensors. They attract attention because of their relatively low mass and expanded π-electron system. Symmetric molecules give rise to dendrimers, bowl-shape molecules and fullerenes. Further advantages are shown by the recently discovered non-symmetric truxenes obtained via one- or two-heteroatom displacement. Thus, the chemistry of new heteroaromatic compounds has been expanded.
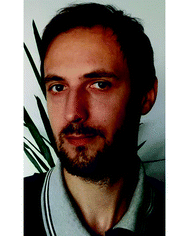
Krzysztof Górski
| Dr Krzysztof Górski received his MSc from Warsaw University in 2012 under the supervision of Professor Marek Pietraszkiewicz and Professor Andrzej Kudelski. He continued his research on heterotruxenes at the Institute of Physical Chemistry of the Polish Academy of Sciences, where he obtained his PhD in 2020 under the supervision of Professor Marek Pietraszkiewicz. Currently, he is a Postdoctoral Researcher in Prof. Daniel Gryko's group. His research interests are focused on the synthesis and physicochemistry of (hetero)aromatic functional dyes. |
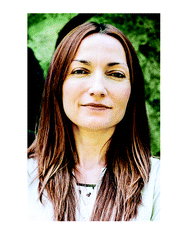
Justyna Mech-Piskorz
| Dr Justyna Mech-Piskorz was born in Bochnia (Poland). She graduated with her MSc Eng. from Jagiellonian University, specializing in photonics and nanotechnology and supervised by Dr Jerzy Zachorowicz. During her PhD studies, she participated in interdisciplinary studies involving chemistry and solid state physics. She graduated with a PhD from AGH University of Science and Technology, supervised by Prof. Konrad Szaciłowski in 2014. She started her own project at the Institute of Physical Chemistry PAS in 2015 concerning the physicochemical aspects of hybrid materials. Currently, she is working on non-linear effects in truxenes and its hybrids. |
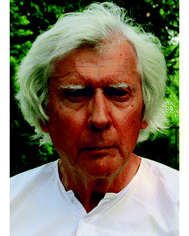
Marek Pietraszkiewicz
| Prof. Marek Pietraszkiewicz was born in Warsaw (Poland). He obtained his PhD at the Institute of Organic Chemistry, Polish Academy of Sciences, in 1978, with Prof. Osman Achmatowicz as his supervisor. He was a Post-Doc Researcher at Orlean University, supervised by Prof. Pierre Sinay, working on the total synthesis of cerulenin antibiotics. He held a second Post-Doc position at University Louis Pasteur, Strasbourg, France, supervised by Prof. Jean-Marie Lehn, working on photoluminescent lanthanide complexes. He obtained his DSci (habilitation) at the Institute of Physical Chemistry, Polish Academy of Sciences. His scientific research interests include organic synthesis, supramolecular chemistry, lanthanide photoluminescent complexes, and materials for organic electronics. He is the founder and chairman of the Polish and Global Supramolecular Chemistry Networks. |
1. Introduction
Truxene is a C3h aromatic hydrocarbon possessing seven fused rings, specifically, three five-membered and four six-membered rings, as shown in Fig. 1. Nowadays, this name also refers to its heteroanalogues. Most (hetero)truxenes are cyclotrimerization products of commercially available ketones and oxindoles. To obtain mono- and diheterotruxenes, 6π-electrocyclization is required. The latter requires the synthesis of specific substrates because they are not commercially available. Truxenes has attracted vast interest, similar to other polycyclic aromatics, in the last few years due to the growing demand for organic emitters and semiconductors. Accordingly, scientific efforts are focused on the photostability of materials, purity of their colours, and increasing their charge carrier mobility. Easy processing is also an essential factor. This trend includes truxenes, as evidenced by numerous review papers.1–5 The typical features of truxenes is their significant thermal and photostability. Truxenes and their heteroanalogues can be utilized in OLEDs,6–9 OFETs,10–12 DSSCs,13–15 NLO,16–19 sensors,20,21 gases and small molecule storage22–24 materials, and many more. The enormous diversity of truxenes is due to the unprecedented possibilities for their functionalization. Their modification can be realized on two levels. The first level is individual molecule derivatization, and the second level is building structured materials from the bottom up. In this manner, materials with designed physicochemical properties and structures can be obtained. Furthermore, heteroatom displacement offers the unique possibility of electronic structure tuning.
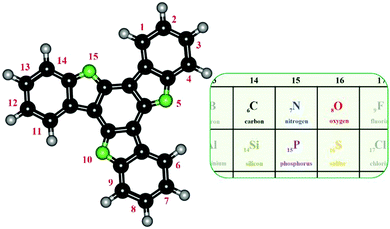 |
| Fig. 1 Possible structures of (hetero)truxenes. | |
One example is 5,10,15-triazatruxene derivatives, which are characterized by a high-lying HOMO of −5.1 eV and exhibit good hole mobility of 8.9 × 10−4 cm2 V−1 s−1.25Fig. 1 presents the various configurations of heteroatom(s) insertion. Truxenes, due to their specific structure, can be polyradicals.26 They are utilized to synthesize strained aromatic moieties,27 including fullerenes,28 chiral molecules,29,30 and supramolecular units.31,32 The main goal of this review is to present the structure-electronic relationship of truxenes. This information can be beneficial for the appropriate design of truxene-like structures. In the present review, truxenes are systematized in terms of their symmetry (presence or absence of a triple-axis of symmetry). Then, in each of these groups, the different heteroatoms are distinguished.
2.
C
3h-Symmetric truxenes
2.1. Truxene: synthesis and reactivity
Truxene 4, for the first time, was accidentally obtained in 1889 by Hausmann during the synthesis of indan-1-one 2 from 3-phenylpropanoic acid 1 (Scheme 1). The mechanism of this reaction, with the participation of the α,β-unsaturated intermediate 3, was elucidated many years later.33 This cyclotrimerization of aldol is still the most frequently used method for the synthesis of truxenes.
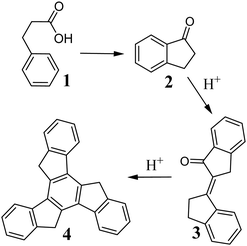 |
| Scheme 1 First truxene synthesis. | |
H+ is necessary to initiate condensation, and hence 4 is formed in strong acidic media, e.g., polyphosphoric acid34 or mixture of conc. AcOH and aqueous HCl.35 The reaction yield of the latter is a record-breaking 98%. Truxenes are also obtained from appropriately functionalized indan-1-ones to synthesize, for example, hexamethoxy derivatives.36 Other truxene derivatives were obtained under harsher conditions but with a lower yield (Scheme 2).37 This can be explained by the negligible solubility of the corresponding α,β-unsaturated ketone.
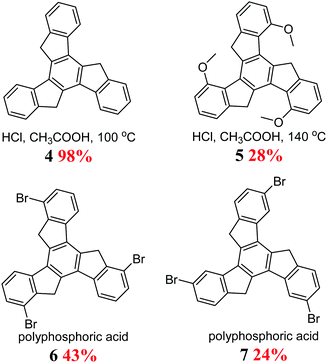 |
| Scheme 2 Indan-1-one cyclotrimerization products, conditions and reaction yields. | |
The described methods require a large excess of acid relative to ketone, but this is not necessary for efficient cyclotrimerization. The use of 3.5 equiv. of p-TsOH and propionic acid in 1,2-dichlorobenzene at 105 °C allows the efficient condensation (59–89%) of indan-1-ones to the corresponding truxenes.38 Further, using 1 equiv. of MeSO3H with Si(OEt)4 in DCM at room temperature allows the cyclotrimerization of 2 with 80% yield.39 Indan-1-ones also undergo condensation in the presence of a catalytic amount of Rh(III) complex at 150–200 °C without solvent.40 The cyclotrimerization of more complexed indanones in the presence of POCl3 is worth mentioning.41 Isomeric compounds 2 and 8 undergo cyclotrimerization to 4 in the presence of SiCl4 in EtOH (Scheme 3).42 However, the broader use of indan-2-ones for the synthesis of truxenes is limited, mainly due to their availability.
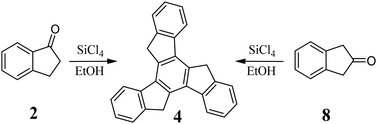 |
| Scheme 3 Cyclotrimerization in the presence of SiCl4/EtOH. | |
The described cyclotrimerization processes allow truxenes that are unfunctionalized in positions 5, 10, and 15 to be obtained. These positions are important for modifying the electronic structure and improving the solubility of truxenes. Indan-1,3-dione 9 in the presence of concentrated H2SO4 forms truxene-5,10,15-trione 11 (Scheme 4).35 Microwave-assisted cyclotrimerization significantly reduces the reaction time while maintaining a high yield of 77%.43 Likewise, 2,2-dibromoindan-1-one 10, under pyrolysis condition, undergoes cyclotrimerization to form 11.44 The main advantage of using 9 and 10 is the direct synthesis of functionalized truxene in positions 5, 10, and 15. The carbonyl groups in 11 undergo ketone-specific reactions, and hence N2H4 can convert it to 4.45 Reverse conversion, i.e., oxidation of the methylene moieties of 4 in positions 5, 10, and 15, is also possible. The use of a composite of KOH and graphene46 or a suspension of KOH in THF47 in the presence of O2 efficiently oxidizes 4 to 11. The base used in this process must be strong enough to ionize the 5, 10, and 15 positions; therefore, KOH can be replaced by Me4NOH or K2CO3.48 Recent studies indicate that in the presence of light and O2, 4 can be oxidized to a monocarbonyl derivative, namely truxene-5-on.49
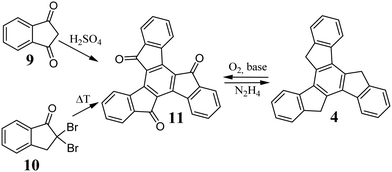 |
| Scheme 4 Synthesis of truxene-5,10,15-trione. | |
Unsubstituted truxenes display low solubility, which is a direct consequence of their strong π-stacking interactions. Thus, to reduce this intermolecular effect, alkyl substituents are introduced in the 5, 10, and 15 positions. Strong bases can ionize the acidic hydrogen atoms in a five-membered ring. The produced aromatic anions 43− and 123− can serve as valuable substrates for further derivatization of the truxene core, for example, C-alkylation (Scheme 5).
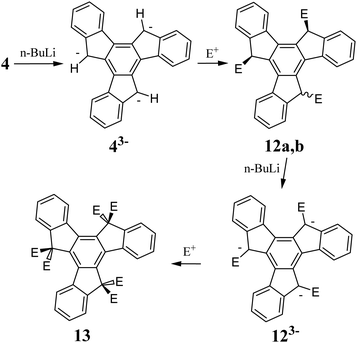 |
| Scheme 5 Triple lithiation of 4. | |
The alkali metal hydrides NaH50 or KH51 and organolithium compounds are the most commonly used bases to generate 43−. In the reaction between 4 and n-BuLi, the orange fluorescent 43− is formed. Subsequent reaction of 43− with, e.g., alkyl halide, results in a mixture of two diastereomers, containing mainly the anti-product 12a. The syn-product 12b can be obtained from 12a after reacting with t-BuOK in t-BuOH.52 In the case that the solubility of the reactants appears to be insufficient, DCM is added to increase their solubility and the isomerization yield. A mixture of 12a and b can be ionized and converted to the corresponding hexa-substituted truxenes 13. Alkyl or benzyl halides are the most commonly used electrophilic agents. However, by using appropriate dibromoalkanes, spiro systems 15 can be obtained (Scheme 6).53
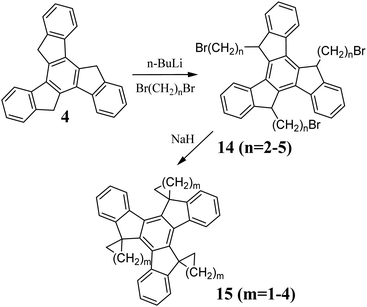 |
| Scheme 6 Synthesis of cycloalkanospirotruxenes. | |
The isolation of products can be troublesome due to their similar polarity after alkylation is performed. Thus, for practical reasons, truxenes are often subjected to exhaustive alkylation to 13 under anaerobic conditions to avoid oxidation.
Complementary modifications can be realized via the carbonyl groups in 11. As a result of the reaction with both organolithium54 and organomagnesium37 compounds, the corresponding trihydroxy derivatives are formed (Scheme 7).
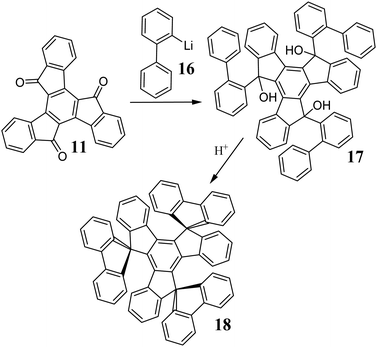 |
| Scheme 7 Synthesis of fluorenespirotruxenes. | |
11 undergoes condensation with malononitrile,55 Wittig-Horner olefination,56 and reacts with organometallic compounds such as PhLi, and more complex derivatives of biphenyl 16,57 anthracene or phenanthrene.58 The use of 16 allows the synthesis of more exotic systems, i.e., trispirotruxenes 18. It is worth noting that trispirotruxenes possessing an external alkyl ring can be obtained via ring-closing metathesis.59
The main limitation of the described methods is the low solubility of 11. Therefore, some modifications may prove challenging to implement. An alternative approach to introduce aryl substituents in the truxene five-membered rings is the addition of an organometallic compound to ester 19 (Scheme 8). The obtained alcohol 20, in the presence of an acid, undergoes cyclization to hexaaryltruxene 21.6020 may also participate in competitive reactions. Cyclization using HClaq may result in the formation of chlorinated by-products; thus, H2SO4 or MeSO3H is suitable. In contrast to 11, compounds 19 and 20 are relatively soluble, making this method attractive for their further functionalization. Truxenes can be functionalized via electrophilic substitution at positions 2, 7, and 12 (Scheme 9). In the presence of Ac2O and AlCl3, 22 undergoes Friedel–Crafts reaction to form 23,61 which proved to be a valuable substrate in the synthesis of dendrimers.62 Using excess AcCl and AlCl3, 24 is obtained.63 Truxene also undergoes other electrophilic substitutions, i.e., nitration using HNO3 in 1,2-dichloroethane. Mono- 25, di- 26, and trinitro derivatives 27 can be obtained in good yield.64 Alternatively, the reduction of NO2 to NH2 allows the further peripheral expansion of the π-electron system.65,66
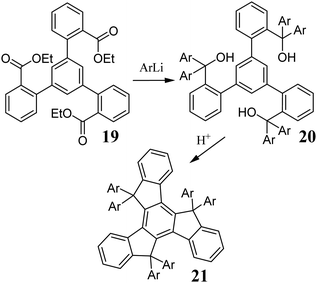 |
| Scheme 8 Synthesis of hexaaryltruxenes. | |
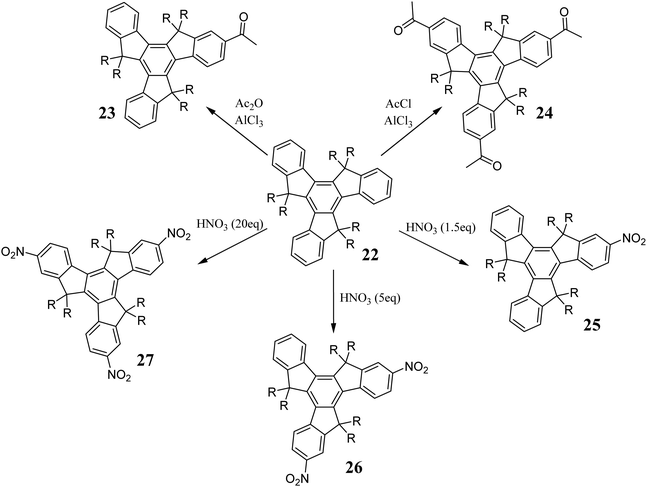 |
| Scheme 9 Electrophilic substitution of truxene. | |
Halogenation is the most common method used for the functionalization of truxenes (Scheme 10). In the presence of N-bromosuccinimide (NBS), 22 undergoes monobromination 28a,67 while using the right stoichiometry of Br2, di- 29a68 and tribromoderivatives 30a can be obtained.69 Six-fold bromination occurs in the presence of a catalytic amount of I231a.70 Iodination is also possible; however, due to the lower reactivity of I2, the presence of an oxidant is required. Mono-iodination product 28b is obtained using a mixture I2/H5IO671 or I2/HIO3.72 However, excess I2/HIO3 at 80 °C allows the introduction of two 29b or three 30b iodine atoms. By increasing the iodination temperature to 115 °C, hexaiodotruxene 31b is obtained. 31b is also a product of the reaction between 22 and I2 in the presence of PIFA.73 Obtaining 28 and 29 is achievable by using the appropriate stoichiometry; however, isolating the desired compound can be troublesome. It is interesting that monosubstituted truxenes possessing electron-withdrawing groups such as formyl undergo efficient double halogenation.74,75
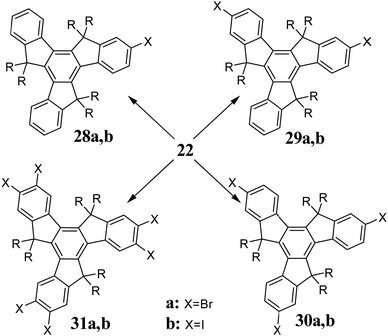 |
| Scheme 10 Halogenation of truxenes. | |
Halogenated truxenes undergo lithium-halogen exchange, which enables the introduction of a carboxyl76 or formyl77,78 group or their conversion to appropriate boronic acids.79 Halogen atom replacement with a boronic ester was also carried out catalytically using Pd(dppf)2Cl2.80 Truxenes also undergo direct triple borylation in the presence of [Ir(OCH3)(COD)]2.81 Under Ulman82 or Buchwald-Hartwig83 reaction, halogenotruxenes are converted into the corresponding 3° amines. However, the most crucial halogenotruxene transformation is Pd-catalysed coupling. The peripheral functionalization via Suzuki reaction allows easy π-electron expansion of the truxene core.84 Halogenotruxenes react with simple boronic acids85–88 and π-expanded derivatives.89–96 The connection of benzoic acid and benzaldehyde is also possible via this process.97 The mentioned Suzuki transformation allows the synthesis of yellow98 and white99 polymeric emitters, as well as the creation of ordered porous materials, namely, two-dimensional COFs (covalent organic frameworks) 32100 and three-dimensional MOFs (metal–organic frameworks) 33101 (Scheme 11). The microwave-assisted coupling of halogenotruxenes with boronic acids allows the introduction of up to six oligofluorene moieties with high reaction yield.102 The Suzuki reaction allows the direct connection of truxene subunits, leading to truxene-only dendrimers103 and polymers.104 An alternative coupling often used to expand the π-electron truxene system is the Sonogashira reaction.105–110 Other Pd-catalyzed couplings, such as the Heck67 and Negishi111 reactions, are occasionally used for the functionalization of truxenes.
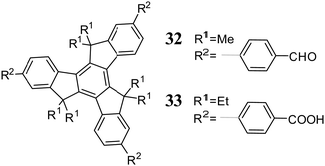 |
| Scheme 11 Structural subunits 32 – COF and 33 – MOF. | |
Truxenes decorated with chelating subunits were used to synthesize multi-core Os2+, Ru2+, and Pt2+ complexes.17,112–116 Some of these systems exhibit non-linear optical (NLO) properties. An interesting example of a truxene-based ligand is the 5,10,15-tri(pyrid-3-ylmethyl) derivative, which coordinates the Re+, placing it on the C3 axis (Fig. 2).117
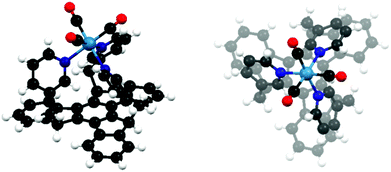 |
| Fig. 2 Structure of carbonyl Re(I) complex. | |
The π-expansion of truxenes can lead to uncommon strained systems such as monkey saddle27 or fullerene fragments, and precursors of C6028,118–120 and C84120,121 (Fig. 3). Several research groups successfully synthesized spherical molecules. Appropriately functionalized truxenes have been proposed as precursors for the synthesis of short carbon nanotubes.122
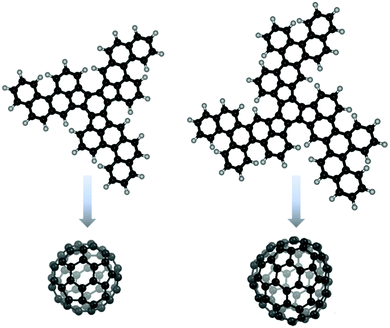 |
| Fig. 3 Truxene-based fullerenes precursors: left – C60 and right – C84. | |
2.2. Truxenes: physicochemical properties and applications
The C3h symmetry of the truxene molecule has an immense impact on its photophysical properties. The HOMO and LUMO levels of truxene are double degenerate. This means that the first two electronic transitions, i.e., S0 → S1 and S0 → S2, are symmetry forbidden. The fluorescence quantum yield of simple C3-symmetric truxenes is relatively low at about 9%. Truxenes also possess a very unusual phosphorescence spectrum with one dominant narrow peak, the origin of which was explained by Baunsgaard et al.123 The T1 → S0 transition is forbidden due to multiplicity and symmetry, resulting in a very long phosphorescence lifetime of about 16 s.124 The truxene moiety exhibits a reversible oxidation process and irreversible reduction.125 In contrast, in its derivatives, both redox processes are reversible,47,55,126 which is beneficial for optoelectronics.
Truxenes having long alkyl chains were the first derivatives thoroughly investigated. They exhibit liquid crystalline properties and rich polymorphism.127–132 Due to their strong π-stacking interactions, truxenes exhibit a tendency to undergo columnar ordering in the solid state. The aggregation combined with the reversible reduction process of truxene-5,10,15-trione makes it promising for the synthesis of new n-type semiconductors.133 Recent studies also suggest the possibility of using truxenes decorated with long alkoxy chains in NLO (Scheme 12).134
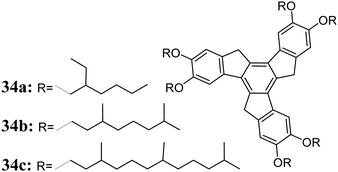 |
| Scheme 12 NLO liquid crystalline truxenes. | |
Truxene 35 has been the subject of many application studies (Scheme 13).135–139 Regardless of the substituent type, 35 has very high fluorescence quantum yields up to 99%.140 The π-electron system expansion upon oligoaryl chain elongation is visible by the redshift in its absorption and emission spectra.141,14235 is thermally and electrochemically stable,143 even exhibiting chemiluminescence.144 These properties make 35 and its derivatives valuable compounds for LEDs,145 OPVs,146 and OFETs.11 They also act as good emitters147 and light-harvesting dyes.148,149
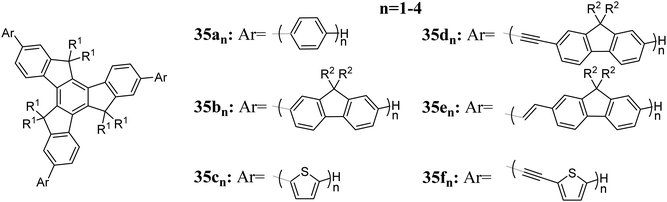 |
| Scheme 13 Oligoaryl truxenes. | |
35b has also been tested for amplified spontaneous emission (ASE).150,151 Similar truxene structures substituted by oligofluorenes152 and oligofluorenes terminated with pyrenes153 or diphenylamines154,155 were tested in a blue laser. The non-linear properties of truxenes 35b and e reveal significant two-photon absorption (TPA).156,157 Theoretical studies suggest that 35c should also exhibit similar properties.158 Besides optoelectronic applications, truxenes,159 truxenes modified by thienyl chains,160,161 and dendrimeric structures made of them,162 have been used as stationary phases in gas chromatography.
The presence of aromatic subunits enables tuning of the physicochemical properties of the π-electron system.163 The introduction of donating subunits increases the electron density significantly in the truxene core, increasing its hole conductivity (Scheme 14).164–171 Its conducting properties can also be tuned differently. The presence of peripheral long alkoxy chains increases the intermolecular interactions, changing the conductivity of substituted truxenes, resulting in hole172 or ambipolar173 conductivity. Donor subunits result in a red shift in the absorption and fluorescence spectra of truxene derivatives.174 Consequently, they can be used as blue emitters in OLEDs6 and lasers.175 Recent research indicates that the presence of the truxene core significantly impacts the light-harvesting properties of materials.15
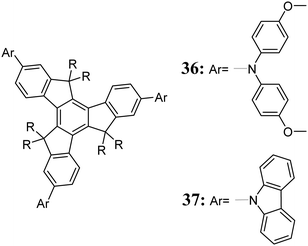 |
| Scheme 14 Examples of electron-rich truxenes. | |
Alternatively, an acceptor unit, such as fullerene,176 attached to truxene, increases its electron conductivity. Current photovoltaic electron-deficient systems do not contain fullerene molecules.126,133,177–182 However, better electron conductivity is not the only advantage of electron-withdrawing substituents. π-Electron systems equipped with diarylboron substituents 39 (Scheme 15) are characterized by more than 4-fold higher fluorescence quantum yield compared to that with 4.183
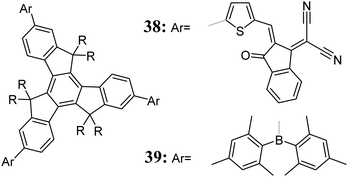 |
| Scheme 15 Examples of electron-deficient truxenes. | |
The donor properties of dithiole-modified truxene and its characteristic concave shape can be used in noncovalent interaction with fullerene C60, which is known for its accepting behavior. This noncovalent donor–acceptor pair is presented in Fig. 4.
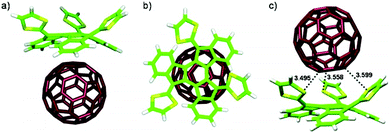 |
| Fig. 4 Structures of the 3 a·C60 complex calculated at the MPWB1K/6-31G** level. (a) Side view of the preferred configuration. (b) Top view of the same configuration, showing the stack between the central benzene ring of the truxene core and one of the hexagonal rings of C60. (c) Side view of the other possible configuration, with C60 approaching 3 a on the dithiole side. S–C short contacts [Å] are also shown. The carbon atoms of the fullerene are depicted in red for clarity. Reproduced from E. M. Pérez, M. Sierra, L. Sánchez, R. Torres, R. Viruela, P. M. Viruela, E. Ortí, N. Martín, Angew. Chem., Int. Ed., 2007, 46, 1847–1851, with permission from John Wiley and Sons. | |
Despite the already discussed donor or acceptor substituents for truxene, it is worth paying more attention to covalent D–A systems.184–188 The common feature of these systems is solvatochromism, resulting from excited-state charge separation.189 The presence of D–A subunits in OPVs inhibits charge recombination.190 This feature makes D–A truxenes applicable in dye-sensitized solar cells (DSSC).191,192
Due to the high affinity of F− to the boron atom, some truxenes can act as fluorescent switches.193 As the concentration of F− increases, the CT state fluorescence intensity decreases in favour of local emission, changing the colour of the emitted light. Besides, some truxenes can detect cations,194,195 nitro compounds,196,197 and even proteins containing metalloporphyrin subunits.198 Recent studies showed that their sensing property is directly related to the presence of the truxene core and the substitution position.199
Truxene-based MOFs and COFs can be used for the storage of gases or small molecules,22 as well as for HCl sensing.20 Surprisingly, an adequately designed structure may lead to electron conductive materials200 or water desalination membranes.201 Some truxene-based polymers show interesting sorption properties, e.g., for CH4.202 Another truxene-based polymeric material exhibited photocatalytic properties203 and could be applied as solar fuels204,205 or for the homocoupling of amines.206 An interesting work on the properties of various truxene-based COFs and MOFs was presented by Berta Gómez-Lor and colleagues, as shown in Fig. 5.207
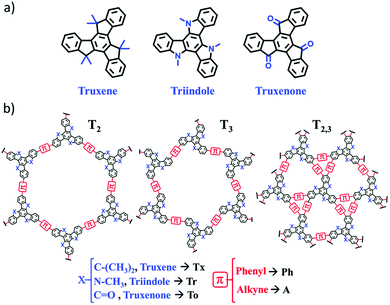 |
| Fig. 5 General chemical structures of the conjugated cores (a) and 2D polymers (b) examined in this study with the notation used throughout the text. Note that the T2 and T3 polymers refer to tri-substituted materials with different linkage positions (2, 7, and 12 in T2 and 3, 8, and 13 in T3), while T2,3 corresponds to hexa-substituted materials in the 2, 3, 7, 8, 12 and 13 positions. Reproduced from S. Gámez-Valenzuela, M. Echeverri, B. Gómez-Lor, J. I. Martínez, M. C. Ruiz Delgado, J. Mater. Chem. C, 2020, 8, 15416–15425, with permission from The Royal Society of Chemistry. | |
Every truxene monomer can be applied to create 2D or 3D materials in multiple ways, where DFT calculations can enable the preselection of suitable monomers. It is worth mentioning that truxene derivatives can be used for the amplification of chirality in discrete molecular assemblies, where one chiral molecule can control the chirality of the entire assembly.78
2.3. 5,10,15-Trisilatruxene
The preparation of heavier analogues of truxenes has been unattainable for years. However, the silole ring formation development, including the sila-Friedel–Crafts reaction208 and catalytic silylation,209 enabled real attempts for the synthesis of 5,10,15-trisilatruxene. In 2017, Ogaki et al. synthesized an alkyl derivative of 5,10,15-trisilatruxene 41 (Scheme 16).124
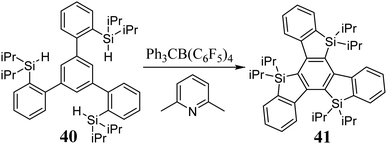 |
| Scheme 16 Synthesis of 5,10,15-trisilatruxene. | |
The three silicon atoms present in the truxene core do not change the symmetry of the system, and hence the first two-electron transitions, as in truxene, are forbidden. The energy of the HOMO is slightly lower, while that of the LUMO is significantly lower compared to truxene. This is due to the σ*–π* interaction in the silole ring. Consequently, a redshift in the absorption and emission spectra is observed.
Generally, the presence of silicon atoms does not affect the fluorescence quantum yield but visibly changes the phosphorescent properties. Two intense peaks are present in the spectrum and the phosphorescence lifetime is four-times shorter, i.e., τphos ≈ 4 s, than in truxene. Due to the relatively high energy of its T1 state, 41 can be used as a sensitizer for blue phosphorescent OLEDs. In comparison to their carbon analogues, siloles have better electron conductivity,210 and hence 5,10,15-trisilatruxenes can be considered semiconductors.
2.4. 5,10,15-Triazatruxene: synthesis and reactivity
The 5,10,15-triazatruxene 47 was presented for the first time by Bergman et al. concerning the condensation of indole 42 and 2-oxindole 43 (Scheme 17).21147 is a product of a multistep synthesis. It begins from bisindole 44, which is obtained during thermolysis of 2-iodoindole 45 or via the Vilsmeier reaction between 42 and 43. Another Vilsmeier reaction between 43 and 44 gives trimer 46, followed by conversion to 47 by cationic radical cyclization, with a total yield of 30.6%. Using activated Cu bronze at 205 °C allowed the conversion of 45 to 47 with 81% yield.212
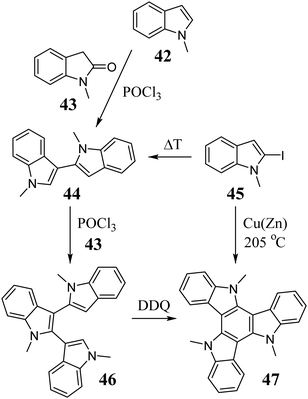 |
| Scheme 17 Synthetic approaches to 47. | |
The electron-rich indoles 48a–e, when treated with (NH4)2S2O8213 or Na2S2O8,214 undergo oxidative cyclotrimerization, leading to isomeric aromatic compounds, i.e., triazatruxene (49a–e) and triazaisotruxene (50a–e) (Scheme 18). Trimerization in the presence of S2O82− is strongly dependent on the solution pH; hence, the best reaction yields (∼30%) are obtained at pH = 1.4. It should also be mentioned that 48f and g under the same reaction conditions lead to triazaisotruxene 50f and g as the only cyclotrimer, respectively. However, due to the large share of byproduct 50 (yield ∼20%), oxidative cyclotrimerization may not be an attractive method for the synthesis of 5,10,15-triazatruxenes.
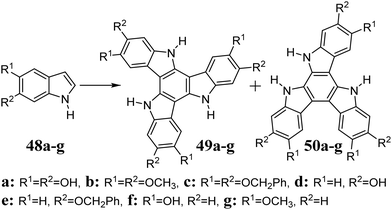 |
| Scheme 18 Oxidative cyclotrimerization of 48. | |
In 2009, Ginnari-Satriani et al. reported the one-step synthesis of 5,10,15-triazatruxene 52 from 2-oxindole 51 (Scheme 19).215 The result of this reaction is highly dependent on both the temperature and the concentration of POCl3. As the concentration of POCl3 increases, the yield of cyclotrimer 52 decreases in favour of cyclotetramer formation 53.216 The discovery of this method initiated further development, which allows the condensation of substituted 2-oxindoles 54.16,217,218
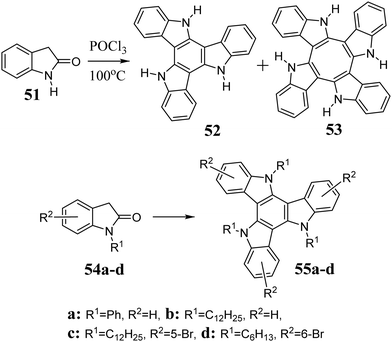 |
| Scheme 19 2-Oxoindole condensation. | |
Indole 56 in the presence of Br2 undergoes cyclotrimerization to 5,10,15-triazatruxene. The triazatruxene core is highly reactive towards electrophilic substitution and with excess Br2, leads to the hexabromo derivative 57.219 After the debromination of 57 in the presence of Pd/C and HCOOHNEt3, 58 can be obtained (Scheme 20).220 Alternatively, some deactivated indoles can be synthesized via cyclotrimerization in AcOH in the presence of Br2.221
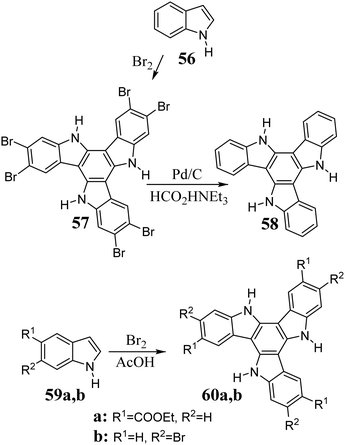 |
| Scheme 20 Bromine-promoted indole cyclotrimerization. | |
As a result of the reaction between indole and NBS, polybrominated 5,10,15-triazatruxenes are obtained. After debromination, they lead to functionalized derivatives 61, 62 and 63 (Scheme 21).222
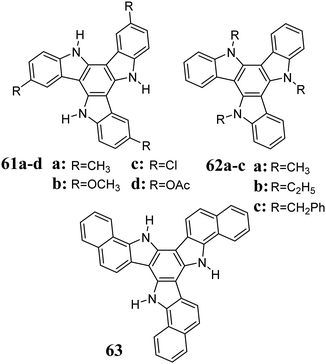 |
| Scheme 21 Indole condensation products. | |
5,10,15-Triazatruxene is very reactive toward electrophilic substitution. The most reactive positions are located opposite the nitrogen atom (3, 8, and 13 – green dots, Fig. 6). Alternatively, in the presence of excess electrophile, the substitution may proceed further at positions 2, 7 and 12 (blue dots).
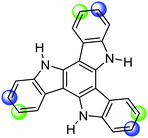 |
| Fig. 6 Positions susceptible to electrophilic attack in 5,10,15-triazatruxene. | |
5,10,15-Triazatruxene, in the presence of TfOH, reacts with 3° aromatic alcohols via triple electrophilic substitution.223 Upon treatment with SnCl4/CHCl2OCH3, 5,10,15-triazatruxene undergoes formylation, leading to trialdehyde 65.18 The formyl group was used for further functionalization, enabling the synthesis of D–A system 66 and BODIPY and 5,10,15-triazatruxene hybrids 67 (Scheme 22).224
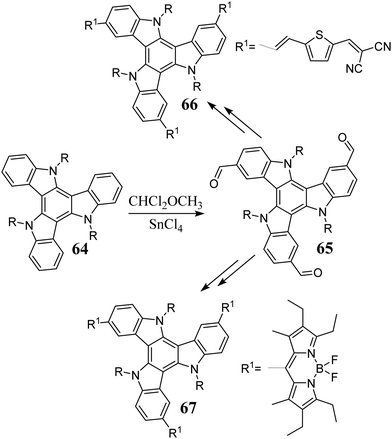 |
| Scheme 22 Synthesis of 5,10,15-triazatruxene 65 and its reactivity. | |
Bromination is the primary electrophilic substitution reaction to which 5,10,15-triazatruxene is subjected. Three factors including appropriate stoichiometry, NBS as the brominating agent, and a lower temperature are relevant to obtain greater control over the final composition of the reaction mixture. Accordingly, the appropriate mono- 68,217 di- 69,225 or tribromo derivative 70226 can be obtained. 68 is also a product of the partial debromination of 70 in the presence of HCOONa and Pd (Scheme 23).
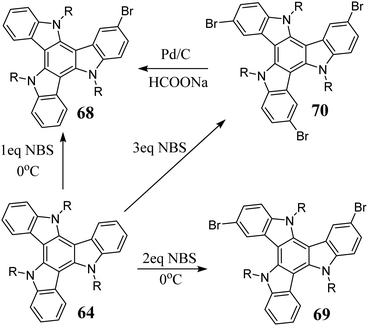 |
| Scheme 23 Bromination of 5,10,15-triazatruxene. | |
As a result of the coupling between bromotriazatruxenes 71 and boronic acids, π-electron-expanded, efficient blue emitters were obtained (Scheme 24).227–231 The Suzuki reaction can be performed in the presence of microwave radiation.22671 also undergo catalytic halogen to boron exchange.232
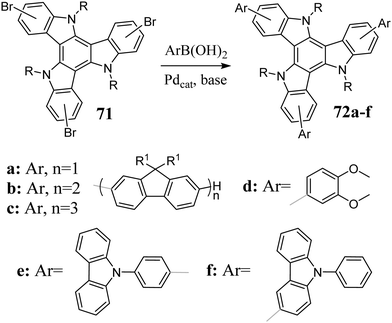 |
| Scheme 24 5,10,15-Triazatruxenes obtained by the Suzuki reaction. | |
Suzuki coupling allows the synthesis of robust 5,10,15-triazatruxenes.233 However, desirable products for optoelectronics can be obtained via alternative methods such as the Sonogashira reaction234,235 and microwave-assisted Stille coupling (Scheme 25).236 It should be mentioned that 5,10,15-triazatruxenes unprotected at the nitrogen atom undergo the Buchwald-Hartwig reaction.237,238
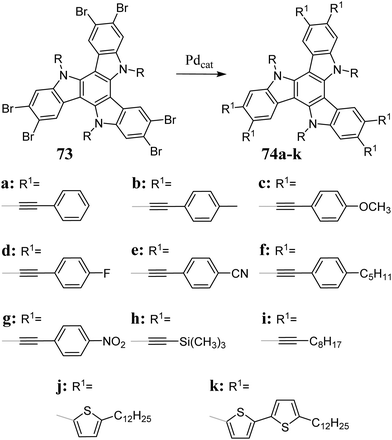 |
| Scheme 25 Sonogashira and Stille coupling products. | |
A significant reaction that 58 undergoes is N-alkylation under basic conditions. This crucial transformation in 5,10,15-triazatruxene chemistry allows protection of reactive positions and increases the product solubility. Strong bases such as KOH,239 NaH,234t-BuOK,13 and n-BuLi235 are used as ionizing agents (Scheme 26). Anion 75, formed during ionization, is extremely easily oxidized, accompanied by a change in solution color to sapphire. Therefore, its deprotonation is performed under strict anaerobic conditions.
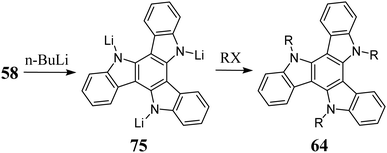 |
| Scheme 26
N-Alkylation of 5,10,15-triazatruxene. | |
Various types of chlorides, bromides, and iodides can serve as alkylating reagents.240 Benzyl moieties are attractive for protecting nitrogen atoms because they can be easily removed afterward.234 It is also worth mentioning that the use of an appropriate alkylating agent increases the product solubility in organic solvents and water.241
An exciting example of 58N-alkylation is its reaction with benzyl bromide 76, resulting in cyclophane 77. Further reaction with PIFA leads to the stable cation radical 78, located at two π-stacking triazatruxene subunits (Scheme 27).
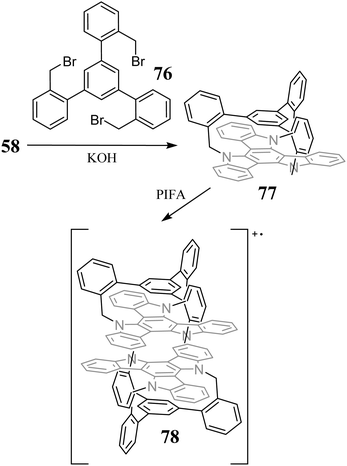 |
| Scheme 27 Synthesis of cyclophane and stable cation radical. | |
N-Alkylation was used to expand the aromatic system. Compounds 79, due to Pd-catalyzed intramolecular arylation, are transformed into π-expanded systems 80 (Scheme 28).220 It is worth mentioning that 80 adsorbed on the surface of Pt at 750 °C undergoes cyclodehydrogenation, leading to the formation of the spherical molecule C57N3, triazafullerene.242
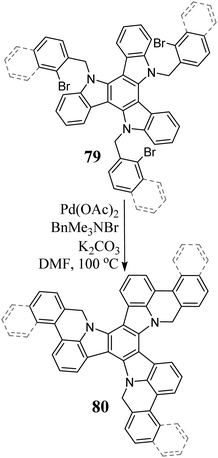 |
| Scheme 28 Synthesis of π-expanded 5,10,15-triazatruxenes. | |
2.5. 5,10,15-Triazatruxene: physicochemical properties and applications
In 5,10,15-triazatruxene, the same as in truxene, the first two-electron transitions are symmetry forbidden, reflecting a low fluorescence quantum yield (QY = 3%).243 A reduction in its HOMO–LUMO gap causes a clear redshift in its absorption and emission spectra compared to truxene. The relatively high energy of its HOMO level is responsible its reversible oxidation, which combined a with its significant tendency to aggregate, leads to high hole conductivity, characteristic for this type of system. Alternatively, the phosphorescence spectrum indicates that the T1 state of 58 is much more populated, in contrast to 4. An efficient S1 → T1 intersystem transition is responsible for the low fluorescence quantum yield.
N-Alkylation of 58 slightly affects its optoelectronic properties in the solution but causes evident structural changes in the solid state. The presence of an alkyl chain deforms the 5,10,15-triazatruxene core from planarity,244 increasing its intermolecular distances and limiting effective π-stacking interactions. However, further elongation of the alkyl chain results in columnar ordering. This behaviour results from the increasing interactions between the alkyl chains and π-system.10 An exciting example illustrating the aggregation upon alkyl chain elongation is the increase in photocurrent generation observed in a tri(n-octyl) derivative.245 In turn, introducing a branched alkyl chain increases the solubility but significantly limits the π-stacking interactions, decreasing the hole conductivity.246 However, N-alkylation does not always lead to strong π-stacking interactions. If the system is already peripherally functionalized (81), the opposite effect is observed, where if the alkyl chain is shortened, the hole conductivity increases (Fig. 7).247
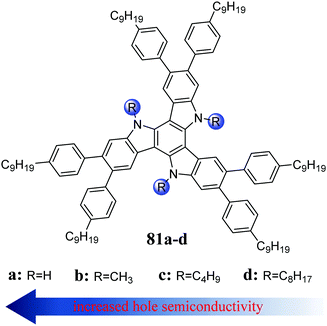 |
| Fig. 7 Increase in conductivity of 5,10,15-triazatruxenes. | |
The self-assembly of 5,10,15-triazatruxenes248 was employed to create optical waveguides249 and design new semiconductor materials for use in prototypical organic thin-film transistors,250 organic field-effect transistors12,251,252 and OPVs.253 In the case of OPVs, 47 or 58 are hole-transporting materials (HTM) and their efficiency increased by 26% compared to the reference devices.253 The desirable properties of 5,10,15-triazatruxenes result directly from their electronic structure. The relatively low energy of their HOMO level allows effective hole conductivity,25 while the high energy of their LUMO level limits electron leakage;254 therefore, they can be used as a cheap HTMs.255,256 The semi-conductivity of 5,10,15-triazatruxenes can be modulated by increasing their degree of aggregation via peripheral functionalization228,235,257–259 or the presence of donor subunits (Scheme 29).260–264 Devices based on functionalized systems can achieve a photocurrent generation efficiency of up to 18.8%.265
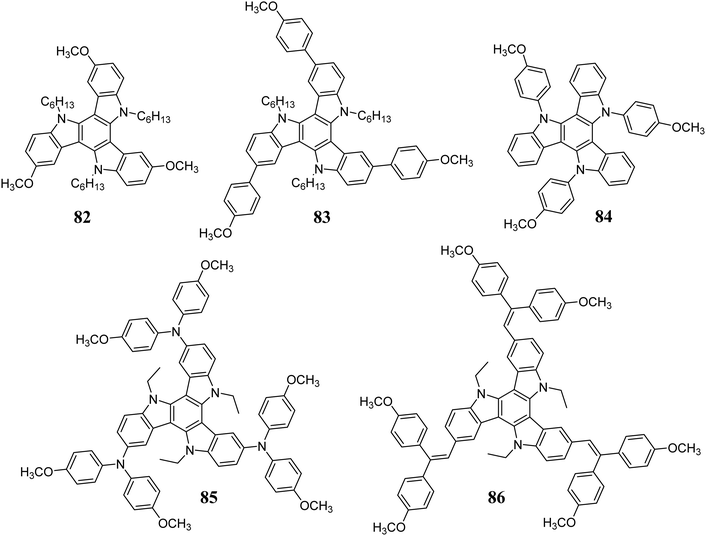 |
| Scheme 29 5,10,15-Triazatruxene-based HTMs. | |
The use of 5,10,15-triazatruxenes in OPVs is not only limited to their semiconductive properties. Some derivatives were used in DSSCs.14 Due to their aggregation and strong intramolecular interactions, it was possible to obtain a high loading of 5,10,15-triazatruxene-based dye at the TiO2 surface, improving the performance of the prototype DSSC device.266 Alternatively, the combination of BODIPY with the 5,10,15-triazatruxene core results in blue dyes with a molar absorption coefficient of 106 M−1 cm−1. These systems can serve as light-harvesting materials.217 The introduction of acceptor subunits13,267–269 increases the electron conductivity, allowing more balanced charge transport inside the DSSC device.
Functionalization of 5,10,15-triazatruxene with oligothiophene subunits results in materials with good hole conductivity.270 Alternatively, oligofluorene 5,10,15-triazatruxenes are characterized by a high fluorescence quantum yield231 and exhibit solvatochromism.226 The introduction of carbazoles has a similar effect on their spectroscopic properties such as oligofluorene and improves their thermal stability.271 A redshift, which is associated with oligoaryl chain elongation, was investigated theoretically by Zhang et al. (Scheme 30).272 They performed calculations on systems with 1 to 4 oligoheterofluorene subunits 87. It was found that regardless of the heteroatom introduced, the HOMO level is mainly located at the 5,10,15-triazatruxene core and its energy practically does not change upon elongation of the substituents. However, extending the oligoaryl chain lowers the LUMO level energy, with a simultaneous shift in the electron density from the triazatruxene core to the oligoaryl chain. The spatial separation of the HOMO and LUMO orbitals explains the solvatochromism observed in the oligofluorene 5,10,15-triazatruxenes.
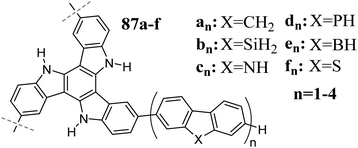 |
| Scheme 30 Tri(oligoheterofluorene) 5,10,15-triazatruxenes. | |
5,10,15-Triazatruxenes are used to create D–A systems, exhibiting thermally activated delayed fluorescence (TADF),7,273,274 which is utilized in new-generation OLEDs. The presence of triazine subunits in systems 88 and 89 (Scheme 31) results in a redshift in their emission, high photoluminescence quantum yield of about 87%, and an energy difference between S1 and T1 equal to 23 meV.275 Green OLEDs containing 88 or 89 in the emissive layer are characterized by an external quantum efficiency of 31.4%. Moreover, a properly designed D–A system can act as the sensitizer in TADF OLEDs.276
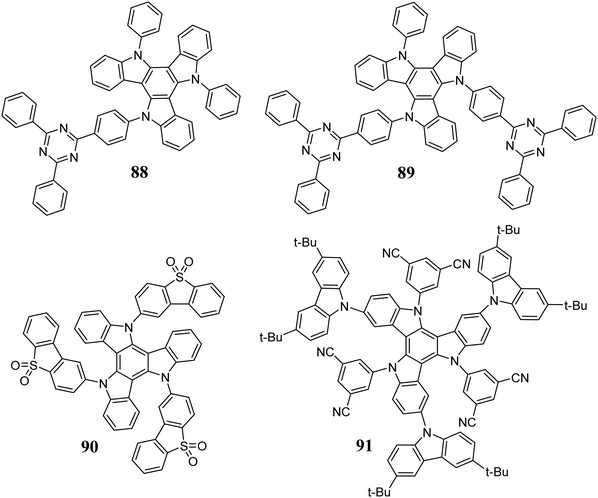 |
| Scheme 31 Triazatruxene-based D–A systems. | |
The dibenzo[b,d]thiophene sulfone-based D–A system 90 exhibits a very short delayed fluorescence decay time.8 This phenomenon is attributed to about 30 different couplings between the S1 and T1 states. Very fast T1 →S1 conversion results in slight energy loss, and this the photoluminescence quantum efficiency is close to 100%. Penfold et al. explained the efficient TADF process in D–A systems based on 90.277 D–A systems can be utilized as TADF emitters or NLO materials.18,19 These compounds show increased TPA, resulting from the spatial separation between the excited and ground states. It was found that the cross-section value for TPA also depends on the position of the acceptor subunit, where 92 exhibits stronger TPA than 93 (Scheme 32).278 It is worth mentioning that the NLO properties of 5,10,15-triazatruxenes have been successfully used in cell imaging.279
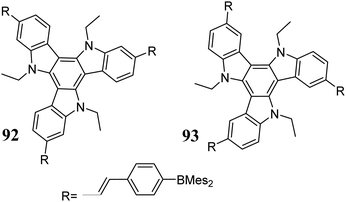 |
| Scheme 32 Isomeric D–A systems exhibiting NLO properties. | |
5,10,15-Triazatruxene was also used to create macromolecular systems such as COFs,280 MOFs,281,282 and polymers.283,284 5,10,15-Triazatruxene-based polymers can be used as capacitor materials.284 Some COFs280 and polymers285 exhibit higher selectivity towards CO2 adsorption compared to N2 or can store H2 (Fig. 8).23 The storage capacity of triazatruxene polymers increases in the presence of Li+ ions. Thus, it is crucial to redistribute Li+ ions homogenously in the material, which is achieved by cation–π interactions.
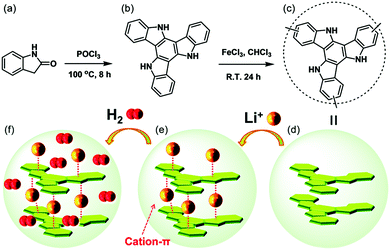 |
| Fig. 8 (a)–(c) Synthetic route for the conjugated microporous polymer PTAT. Schematic representation showing the installation of lithium-doped CMP Li+–PTAT through point-to-face cation–π interactions (d and e) and its hydrogen adsorption (f). Reproduced from L. Yang, Y. Ma, Y. Xu, G. Chang, Chem. Commun., 2019, 55, 11227–11230 with permission from The Royal Society of Chemistry. | |
Some of these substances exhibit fluorescence quenching upon interaction with electron-deficient systems.
This property inspired the synthesis of low molecular weight and polymeric materials for the detection of explosives.286–288 Adequate peripheral functionalization allowed the detection of HCl289 and N2H4 vapors.290 5,10,15-Triazatruxene, due to its semiconductive properties, has been used in the synthesis of new, thermally stable polymeric HTMs.291–293 A 5,10,15-triazatruxene-based microporous polymer could capture heavy metals.294 A similar interaction with Li+ cations resulted in a significant increase in the electrical capacity of the polymeric anode.24 Interactions between 5,10,15-triazatruxene and Fe2+
295 or Zn2+
296 connected with iminopyridyl led to interesting chiral, tetrahedral molecules. Alternatively, the rational design of the molecule may lead to Al3+, Cr3+ and Fe3+ sensors.297 5,10,15-Triazatruxene peripherally decorated with carboxyl groups can be used to modify the surface of TiO2, enhancing the electron injection in DSSCs.298
5,10,15-Triazatruxene modified by mannose21 or lactose299 was used for sensing concanavalin A. 5,10,15-Triazatruxene, upon N-alkylation with alkylpiperidine chains, becomes soluble in water and shows amphiphilic properties.215,300 These substances also have enormous affinity and selectivity toward DNA G-quadruplexes, creating new anti-cancer drugs.301,302
2.6. 5,10,15-Triphosphatruxene
5,10,15-Triphosphatruxene has been synthetically unavailable for years. One of the problems arising during the formation of the phosphole ring is that the known methods are not stereoselective. The phosphorus atom in the phosphole ring, unlike the nitrogen atom in pyrrole, has sp3 hybridization, becoming a potential stereogenic centre. The 5,10,15-triphosphatruxene molecule has three stereogenic phosphorus atoms, but due to its symmetry, the number of possible stereoisomers is reduced to four (Scheme 33).
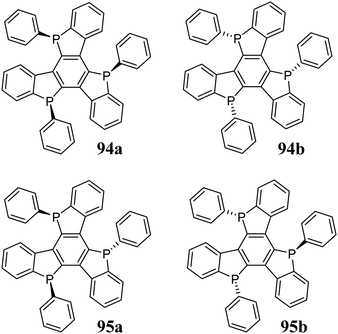 |
| Scheme 33 5,10,15-Triphosphatruxene stereoisomers. | |
In 2014, Kojima et al.303 synthesized this complex system via aromatic nucleophilic substitution to obtain a mixture of syn 94 and anti 95 isomers in a ratio of about 1
:
15. However, due to the similar properties of these diastereomers, their separation was complicated. Therefore, their crude mixture was subjected to subsequent oxidation. This procedure enabled crystallization of the pure oxidized isomer anti 98 (Scheme 34). Alternatively, upon sublimation of a crude mixture of 94 and 95, it underwent complete conversion to the thermodynamically more stable isomer 94. 5,10,15-Triphosphatruxene isomers differ in the symmetry of their molecule. The syn-isomer has C3 symmetry, whereas the anti-isomer C1. Therefore, different spectroscopic properties are expected.
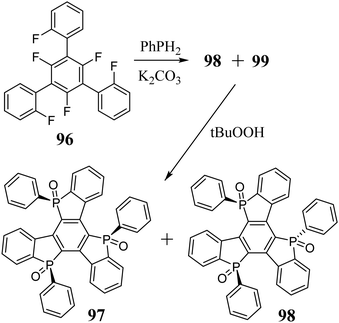 |
| Scheme 34 Synthesis of 5,10,15-triphosphatruxenes. | |
2.7. 5,10,15-Trioxatruxene
The first report on the synthesis of 5,10,15-trioxatruxenes is dated 1984, when Destwde et al. obtained several derivatives of this heterocyclic system.304 Compounds 100 are products of benzo[b]furan-3-one 99 cyclotrimerization in the presence of PPE (Scheme 35). After deprotection of the hydroxyl groups, 5,10,15-trioxatruxene can be further modified to increase its solubility.
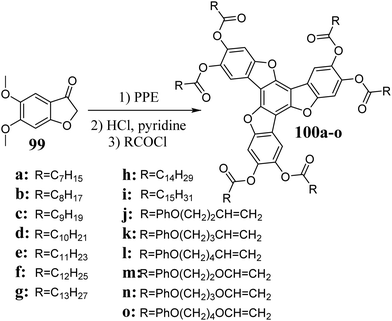 |
| Scheme 35 Synthesis of 5,10,15-trioxatruxene ester derivatives. | |
The 5,10,15-trioxatruxenes 102a and b were synthesized using a similar procedure. To carry out the cyclotrimerization of 101a and b, CF3COOH305 was used instead of PPE, as mentioned above (Scheme 36).
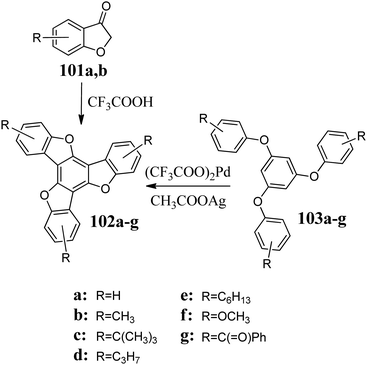 |
| Scheme 36 Synthesis of 5,10,15-trioxatruxenes. | |
The described methods were used to synthesize several 5,10,15-trioxatruxene monomers, 100j–o, containing peripheral polymerizable moieties, making it possible to produce a cross-linked polymer (Scheme 35).306
Sato et al. refined the synthesis of hexahydroxy-5,10,15-trioxatruxene, enabling the isolation of the condensation products and avoiding chromatography. Thus, this method is attractive for industrial applications.307 Sato observed that during deprotection, partial oxidation to quinone occurs. 1,3,5-Triaryloxybenzenes 103a–g, in the presence of (CF3COO)2Pd/CH3COOAg, are converted to 102 in high yield (Scheme 36).308 However, for derivatives containing donor or acceptor groups, the efficiency of oxidative coupling is relatively low. An exciting example of the efficient synthesis of 102a is the use of aromatic nucleophilic substitution.124
Similar to the previously described systems, the first two electronic transitions in 5,10,15-trioxatruxene are symmetry forbidden.124
5,10,15-Trioxatruxene is characterized by a relatively low fluorescence quantum yield of ∼17%. Alternatively, the shape of its phosphorescence spectrum suggests the presence of strong coupling between the T1 and S0 oscillation states. Moreover, the phosphorescence lifetime of about 5.4 s indicates the forbidden nature of the T1 → S0 transition due to the spin multiplicity and the symmetry of the orbitals.124102c was examined towards electron mobility.309 The presence of t-butyl groups limits the intermolecular interactions, increasing the solubility of the system and making the oxidation process reversible.308
Alkylated 5,10,15-trioxatruxenes exhibit liquid-crystal properties and rich polymorphism.304 The self-assembly of 102a and its liquid crystalline derivatives toward columnar ordering, combined with reversible oxidation, allows their use as p-type semiconductors. Substituted 5,10,15-trioxatruxenes, containing double bonds on their peripheral alkyl chains, have been used to synthesize cross-linked polymers,306 while 104 were used as photopolymerization agents, creating so-called “self-healing” LCDs (Scheme 37).310
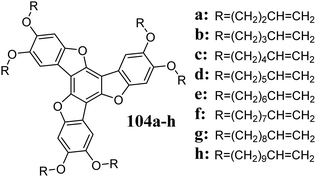 |
| Scheme 37 5,10,15-Trioxatruxene-based photopolymerization monomers. | |
2.8. 5,10,15-Trithiatruxene
Peripherally substituted 5,10,15-trithiatruxenes 106 were described for the first time in 1985 by Nguyen et al.311 Their synthesis was based on the acidic condensation of substituted benzo[b]thiophen-3-one, 105, in the presence of PPE (Scheme 38).
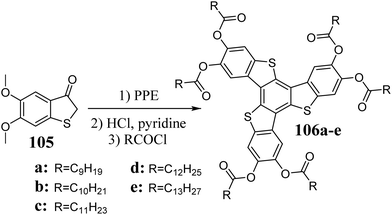 |
| Scheme 38 Synthesis of 5,10,15-trithiatruxene ester derivatives. | |
Egestad et al. showed that the treatment of benzo[b]thiophen-3-one with HCl in AcOH leads to a complex mixture of dimeric, trimeric, and tetrameric systems.312 The same research group performed the cyclotrimerization of 3-acetoxybenzo[b]thiophene in CF3COOH. They obtained 5,10,15-trithiatruxene in a yield of 13%.305 5,10,15-Trithiatruxenes are cyclization products of appropriate thioethers in the presence of (CF3COO)2Pd/CH3COOAg at elevated temperatures. Thus, isomeric t-butyl peripherally functionalized 5,10,15-trithiatruxenes were obtained. Unfortunately, the reaction yields were much lower than that observed in the case of their oxygen counterparts.309
Similar to other symmetrical systems, 5,10,15-trithiatruxene reveals the first two electronic transitions forbidden by symmetry. The presence of sulfur atoms increases the intersystem crossing, resulting in phosphorescence in the solid state at room temperature.309 Miura et al. measured the phosphorescence spectra of two tert-butylated trithiatruxenes. The results are eye-catching. The phosphorescence of trithiatruxenes in PMMA films was only observed under vacuum. The authors measured the phosphorescence at low temperatures in methylcyclohexane. They observed almost pure phosphorescence (with minimal fluorescence signal) under these conditions for trithiatruxenes.
Hexa-substituted 5,10,15-trithiatruxenes, like their oxygen analogs, are characterized by rich polymorphism and columnar ordering in the solid state.311,313 This property has been exploited in epoxy resins, encapsulation, and electronic and structural laminates.314 Currently, due to the dynamic development of optoelectronics, some 5,10,15-trithiatruxene derivatives can be used in OLEDs,315,316 OFET,317 and OPVs.318 The expanded, liquid-crystalline 5,10,15-trithiatruxene derivatives 107–109 developed by Aida et al. tend to self-assemble, increasing their semiconductive properties and leading to balanced, ambipolar conductivity of 0.18 cm2 V−1 s−1 (Scheme 39).319
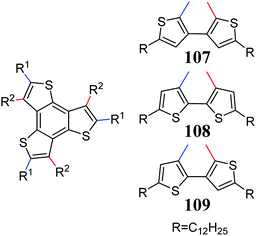 |
| Scheme 39 5,10,15-Trithiatruxene-based ambipolar semiconductors. | |
2.9. Borazatruxene
Truxenes in which the central benzene ring is replaced by borazine are structurally interesting systems, formally leading to hexaheterotruxenes. In 2019, Limberti et al. described a three-step synthesis in which 110 is converted to the corresponding borazatruxenes 111 in the presence of microwave radiation at 180 °C (Scheme 40).320 The substrates enabling the synthesis of 110 are the appropriate nitriles or aldehydes. Their availability significantly increases the variety of borazatruxenes, allowing the synthesis of halogen derivatives and π-expanded systems. It should also be mentioned that the use of appropriate aromatic ketones enables the synthesis of derivatives functionalized at the 5, 10, and 15 positions.
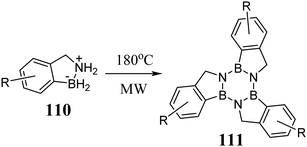 |
| Scheme 40 Borazatruxene synthesis. | |
In the case of 111, a clear blueshift can be observed in its absorption and emission spectra,320 while its fluorescence quantum yield does not exceed 10%. Borazatruxenes are air and moisture stable and exhibit better solubility, especially in moderately polar solvents, than the practically insoluble 4. The flat structure of heterotruxene core and strong intramolecular interactions drive columnar ordering of the molecules in the solid state, creating potential organic semiconductors.
3. Non-symmetrical truxenes
The non-symmetrical truxenes described in this chapter are molecules of lower symmetry (without the triple-axis). Currently, the known non-symmetrical heterotruxenes possess at least one heteroatom. When working with polycyclic condensed aromatic systems, a remarkable problem is their poor solubility. The same issue also applies to truxenes. Truxene and 5,10,15-triazatruxene can be alkylated within 5-membered rings to improve their solubility. However, this procedure cannot be used for systems containing oxygen or sulfur atoms. This obstacle is partially solved by the peripheral functionalization of the π-electron system with alkyl substituents. Nevertheless, this procedure prevents their further modification. Another solution to overcome their low solubility, while maintaining their unique heteroatom properties, is the concept of 5-monohetero and 5,10-diheterotruxenes (Fig. 9).
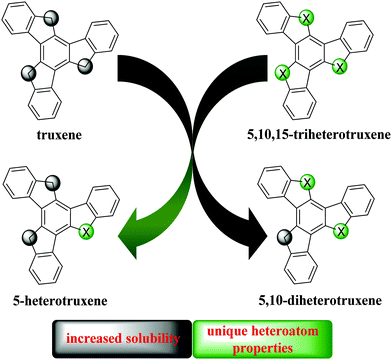 |
| Fig. 9 Mono and diheterotruxenes as hybrids of symmetrical systems. | |
An unquestionable advantage of non-symmetrical truxenes is their solubility, resulting from C-alkylation. It is also worth mentioning that symmetry breaking of a molecule affects its physicochemical properties. Thus, targeted functionalization for mono-substitution is an undisputed advantage in low-symmetrical cores. However, this procedure in the case of C3-symmetric truxenes is a challenging task.
3.1. 5-Oxatruxene
5-Oxatruxene was synthesized by Górski et al. in 2018 during the photocyclization of the corresponding triene 113 (Scheme 41).321 5-Oxatruxene, similar to other unsubstituted truxenes, is characterized by relatively low solubility. However, the alkylation of its cyclopentadienyl rings in a basic environment significantly increases its solubility. For example, the obtained tetraethyl derivative 114 exhibits good solubility in most organic solvents. 5-Oxatruxene undergoes electrophilic substitution and in excess Br2, can be converted to the corresponding tribromo derivative 115.125
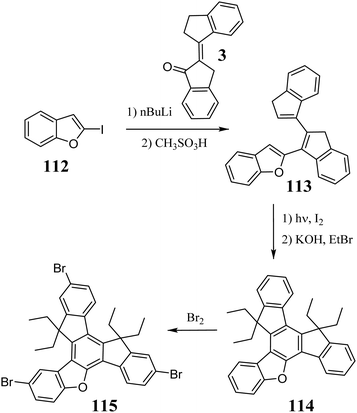 |
| Scheme 41 Synthesis and reactivity of 5-oxatruxene. | |
The presence of an oxygen atom in the molecule causes structural changes, which affect the electronic levels of 5-oxatruxene. Symmetry breaking lifts the HOMO and LUMO degeneration, which is visible in the redshift in its absorption and emission spectra. Moreover, a 4-fold increase in the fluorescence quantum yield is observed.321 Unlike truxene, 5-oxatruxene exhibits better thermal properties, remarkable photostability, and a reversible oxidation process.125 The presence of an oxygen atom leads to π-stacking interactions, which combined with other properties, suggest its use as an HTM.
3.2. 5-Azatruxene
The first synthesis of the soluble 5-azatruxene 118 was described by Górski et al. in 2019.322118 is a product of multistep synthesis, where the last step is the lithiation of 116, followed by BF3-mediated cyclodehydration (Scheme 42). An alternative method based on a solvent-free Cadogan reaction was developed one year later.125 The nitro compound 117 heated with PPh3 is converted to 5-azatruxene, unprotected at the nitrogen atom. The base-promoted N–H bond ionization, followed by reaction with an alkyl halide, finally leads to the alkyl derivative 118. The electron-rich 5-azatruxene core readily undergoes electrophilic substitution. Thus, mono- 119,322 tri- 120, and tetrabromo derivative 121125 can be obtained in good yield.
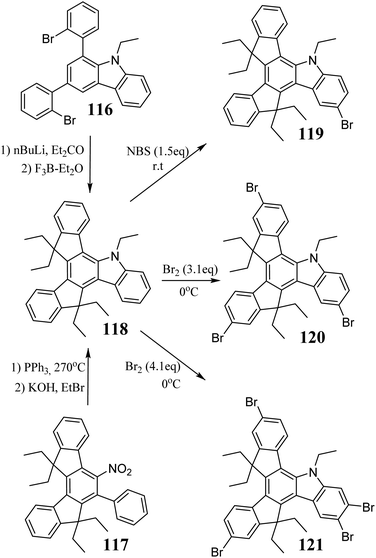 |
| Scheme 42 Synthesis and reactivity of 5-azatruxene. | |
The introduction of a nitrogen atom causes degeneration lifting and significant destabilization of the HOMO level.125 Thus, among the known 5-heterotruxenes, 5-azatruxene has the most redshifted absorption and fluorescence spectra. Compared to 4, 5-azatruxene exhibits a 4-times higher fluorescence quantum yield. Moreover, 5-azatruxene undergoes reversible oxidation, forms stable glasses, and shows the highest photostability among the known 5-heterotruxenes.
3.3. 5-Thiatruxene
In 2017, Maciejczyk et al. published a multistep synthetic protocol for 5-thiatruxene 124 (Scheme 43).323 Two years later, 124 was synthesized via the photocyclization of 123, clearly shortening the synthesis time.322 The various 5-thiatruxene reactivity results not only from the presence of an expanded aromatic system but also from the sulfur atom. The monobromo derivative 125 can be obtained by direct lithiation of 124 and subsequent reaction with 1,2-dibromoethane.323 5-Thiatruxene readily reacts with Br2, leading to both di- 126323 and tribromo 127322 derivatives. Interestingly, both experiments showed that the bromination process occurs first on the peripheral benzene rings away from the heterocyclic system. Due to the presence of a sulfur atom, it is possible to change its oxidation state. As a result of the reaction between 5-thiatruxene and H2O2 or mCPBA, the corresponding sulfoxide 128 or sulfone 129 is formed.322 Subsequently, the SO2 group present in 129 significantly facilitates direct ortho lithiation with TMPLi. Moreover, the generated organometallic derivatives undergo typical reactions with electrophiles, allowing further functionalization of the 5-thiatruxene core. The synthesis of monoiodo derivative 130 was performed using the protocol mentioned.322 Oxidation of the sulfur atom changes the nature of the 5-thiatruxene core from electron-donating to electron-accepting.125 Thus, D–A systems 131–134 can be created with 5-thiatruxene as an electron acceptor (Scheme 44).9
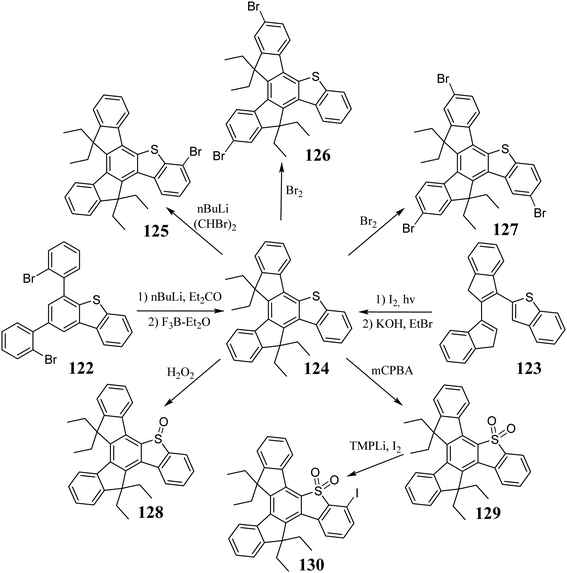 |
| Scheme 43 Synthesis and reactivity of 5-thiatruxene. | |
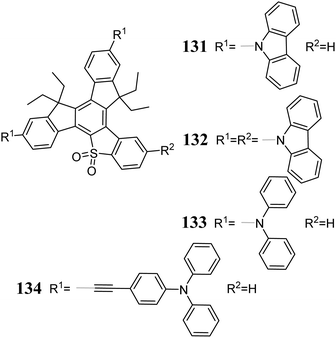 |
| Scheme 44 5-Thiatruxene-based D–A systems. | |
Due to the symmetry breaking in 5-thiatruxene, no degeneration of electron levels is observed.125 Alternatively, the electron-donating properties of the sulfur atom cause noticeable destabilization of its HOMO level compared to 4. Despite the symmetry breaking, a decrease in its fluorescence quantum yield is observed. The factor responsible for this is the presence of a heavy atom, increasing intersystem crossing and T1 population. The oxidation of the sulfur atom leads to sulfone 129, which is much more stable. Moreover, the thermal properties and photostability are improved compared to 4. 5-Thiatruxene exhibits reversible oxidation and irreversible reduction. However, in the case of the sulfone derivative, the opposite behavior is observed. 5-Thiatruxene D–A systems 131–134 were used as blue emissive layers in prototype OLEDs. These substances exhibited very high thermal and photostability, which directly influence the device performance, even up to 10 kCd m−2, at high brightness.9
3.4. 5,10-Dioxatruxene
5,10-Dioxatruxene, like 5-oxatruxene, was not available synthetically for a long time. In 2020, Górski et al. published the multi-step synthesis of unsubstituted 5,10-dioxatruxene, in which the final reaction is the photocyclization of triene 135 (Scheme 45).324 Further, basic C-alkylation leads to a well-soluble derivative 136. Like other truxenes, 5,10-dioxatruxene undergoes bromination to 137.
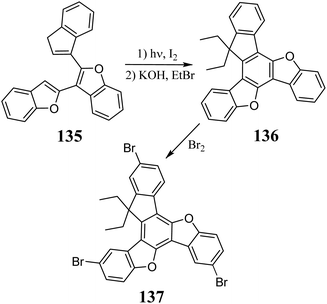 |
| Scheme 45 Synthesis and reactivity of 5,10-dioxatruxene. | |
The introduction of two oxygen atoms in the truxene core leads to molecular symmetry breaking (C3h → Cs); thus, no degeneration in electron structure is observed.324 The described modification leads to slight destabilization of its HOMO and stabilization of its LUMO compared to 4. Thus, a redshift in its absorption and emission spectra is observed due to the decrease in HOMO–LUMO. The fluorescence quantum yield of 136 is 39.5%. The presence of oxygen atoms in the 5,10-dioxatruxene core enables effective π-stacking, making 5,10-dioxatruxenes promising materials for solution-processable organic semiconductors.
3.5. 5,10-Diazatruxene
The first references to 5,10-diazatruxene appeared in the work by El Sayed et al. in 2017.325 Consequently, the condensation of 56 was performed (Scheme 46). Subsequent studies also allowed condensation of 6-bromo326 and 5-nitroindoles,327 directly leading to doubly substituted 5,10-diazatruxenes. Moreover, the N-substituted indoles also undergo this reaction, allowing the direct synthesis of N-protected 5,10-diazatruxene-15-ones 140c,324 and condensation using a stoichiometric amount of MeSO3H acid in 1,2-dichloroethane.
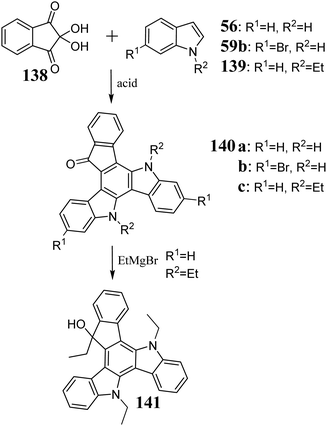 |
| Scheme 46 Synthesis and reactivity of 5,10-diazatruxen-15-ones. | |
In the presence of a base, 140a and b undergo ionization, allowing reaction with various electrophiles, such as acyl chlorides,325 simple alkyl halides, and more complex derivatives containing a piperidine or oxirane ring.327 Alternatively, reducing the carbonyl group in 140c by treatment with N2H4 in boiling ethylene glycol makes it possible to obtain the parent 5,10-diazatruxene 142,324 which after alkylation, is transformed to the highly soluble 143 (Scheme 47). Moreover, the presence of the carbonyl group enables the reaction between 140c and organometallic substances, leading to the appropriate 3° alcohols 141 (Scheme 46), significantly extending the functionalization possibilities of the 5,10-diazatruxene core.324 Alternatively, 143 can be easily converted to the corresponding tri and pentabromo derivatives 144 and 145, respectively, depending on the amount of Br2. 140b, 144, and 145 are crucial derivatives in further peripheral functionalization (Scheme 47).326
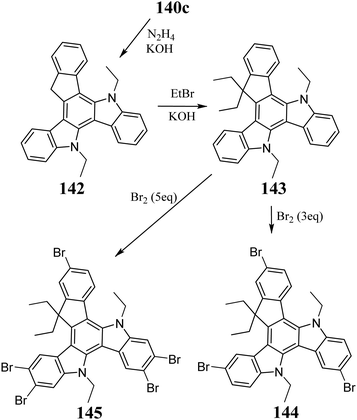 |
| Scheme 47 Synthesis and reactivity of 5,10-diazatruxene. | |
The two nitrogen atoms present in the aromatic system lift the electron level degeneration, destabilize the HOMO level significantly, and increase the fluorescence quantum yield (QY = 25.5%) compared to 4.324 5,10-Diazatruxene, like its dioxa counterpart, tends to π-stacking interactions. Its high HOMO energy and aggregation in the solid state suggest the possibility of using this type of substance as an HTM.
Peripherally functionalized 5,10-diazatruxen-15-ones with both donor 146 and acceptor 147 substituents were employed as promising semiconductors (Scheme 48). They exhibit reversible redox processes, which combined with their high π-stacking in the solid state, make them potential ambipolar semiconductors.326 Nevertheless, the interest in 5,10-diazatruxenes is not limited to optoelectronic applications. It has been found that the appropriate modification of the 5,10-diazatruxene core allows effective interaction with DNA, making it applicable as an anti-cancer agent.327
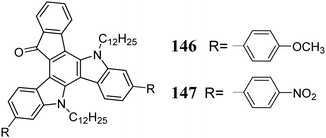 |
| Scheme 48 5,10-Diazatruxene-15-one-based D–A ambipolar semiconductors. | |
3.6. 5,10-Dithiatruxene
In 2009, Suze et al. described the condensation between benzo[b]thiophene 148 and 138 in AcOH and the presence of H2SO4, leading to 5,10-dithiatruxen-15-one 149, with a yield of 68% (Scheme 49).328 The same reaction can be performed using a stoichiometric amount of MeSO3H in 1,2-dichloroethane, increasing the yield to 74%.324 However, due to the negligible solubility resulting from π-stacking, obtaining 149 and its further functionalization are significantly impeded. Nevertheless, the reaction between 149 and organometallic reagents, leading to soluble 3° alcohol 150, is possible.324
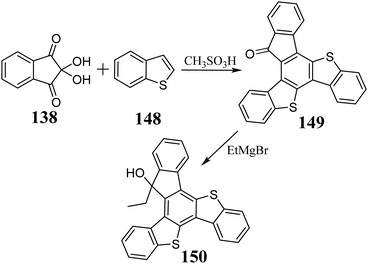 |
| Scheme 49 Synthesis and reactivity of 5,10-dithiatruxene-15-one. | |
The soluble 5,10-dithiatruxene 152 was synthesized for the first time in 2020 by Górski et al.151, in the presence of a catalytic amount of I2 and UV light, undergoes photocyclization to unsubstituted 5,10-dithiatruxene (Scheme 50). After C-alkylation, within a carbocyclic five-membered ring, its solubility significantly increases. By treating 152 with excess Br2, the appropriate bromo derivative 153 is obtained, with nearly quantitative yield.
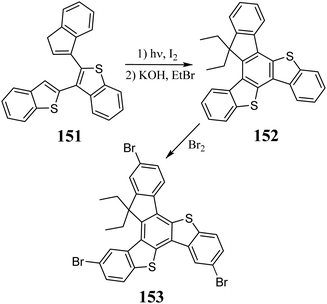 |
| Scheme 50 Synthesis and reactivity of 5,10-dithiatruxene. | |
The HOMO level of 5,10-dithiatruxene is destabilized compared to 4.324 Due to the low symmetry of the molecule, the degeneration of its electron levels is lifted. However, despite the electron transitions being allowed, 5,10-dithiatruxene has a relatively low fluorescence quantum yield. The ability to exhibit strong π-stacking interactions in the solid state, characteristic for 5,10-diheterotruxenes, is also observed in the case of 149 and 152. Therefore, 5,10-dithiatruxenes should be considered as potential organic semiconductors.
4. Conclusion
The present review summarized the knowledge on the synthesis and physicochemistry of (hetero)truxenes up to 2021, including their synthesis, reactivity, spectral, and redox properties. The parent heptacyclic, star-shaped hydrocarbon, can be modified towards the desired properties by either heteroatom displacement or peripheral functionalization. The presence of C3 symmetry significantly influences every aspect mentioned. To attract a broader group of readers, the physicochemical properties of (hetero)truxenes together with their potential applications were discussed. The ease of heterotruxene functionalization allows fitting physicochemical properties and constructing macromolecules, COFs, and MOFs. We highlighted the difficulties in synthesizing heteroanalogues and their different reactivity. Multiple citations next to each subsection allow the choice of a suitable synthetic path. This review is expected to be helpful for both synthetic and material chemists.
Conflicts of interest
There are no conflicts to declare.
Acknowledgements
This work was supported by National Science Centre (grant no. FUGA 4, 2015/16/S/ST4/00427) and in part by the Institute of Physical Chemistry, Polish Academy of Sciences.
References
- X. C. Li, C. Y. Wang, W. Y. Lai and W. Huang, J. Mater. Chem. C, 2016, 4, 10574–10587 Search PubMed.
- M. T. El Sayed, J. Heterocycl. Chem., 2018, 55, 21–43 Search PubMed.
- K. Shi, J. Y. Wang and J. Pei, Chem. Rec., 2015, 15, 52–78 Search PubMed.
- F. Goubard and F. Dumur, RSC Adv., 2015, 5, 3521–3551 Search PubMed.
- S. A. Wagay, I. A. Rather and R. Ali, ChemistrySelect, 2019, 4, 12272–12288 Search PubMed.
- C. Yao, Y. Yu, X. Yang, H. Zhang, Z. Huang, X. Xu, G. Zhou, L. Yue and Z. Wu, J. Mater. Chem. C, 2015, 3, 5783–5794 Search PubMed.
- Y. Liu, Y. Chen, H. Li, S. Wang, X. Wu, H. Tong and L. Wang, ACS Appl. Mater. Interfaces, 2020, 12, 30652–30658 Search PubMed.
- P. L. dos Santos, J. S. Ward, D. G. Congrave, A. S. Batsanov, J. Eng, J. E. Stacey, T. J. Penfold, A. P. Monkman and M. R. Bryce, Adv. Sci., 2018, 5, 1700989 (1–9) Search PubMed.
- M. R. Maciejczyk, S. Zhang, G. J. Hedley, N. Robertson, I. D. W. Samuel and M. Pietraszkiewicz, Adv. Funct. Mater., 2019, 1807572, 1–13 Search PubMed.
- C. Ruiz, I. Arrechea-Marcos, A. Benito-Hernández, E. Gutiérrez-Puebla, M. Á. Monge, J. T. López Navarrete, M. C. Ruiz Delgado, R. P. Ortiz and B. Gómez-Lor, J. Mater. Chem. C, 2018, 6, 50–56 Search PubMed.
- Y. Sun, K. Xiao, Y. Liu, J. Wang, J. Pei, G. Yu and D. Zhu, Adv. Funct. Mater., 2005, 15, 818–822 Search PubMed.
- S. Mula, T. Han, T. Heiser, P. Lévêque, N. Leclerc, A. P. Srivastava, A. Ruiz-Carretero and G. Ulrich, Chem. – Eur. J., 2019, 25, 8304–8312 Search PubMed.
- B. Pan, Y.-Z. Zhu, D. Ye, F. Li, Y.-F. Guo and J.-Y. Zheng, New J. Chem., 2018, 42, 4133–4141 Search PubMed.
- L. Zhang, X. Yang, W. Wang, G. G. Gurzadyan, J. Li, X. Li, J. An, Z. Yu, H. Wang, B. Cai, A. Hagfeldt and L. Sun, ACS Energy Lett., 2019, 4, 943–951 Search PubMed.
- L. Miu, S. Yan, H. Yao, Q. Chen, J. Zhang, Z. Wang, P. Cai, T. Hu, S. Ding, J. Chen, M. Liang and S. Yang, Dyes Pigm., 2019, 168, 1–11 Search PubMed.
- L. Wang, Q. Fang, Q. Lu, S.-J. Zhang, Y.-Y. Jin and Z.-Q. Liu, Org. Lett., 2015, 17, 4164–4167 Search PubMed.
- C. K. M. Chan, C.-H. Tao, H.-L. Tam, N. Zhu, V. W.-W. Yam and K.-W. Cheah, Inorg. Chem., 2009, 48, 2855–2864 Search PubMed.
- J. Shao, Z. Guan, Y. Yan, C. Jiao, Q.-H. Xu and C. Chi, J. Org. Chem., 2011, 76, 780–790 Search PubMed.
- G. He, J. Shao, Y. Li, J. Hu, H. Zhu, X. Wang, Q. Guo, C. Chi and A. Xia, Phys. Chem. Chem. Phys., 2016, 18, 6789–6798 Search PubMed.
- Y. Nailwal, A. D. D. Wonanke, M. A. Addicoat and S. K. Pal, Macromolecules, 2021, 54, 6595–6604 Search PubMed.
- K.-R. Wang, Y.-Q. Wang, H.-W. An, J.-C. Zhang and X.-L. Li, Chem. – Eur. J., 2013, 19, 2903–2909 Search PubMed.
- L. K. Macreadie, R. Babarao, C. J. Setter, S. J. Lee, O. T. Qazvini, A. J. Seeber, J. Tsanaktsidis, S. G. Telfer, S. R. Batten and M. R. Hill, Angew. Chem., Int. Ed., 2020, 59, 6090–6098 Search PubMed.
- L. Yang, Y. Ma, Y. Xu and G. Chang, Chem. Commun., 2019, 55, 11227–11230 Search PubMed.
- L. Yang, W. Wei, Y. Ma, Y. Xu and G. Chang, J. Power Sources, 2020, 449, 227551 Search PubMed.
- G. W. Kim, R. Lampande, D. C. Choe, H. W. Bae and J. H. Kwon, Thin Solid Films, 2015, 589, 105–110 Search PubMed.
- X. Yang, D. Zhang, Y. Liao and D. Zhao, J. Org. Chem., 2020, 85, 5761–5770 Search PubMed.
- T. Kirschbaum, F. Rominger and M. Mastalerz, Angew. Chem., Int. Ed., 2020, 59, 270–274 Search PubMed.
- R. Dorel and A. M. Echavarren, Acc. Chem. Res., 2019, 52, 1812–1823 Search PubMed.
- X. Wang, P. Peng, W. Xuan, Y. Wang, Y. Zhuang, Z. Tian and X. Cao, Org. Biomol. Chem., 2018, 16, 34–37 Search PubMed.
- J. Zhu, D. Zhang, T. K. Ronson, W. Wang, L. Xu, H. Yang and J. R. Nitschke, Angew. Chem., Int. Ed., 2021, 11789–11792 Search PubMed.
- M. Yamashina, Y. Tanaka, R. Lavendomme, T. K. Ronson, M. Pittelkow and J. R. Nitschke, Nature, 2019, 574, 511–515 Search PubMed.
- X. Yang, S. Huang, M. Ortiz, X. Wang, Y. Cao, O. Kareem, Y. Jin, F. Huang, X. Wang and W. Zhang, Org. Chem. Front., 2021, 8, 4723–4729 Search PubMed.
-
M. M. Boorum and L. T. Scott, Modern Arene Chemistry, Wiley-VCH Verlag GmbH & Co. KGaA, Weinheim, FRG, 2002, pp. 20–31 Search PubMed.
- G. Metz, Synthesis, 1972, 614–615 Search PubMed.
- E. V. Dehmlow and T. Kelle, Synth. Commun., 1997, 27, 2021–2031 Search PubMed.
- T. W. Warmerdam, R. J. M. Nolte, W. Drenth, J. C. Van Miltenburg, D. Frenkel and R. J. J. Zijlstra, Liq. Cryst., 1988, 3, 1087–1104 Search PubMed.
- B. Gómez-Lor, Ó. de Frutos, P. A. Ceballos, T. Granier and A. M. Echavarren, Eur. J. Org. Chem., 2001, 2107–2114 Search PubMed.
- A. W. Amick and L. T. Scott, J. Org. Chem., 2007, 72, 3412–3418 Search PubMed.
-
GB 2520791 A, 2015 Search PubMed.
- H. Terai, H. Takaya and T. Naota, Tetrahedron Lett., 2006, 47, 1705–1708 Search PubMed.
- J.-B. Chen, C. Zhou, R.-Q. Lu, X.-C. Wang, H. Qu, M. Saha, H.-L. Liu, H. Zhang and X.-Y. Cao, Chem. – Eur. J., 2019, 25, 1293–1299 Search PubMed.
- S. S. Elmorsy, A. Pelter, K. Smith, M. B. Hursthouse and D. Ando, Tetrahedron Lett., 1992, 33, 821–824 Search PubMed.
- H. Isla, B. Grimm, E. M. Pérez, M. Rosario Torres, M. Ángeles Herranz, R. Viruela, J. Aragó, E. Ortí, D. M. Guldi and N. Martín, Chem. Sci., 2012, 3, 498–508 Search PubMed.
- L. Sanguinet, J. C. Williams, Z. Yang, R. J. Twieg, G. Mao, K. D. Singer, G. Wiggers and R. G. Petschek, Chem. Mater., 2006, 18, 4259–4269 Search PubMed.
- Y. N. Oded and I. Agranat, Tetrahedron Lett., 2014, 55, 636–638 Search PubMed.
- X. Zhang, X. Ji, R. Su, B. L. Weeks, Z. Zhang and S. Deng, ChemPlusChem, 2013, 78, 703–711 Search PubMed.
- G. Zhang, V. Lami, F. Rominger, Y. Vaynzof and M. Mastalerz, Angew. Chemie, 2016, 128, 4045–4049 Search PubMed.
- J.-Y. Wang, J. Yan, L. Ding, Y. Ma and J. Pei, Adv. Funct. Mater., 2009, 19, 1746–1752 Search PubMed.
- H. Jiang, N. Lai, J. Tang, X. Tian, F. Wei, W. Bai and Y. Xu, J. Photochem. Photobiol., A, 2019, 369, 195–201 Search PubMed.
- D. Raksasorn, S. Namuangruk, N. Prachumrak, T. Sudyoadsuk, V. Promarak, M. Sukwattanasinitt and P. Rashatasakhon, RSC Adv., 2015, 5, 72841–72848 Search PubMed.
- T. L. Tisch, T. J. Lynch and R. Dominguez, J. Organomet. Chem., 1989, 377, 265–273 Search PubMed.
- Ó. de Frutos, B. Gómez-Lor, T. Granier, M. Á. Monge, E. Gutiérrez-Puebla and A. M. Echavarren, Angew. Chem., Int. Ed., 1999, 38, 204–207 Search PubMed.
- H. Satoh, G. Yamamoto and Y. Mazaki, Bull. Chem. Soc. Jpn., 2006, 79, 938–943 Search PubMed.
- D. K. Frantz, J. J. Walish and T. M. Swager, Org. Lett., 2013, 15, 4782–4785 Search PubMed.
- K. Isoda, T. Yasuda and T. Kato, Chem. – Asian J., 2009, 4, 1619–1625 Search PubMed.
- E. M. Pérez, M. Sierra, L. Sánchez, M. R. Torres, R. Viruela, P. M. Viruela, E. Ortí and N. Martín, Angew. Chem., Int. Ed., 2007, 46, 1847–1851 Search PubMed.
- M. Kimura, S. Kuwano, Y. Sawaki, H. Fujikawa, K. Noda, Y. Taga and K. Takagi, J. Mater. Chem., 2005, 15, 2393–2398 Search PubMed.
- M. Ruiz, B. Gómez-Lor, A. Santos and A. M. Echavarren, Eur. J.
Org. Chem., 2004, 858–866 Search PubMed.
- S. Kotha, R. Ali, N. R. Panguluri, A. Datta and K. K. Kannaujiya, Tetrahedron Lett., 2018, 59, 4080–4085 Search PubMed.
- M.-T. Kao, J.-H. Chen, Y.-Y. Chu, K.-P. Tseng, C.-H. Hsu, K.-T. Wong, C.-W. Chang, C.-P. Hsu and Y.-H. Liu, Org. Lett., 2011, 13, 1714–1717 Search PubMed.
- X.-Y. Cao, W.-B. Zhang, J.-L. Wang, X.-H. Zhou, H. Lu and J. Pei, J. Am. Chem. Soc., 2003, 125, 12430–12431 Search PubMed.
- X.-Y. Cao, X.-H. Liu, X.-H. Zhou, Y. Zhang, Y. Jiang, Y. Cao, Y.-X. Cui and J. Pei, J. Org. Chem., 2004, 69, 6050–6058 Search PubMed.
- L. Liu and S. G. Telfer, J. Am. Chem. Soc., 2015, 137, 3901–3909 Search PubMed.
- T.-C. Lin, B.-K. Tsai, T.-Y. Huang, W. Chien, Y.-Y. Liu, M.-H. Li and M.-Y. Tsai, Dyes Pigm., 2015, 120, 99–111 Search PubMed.
- K. Lin, S. Wang, Z. Wang, Q. Yin, X. Liu, J. Jia, X. Jia, P. Luo, X. Jiang, C. Duan, F. Huang and Y. Cao, Front. Chem., 2018, 6, 328 Search PubMed.
- M. Tasior, O. Vakuliuk, D. Koga, B. Koszarna, K. Górski, M. Grzybowski, Ł. Kielesiński, M. Krzeszewski and D. T. Gryko, J. Org. Chem., 2020, 85, 13529–13543 Search PubMed.
- M. Sen Yuan, Q. Fang, Y. R. Zhang and Q. Wang, Spectrochim. Acta, Part A, 2011, 79, 1112–1115 Search PubMed.
- H.-W. Wen and P.-C. Yang, RSC Adv., 2016, 6, 60308–60317 Search PubMed.
- X.-Y. Cao, X.-H. Zhou, H. Zi and J. Pei, Macromolecules, 2004, 37, 8874–8882 Search PubMed.
- W.-Y. Lai, Q.-Y. He, Z. Ma and W. Huang, Chem. Lett., 2009, 38, 286–287 Search PubMed.
- X.-Y. Cao, W. Zhang, H. Zi and J. Pei, Org. Lett., 2004, 6, 4845–4848 Search PubMed.
- S. Diring and R. Ziessel, Tetrahedron Lett., 2009, 50, 1203–1208 Search PubMed.
- X. Yang, Q. Zheng, C. Tang, D. Cai, S.-C. Chen and Y. Ma, Dyes Pigm., 2013, 99, 366–373 Search PubMed.
- X. Xu, D. Sun, J. Yang, G. Zhu, Y. Fang, C. P. Gros, F. Bolze and H.-J. Xu, Dyes Pigm., 2020, 179, 108380 Search PubMed.
- J. Yang, F.-J. Cai, X.-M. Yuan, T. Meng, G.-X. Xin, S.-F. Wang, C. P. Gros and H.-J. Xu, J. Porphyr. Phthalocyanines, 2018, 22, 777–783 Search PubMed.
- Y. Kim, S. Das, S. Bhattacharya, S. Hong, M. G. Kim, M. Yoon, S. Natarajan and K. Kim, Chem. – Eur. J., 2012, 18, 16642–16648 Search PubMed.
- J. Yang, H. Jiang, N. Desbois, G. Zhu, C. P. Gros, Y. Fang, F. Bolze, S. Wang and H.-J. Xu, Dyes Pigm., 2020, 176, 108183 Search PubMed.
- Y. Wang, H. Fang, I. Tranca, H. Qu, X. Wang, A. J. Markvoort, Z. Tian and X. Cao, Nat. Commun., 2018, 9, 488 Search PubMed.
- B. Du, D. Fortin and P. D. Harvey, Inorg. Chem., 2011, 50, 11493–11505 Search PubMed.
- Y. Wu, X. Hao, J. Wu, J. Jin and X. Ba, Macromolecules, 2010, 43, 731–738 Search PubMed.
- G. Zhang, F. Rominger and M. Mastalerz, Chem. – Eur. J., 2016, 22, 3084–3093 Search PubMed.
- L. Zhao, W. Wang and M.-S. Yuan, Spectrochim. Acta, Part A, 2015, 135, 63–68 Search PubMed.
- F. Li, B. Zhao, Y. Chen, Y. Zhang, T. Wang and S. Xue, Spectrochim. Acta, Part A, 2017, 185, 20–26 Search PubMed.
- B. Kaur, S. Karuthedath, C. S. P. De Castro, F. Laquai and J. Jacob, ChemistrySelect, 2020, 5, 109–116 Search PubMed.
- H. Tsuji, Y. Ota, S. Furukawa, C. Mitsui, Y. Sato and E. Nakamura, Asian J. Org. Chem., 2012, 1, 34–37 Search PubMed.
- M.-Y. Wang, Q.-J. Zhang, Q.-Q. Shen, Q.-Y. Li and S.-J. Ren, Chin. J. Polym. Sci., 2020, 38, 151–157 Search PubMed.
- E. Aktas, J. Jiménez-López, C. Rodríguez-Seco, R. Pudi, M. A. Ortuño, N. López and E. Palomares, ChemPhysChem, 2019, 20, 2702–2711 Search PubMed.
- Y. Che, X. Yuan, F. Cai, J. Zhao, X. Zhao, H. Xu and L. Liu, Dyes Pigm., 2019, 171, 107756 Search PubMed.
- M. Pfeffermann, R. Dong, R. Graf, W. Zajaczkowski, T. Gorelik, W. Pisula, A. Narita, K. Müllen and X. Feng, J. Am. Chem. Soc., 2015, 137, 14525–14532 Search PubMed.
- W.-Y. Lai, D. Liu and W. Huang, Macromol. Chem. Phys., 2011, 212, 445–454 Search PubMed.
- K. M. Choi, K.-Y. Kim, T.-D. Kim, R. R. Das, B. K. Choi, J. J. Park, J.-M. Kim and K.-S. Lee, J. Nanosci. Nanotechnol., 2010, 10, 6916–6919 Search PubMed.
- J. Huang, B. Xu, J. H. Su, C. H. Chen and H. Tian, Tetrahedron, 2010, 66, 7577–7582 Search PubMed.
- Y. Xie, X. Zhang, Y. Xiao, Y. Zhang, F. Zhou, J. Qi and J. Qu, Chem. Commun., 2012, 48, 4338–4340 Search PubMed.
- X. Zhang, H. Song, J. Xiao, T. Ren, S. Wang, Z. Liu, X. Ba and Y. Wu, Aust. J. Chem., 2015, 68, 505–512 Search PubMed.
- R. Sharma, D. Volyniuk, C. Popli, O. Bezvikonnyi, J. V. Grazulevicius and R. Misra, J.
Phys. Chem. C, 2018, 122, 15614–15624 Search PubMed.
- C. Tang, X.-D. Liu, F. Liu, X.-L. Wang, H. Xu, R.-L. Liu, Z. Rong and W. Huang, J. Chem. Res., 2013, 37, 242–247 Search PubMed.
- X.-F. Duan, J.-L. Wang and J. Pei, Org. Lett., 2005, 7, 4071–4074 Search PubMed.
- X. Wen, D. Zhang, T. Ren, J. Xiao, Y. Wu, L. Bai and X. Ba, Dyes Pigm., 2017, 137, 437–444 Search PubMed.
- Y.-D. Jiu, C.-F. Liu, J.-Y. Wang, W.-Y. Lai, Y. Jiang, W.-D. Xu, X.-W. Zhang and W. Huang, Polym. Chem., 2015, 6, 8019–8028 Search PubMed.
- Y. Fan, Q. Wen, T.-G. Zhan, Q.-Y. Qi, J.-Q. Xu and X. Zhao, Chem. – Eur. J., 2017, 23, 5668–5672 Search PubMed.
- Q. Zhang, C. Zhang, L. Cao, Z. Wang, B. An, Z. Lin, R. Huang, Z. Zhang, C. Wang and W. Lin, J. Am. Chem. Soc., 2016, 138, 5308–5315 Search PubMed.
- W.-Y. Lai, R. Xia, D. D. C. Bradley and W. Huang, Chem. – Eur. J., 2010, 16, 8471–8479 Search PubMed.
- Y. Jiang, Y.-X. Lu, Y.-X. Cui, Q.-F. Zhou, Y. Ma and J. Pei, Org. Lett., 2007, 9, 4539–4542 Search PubMed.
- J.-M. Koenen, S. Jung, A. Patra, A. Helfer and U. Scherf, Adv. Mater., 2012, 24, 681–686 Search PubMed.
- Q.-Q. Chen, F. Liu, Z. Ma, B. Peng, W. Wei and W. Huang, Synlett, 2007, 3145–3148 Search PubMed.
- X.-Y. Cao, H. Zi, W. Zhang, H. Lu and J. Pei, J. Org. Chem., 2005, 70, 3645–3653 Search PubMed.
- R. Sharma, R. Maragani and R. Misra, New J. Chem., 2018, 42, 882–890 Search PubMed.
- R. Misra, R. Sharma and S. M. Mobin, Dalton Trans., 2014, 43, 6891–6896 Search PubMed.
- R. Sharma, M. B. Thomas, R. Misra and F. D’Souza, Angew. Chem., Int. Ed., 2019, 58, 4350–4355 Search PubMed.
- S. Diring, F. Puntoriero, F. Nastasi, S. Campagna and R. Ziessel, J. Am. Chem. Soc., 2009, 131, 6108–6110 Search PubMed.
- J.-L. Wang, X.-F. Duan, B. Jiang, L.-B. Gan, J. Pei, C. He and Y.-F. Li, J. Org. Chem., 2006, 71, 4400–4410 Search PubMed.
- B. Ventura, A. Barbieri, F. Barigelletti, S. Diring and R. Ziessel, Inorg. Chem., 2010, 49, 8333–8346 Search PubMed.
- J.-L. Wang, Y.-T. Chan, C. N. Moorefield, J. Pei, D. A. Modarelli, N. C. Romano and G. R. Newkome, Macromol. Rapid Commun., 2010, 31, 850–855 Search PubMed.
- S. Diring, R. Ziessel, F. Barigelletti, A. Barbieri and B. Ventura, Chem. – Eur. J., 2010, 16, 9226–9236 Search PubMed.
- C. K. M. Chan, C.-H. Tao, K. F. Li, K. Man-Chung Wong, N. Zhu, K.-W. Cheah and V. W. W. Yam, J. Organomet. Chem., 2011, 696, 1163–1173 Search PubMed.
- C. Po, C.-H. Tao, K.-F. Li, C. K. M. Chan, H. L.-K. Fu, K.-W. Cheah and V. W.-W. Yam, Inorg. Chim. Acta, 2019, 488, 214–218 Search PubMed.
- H. Norouzi-Arasi, A. K. Pal, S. Nag, D. Chartrand and G. S. Hanan, Chem. Commun., 2016, 52, 12159–12162 Search PubMed.
- R. Dorel, P. de Mendoza, P. Calleja, S. Pascual, E. González-Cantalapiedra, N. Cabello and A. M. Echavarren, Eur. J. Org. Chem., 2016, 3171–3176 Search PubMed.
- B. Gómez-Lor, Ó. de Frutos, A. M. Echavarren and B. Gómez-Lor, Chem. Commun., 1999, 2431–2432 Search PubMed.
- K. Y. Amsharov, N. Abdurakhmanova, S. Stepanow, S. Rauschenbach, M. Jansen and K. Kern, Angew. Chem., Int. Ed., 2010, 49, 9392–9396 Search PubMed.
- K. Y. Amsharov and M. Jansen, Chem. Commun., 2009, 2691–2693 Search PubMed.
- A. Mueller and K. Y. Amsharov, Eur. J. Org. Chem., 2012, 6155–6164 Search PubMed.
- D. Baunsgaard, N. Harrit, M. El Balsami, F. Negri, G. Orlandi, J. Frederiksen and R. Wilbrandt, J. Phys. Chem. A, 1998, 102, 10007–10016 Search PubMed.
- T. Ogaki, E. Ohta, Y. Oda, H. Sato, Y. Matsui, M. Kumeda and H. Ikeda, Asian J. Org. Chem., 2017, 6, 290–296 Search PubMed.
- K. Górski, K. Noworyta and J. Mech-Piskorz, RSC Adv., 2020, 10, 42363–42377 Search PubMed.
- R. Wang, K. Jiang, H. Yu, F. Wu, L. Zhu and H. Yan, Mater. Chem. Front., 2019, 3, 2137–2142 Search PubMed.
- T. H. Nguyen, J. Malthete and C. Destrade, Mol. Cryst. Liq. Cryst., 1981, 64, 291–298 Search PubMed.
- J. M. Buisine, R. Cayuela, C. Destrade and N. H. Tinh, Mol. Cryst. Liq. Cryst., 1987, 144, 137–160 Search PubMed.
- C. Destrade, P. Foucher, J. Malthete and T. H. Nguyen, Phys. Lett., 1982, 88, 187–190 Search PubMed.
- J. Lejay and M. Pesquer, Mol. Cryst. Liq. Cryst., 1983, 95, 31–43 Search PubMed.
- J. Lejay and M. Pesquer, Mol. Cryst. Liq. Cryst., 1984, 111, 293–310 Search PubMed.
- C. Destrade, H. Gasparoux, A. Babeau, N. Huu Tinh and J. Malthete, Mol. Cryst. Liq. Cryst., 1981, 67, 37–47 Search PubMed.
- S. Gómez-Esteban, A. Benito-Hernandez, R. Termine, G. Hennrich, J. T. L. Navarrete, M. C. Ruiz Delgado, A. Golemme and B. Gómez-Lor, Chem. – Eur. J., 2018, 24, 3576–3583 Search PubMed.
- D. R. Vinayakumara, M. Kumar, P. Sreekanth, R. Philip and S. Kumar, RSC Adv., 2015, 5, 26596–26603 Search PubMed.
- W.-B. Zhang, W.-H. Jin, X.-H. Zhou and J. Pei, Tetrahedron, 2007, 63, 2907–2914 Search PubMed.
- J. Pei, J.-L. Wang, X.-Y. Cao, X.-H. Zhou and W.-B. Zhang, J. Am. Chem. Soc., 2003, 125, 9944–9945 Search PubMed.
- J.-L. Wang, J. Luo, L.-H. Liu, Q.-F. Zhou, Y. Ma and J. Pei, Org. Lett., 2006, 8, 2281–2284 Search PubMed.
- A. L. Kanibolotsky, R. Berridge, P. J. Skabara, I. F. Perepichka, D. D. C. Bradley and M. Koeberg, J. Am. Chem. Soc., 2004, 126, 13695–13702 Search PubMed.
- Q.-Q. Chen, F. Liu, Z. Ma, B. Peng, W. Wei and W. Huang, Chem. Lett., 2008, 37, 178–179 Search PubMed.
- Y. Jiang, J.-Y. Wang, Y. Ma, Y.-X. Cui, Q.-F. Zhou and J. Pei, Org. Lett., 2006, 8, 4287–4290 Search PubMed.
- N. A. Montgomery, J.-C. Denis, S. Schumacher, A. Ruseckas, P. J. Skabara, A. L. Kanibolotsky, M. J. Paterson, I. Galbraith, G. A. Turnbull and I. D. W. Samuel, J. Phys. Chem. A, 2011, 115, 2913–2919 Search PubMed.
- M. M. Oliva, J. Casado, J. T. López Navarrete, R. Berridge, P. J. Skabara, A. L. Kanibolotsky and I. F. Perepichka, J. Phys. Chem. B, 2007, 111, 4026–4035 Search PubMed.
- K. M. Omer, A. L. Kanibolotsky, P. J. Skabara, I. F. Perepichka and A. J. Bard, J. Phys. Chem. B, 2007, 111, 6612–6619 Search PubMed.
- Z. Mohsan, A. L. Kanibolotsky, A. J. Stewart, A. R. Inigo, L. Dennany and P. J. Skabara, J. Mater. Chem. C, 2015, 3, 1166–1171 Search PubMed.
- M. Wu, Z. Gong, A. J. C. Kuehne, A. L. Kanibolotsky, Y. J. Chen, I. F. Perepichka, A. R. Mackintosh, E. Gu, P. J. Skabara, R. A. Pethrick and M. D. Dawson, Opt. Express, 2009, 17, 16436–16443 Search PubMed.
- J. L. Wang, Z. He, H. Wu, H. Cui, Y. Li, Q. Gong, Y. Cao and J. Pei, Chem. – Asian J., 2010, 5, 1455–1465 Search PubMed.
- S. C. Yuan, Q. Sun, T. Lei, B. Du, Y. F. Li and J. Pei, Tetrahedron, 2009, 65, 4165–4172 Search PubMed.
- J.-L. Wang, J. Yan, Z.-M. Tang, Q. Xiao, Y. Ma and J. Pei, J. Am. Chem. Soc., 2008, 130, 9952–9962 Search PubMed.
- J.-L. Wang, Z.-M. Tang, Q. Xiao, Y. Ma and J. Pei, Org. Lett., 2009, 11, 863–866 Search PubMed.
- C. R. Belton, A. L. Kanibolotsky, J. Kirkpatrick, C. Orofino, S. E. T. Elmasly, P. N. Stavrinou, P. J. Skabara and D. D. C. Bradley, Adv. Funct. Mater., 2013, 23, 2792–2804 Search PubMed.
- Y. Wang, G. Tsiminis, Y. Yang, A. Ruseckas, A. L. Kanibolotsky, I. F. Perepichka, P. J. Skabara, G. A. Turnbull and I. D. W. Samuel, Synth. Met., 2010, 160, 1397–1400 Search PubMed.
- C. Liu, T. Lu, W. Lai and W. Huang, Chem. – Asian J., 2019, 14, 3442–3448 Search PubMed.
- W. Xu, J. Yi, W.-Y. Lai, L. Zhao, Q. Zhang, W. Hu, X.-W. Zhang, Y. Jiang, L. Liu and W. Huang, Adv. Funct. Mater., 2015, 25, 4617–4625 Search PubMed.
-
CN 107445849 A, 2017, 1–20 Search PubMed.
- H. Zhang, X. Liu, T. Lu, P. Lv and W. Lai, Chem. – Eur. J., 2019, 25, 3909–3917 Search PubMed.
- B. Guzelturk, A. L. Kanibolotsky, C. Orofino-Pena, N. Laurand, M. D. Dawson, P. J. Skabara and H. V. Demir, J. Mater. Chem. C, 2015, 3, 12018–12025 Search PubMed.
- H. Zhou, X. Zhao, T. Huang, R. Lu, H. Zhang, X. Qi, P. Xue, X. Liu and X. Zhang, Org. Biomol. Chem., 2011, 9, 1600–1607 Search PubMed.
- X. Zhou, J.-K. Feng and A.-M. Ren, Synth. Met., 2005, 155, 615–617 Search PubMed.
- Q. Lv, S. Feng, L. Jing, Q. Zhang, M. Qi, J. Wang, H. Bai and R. Fu, J. Chromatogr. A, 2016, 1454, 114–119 Search PubMed.
- Q. Zhang, M. Qi and J. Wang, J. Chromatogr. A, 2017, 1525, 152–160 Search PubMed.
- Y. Yang, M. Qi and J. Wang, J. Chromatogr. A, 2018, 1578, 67–75 Search PubMed.
- M. Wang, Y. Yang, M. Qi and J. Wang, RSC Adv., 2017, 7, 44665–44672 Search PubMed.
- C. Cheng, Y. Jiang, C.-F. Liu, J.-D. Zhang, W.-Y. Lai and W. Huang, Chem. – Asian J., 2016, 11, 3589–3597 Search PubMed.
- J. Wang, Y. Chen, M. Liang, G. Ge, R. Zhou, Z. Sun and S. Xue, Dyes Pigm., 2016, 125, 399–406 Search PubMed.
- L. Guan, X. Yin, D. Zhao, C. Wang, Q. An, J. Yu, N. Shrestha, C. R. Grice, R. A. Awni, Y. Yu, Z. Song, J. Zhou, W. Meng, F. Zhang, R. J. Ellingson, J. Wang, W. Tang and Y. Yan, J. Mater. Chem. A, 2017, 5, 23319–23327 Search PubMed.
-
CN 107353891 A, 2017 Search PubMed.
- C. Wongsilarat, S. Namuangruk, N. Prachumrak, T. Sudyoadsuk, V. Promarak, M. Sukwattanasinitt and P. Rashatasakhon, Org. Electron., 2018, 57, 352–358 Search PubMed.
- R. Grisorio, R. Iacobellis, A. Listorti, L. De Marco, M. P. Cipolla, M. Manca, A. Rizzo, A. Abate, G. Gigli and G. P. Suranna, ACS Appl. Mater. Interfaces, 2017, 9, 24778–24787 Search PubMed.
- B. Wang, Q. Zeng, Z. Sun, S. Xue and M. Liang, Dyes Pigm., 2019, 165, 81–89 Search PubMed.
- C. Huang, W. Fu, C.-Z. Li, Z. Zhang, W. Qiu, M. Shi, P. Heremans, A. K. Y. Jen and H. Chen, J. Am. Chem. Soc., 2016, 138, 2528–2531 Search PubMed.
- Z. Yang, B. Xu, J. He, L. Xue, Q. Guo, H. Xia and W. Tian, Org. Elelctron., 2009, 10, 954–959 Search PubMed.
- H.-L. Ni, H. Monobe, P. Hu, B.-Q. Wang, Y. Shimizu and K.-Q. Zhao, Liq. Cryst., 2013, 40, 411–420 Search PubMed.
- H. Monobe, C. Chen, K. Q. Zhao, P. Hu, Y. Miyake, A. Fujii, M. Ozaki and Y. Shimizu, Mol. Cryst. Liq. Cryst., 2011, 545, 149–155 Search PubMed.
- B. Kaur, D. Moghe, A. Dey, D. Kabra and J. Jacob, J. Lumin., 2018, 196, 511–519 Search PubMed.
- L. Ma, Z. Wu, G. Zhou, F. Yuan, Y. Yu, C. Yao, S. Ning, X. Hou, Y. Li, S. Wang and Q. Gong, J. Mater. Chem. C, 2015, 3, 7004–7013 Search PubMed.
- L. Sánchez, N. Martín, E. González-Cantalapiedra, A. M. Echavarren, G. M. Aminur Rahman and D. M. Guldi, Org. Lett., 2006, 8, 2451–2454 Search PubMed.
- F. Tang, K. Wu, Z. Zhou, G. Wang, Y. Pei, B. Zhao and S. Tan, Dyes Pigm., 2018, 156, 276–284 Search PubMed.
- D. Tang, J. Wan, X. Xu, Y. W. Lee, H. Y. Woo, K. Feng and Q. Peng, Nano Energy, 2018, 53, 258–269 Search PubMed.
- K. Lin, B. Xie, Z. Wang, R. Xie, Y. Huang, C. Duan, F. Huang and Y. Cao, Org. Electron., 2018, 52, 42–50 Search PubMed.
- W. Wu, G. Zhang, X. Xu, S. Wang, Y. Li and Q. Peng, Adv. Funct. Mater., 2018, 28, 1707493 Search PubMed.
- K. Li, R. Xie, W. Zhong, K. Lin, L. Ying, F. Huang and Y. Cao, Sci. China: Chem., 2018, 61, 576–583 Search PubMed.
- X. Xu, G. Zhang, L. Yu, R. Li and Q. Peng, Adv. Mater., 2019, 31, 1906045 Search PubMed.
- M.-S. Yuan, Q. Fang, Z.-Q. Liu, J.-P. Guo, H.-Y. Chen, W.-T. Yu, G. Xue and D.-S. Liu, J. Org. Chem., 2006, 71, 7858–7861 Search PubMed.
- Y. Hao, M. Liang, Z. Wang, F. Cheng, C. Wang, Z. Sun and S. Xue, Tetrahedron, 2013, 69, 10573–10580 Search PubMed.
- G. Ge, P. Dai, Z. Lu, M. Liang, H. Dong, Z. Sun and S. Xue, Dyes Pigm., 2016, 128, 8–18 Search PubMed.
- M. Liang, M. Lu, Q.-L. Wang, W.-Y. Chen, H.-Y. Han, Z. Sun and S. Xue, J. Power Sources, 2011, 196, 1657–1664 Search PubMed.
- M.-S. Yuan, Q. Wang, W. Wang, D.-E. Wang, J. Wang and J. Wang, Analyst, 2014, 139, 1541–1549 Search PubMed.
- F. Wu, J.-L. Liu, L. T. L. Lee, T. Chen, M. Wang and L.-N. Zhu, Chin. Chem. Lett., 2015, 26, 955–962 Search PubMed.
- G. V. Baryshnikov, B. F. Minaev, V. A. Minaeva, Z. Ning and Q. Zhang, Opt. Spectrosc., 2012, 112, 168–174 Search PubMed.
- P. Dai, H. Dong, M. Liang, H. Cheng, Z. Sun and S. Xue, ACS Sustainable Chem. Eng., 2017, 5, 97–104 Search PubMed.
- Y. Hao, M. Liang, Z. Wang, L. Wang, Y. Sun, Z. Sun and S. Xue, Phys. Chem. Chem. Phys., 2013, 15, 15441–15449 Search PubMed.
- X. Zong, M. Liang, C. Fan, K. Tang, G. Li, Z. Sun and S. Xue, J. Phys. Chem. C, 2012, 116, 11241–11250 Search PubMed.
- M.-S. Yuan, Z.-Q. Liu and Q. Fang, J. Org. Chem., 2007, 72, 7915–7922 Search PubMed.
- P. Sam-ang, K. Silpcharu, M. Sukwattanasinitt and P. Rashatasakhon, J. Fluoresc., 2019, 29, 417–424 Search PubMed.
- P.-C. Yang, H.-W. Wen, C.-W. Huang and Y.-N. Zhu, RSC Adv., 2016, 6, 87680–87689 Search PubMed.
- P. Sam-Ang, D. Raksasorn, M. Sukwattanasinitt and P. Rashatasakhon, RSC Adv., 2014, 4, 58077–58082 Search PubMed.
- W. Huang, E. Smarsly, J. Han, M. Bender, K. Seehafer, I. Wacker, R. R. Schröder and U. H. F. Bunz, ACS Appl. Mater. Interfaces, 2017, 9, 3068–3074 Search PubMed.
- N. Earmrattana, M. Sukwattanasinitt and P. Rashatasakhon, Dyes Pigm., 2012, 93, 1428–1433 Search PubMed.
- M. Echeverri, S. Gámez-Valenzuela, R. C. González-Cano, J. Guadalupe, S. Cortijo-Campos, J. T. López Navarrete, M. Iglesias, M. C. Ruiz Delgado and B. Gómez-Lor, Chem. Mater., 2019, 31, 6971–6978 Search PubMed.
- Q. Zhao, S.-H. Li, R.-L. Chai, X. Ren and C. Zhang, ACS Appl. Mater. Interfaces, 2020, 12, 7504–7509 Search PubMed.
- I. Gadwal, G. Sheng, R. L. Thankamony, Y. Liu, H. Li and Z. Lai, ACS Appl. Mater. Interfaces, 2018, 10, 12295–12299 Search PubMed.
- S. Che, J. Pang, A. J. Kalin, C. Wang, X. Ji, J. Lee, D. Cole, J.-L. Li, X. Tu, Q. Zhang, H.-C. Zhou and L. Fang, ACS Mater. Lett., 2020, 2, 49–54 Search PubMed.
- Q. Zhang, Y. Sun, H. Li, K. Tang, Y. Zhong, D. Wang, Y. Guo and Y. Liu, Research, 2021, 2021, 1–8 Search PubMed.
- K. Lin, Z. Wang, Z. Hu, P. Luo, X. Yang, X. Zhang, M. Rafiq, F. Huang and Y. Cao, J. Mater. Chem. A, 2019, 7, 19087–19093 Search PubMed.
- A. Valverde-González, C. G. López Calixto, M. Barawi, M. Gomez-Mendoza, V. A. de la Peña O’Shea, M. Liras, B. Gómez-Lor and M. Iglesias, ACS Appl. Energy Mater., 2020, 3, 4411–4420 Search PubMed.
- V. R. Battula, H. Singh, S. Kumar, I. Bala, S. K. Pal and K. Kailasam, ACS Catal., 2018, 8, 6751–6759 Search PubMed.
- S. Gámez-Valenzuela, M. Echeverri, B. Gómez-Lor, J. I. Martínez and M. C. Ruiz Delgado, J. Mater. Chem. C, 2020, 8, 15416–15425 Search PubMed.
- S. Furukawa, J. Kobayashi and T. Kawashima, Dalton Trans., 2010, 39, 9329–9336 Search PubMed.
- T. Ureshino, T. Yoshida, Y. Kuninobu and K. Takai, J. Am. Chem. Soc., 2010, 132, 14324–14326 Search PubMed.
- K. Tamao, M. Uchida, T. Izumizawa, K. Furukawa and S. Yamaguchi, J. Am. Chem. Soc., 1996, 118, 11974–11975 Search PubMed.
- N. Bergman and J. Eklund, Tetrahedron, 1980, 36, 1445–1450 Search PubMed.
- J. Bergman and N. Eklund, Tetrahedron, 1980, 36, 1439–1443 Search PubMed.
- P. Manini, M. d’Ischia, M. Milosa and G. Prota, J. Org. Chem., 1998, 63, 7002–7008 Search PubMed.
- P. Manini, V. Criscuolo, L. Ricciotti, A. Pezzella, M. Barra, A. Cassinese, O. Crescenzi, M. G. Maglione, P. Tassini, C. Minarini, V. Barone and M. D’Ischia, ChemPlusChem, 2015, 80, 919–927 Search PubMed.
- L. Ginnari-Satriani, V. Casagrande, A. Bianco, G. Ortaggi and M. Franceschin, Org. Biomol. Chem., 2009, 7, 2513–2516 Search PubMed.
- F. Wang, X.-C. Li, W.-Y. Lai, Y. Chen, W. Huang and F. Wudl, Org. Lett., 2014, 16, 2942–2945 Search PubMed.
- T. Bura, N. Leclerc, S. Fall, P. Lévêque, T. Heiser and R. Ziessel, Org. Lett., 2011, 13, 6030–6033 Search PubMed.
- T. Bura, N. Leclerc, R. Bechara, P. Lévêque, T. Heiser and R. Ziessel, Adv. Energy Mater., 2013, 3, 1118–1124 Search PubMed.
- N. Robertson, S. Parsons, E. J. MacLean, R. A. Coxall and A. R. Mount, J. Mater. Chem., 2000, 10, 2043–2047 Search PubMed.
- B. Gómez-Lor and A. M. Echavarren, Org. Lett., 2004, 6, 2993–2996 Search PubMed.
- R. A. Valentine, A. Whyte, K. Awaga and N. Robertson, Tetrahedron Lett., 2012, 53, 657–660 Search PubMed.
- N. Toworakajohnkun, M. Sukwattanasinitt and P. Rashatasakhon, Tetrahedron Lett., 2017, 58, 4149–4152 Search PubMed.
- L. Rong, Q. Liu, Y. Shi and J. Tang, Chem. Commun., 2011, 47, 2155–2157 Search PubMed.
- A. E. Sadak, A. C. Gören, Ö. A. Bozdemir and N. Saraçoğlu, ChemistrySelect, 2017, 2, 10512–10516 Search PubMed.
- Q. Huaulmé, E. Cece, A. Mirloup and R. Ziessel, Tetrahedron Lett., 2014, 55, 4953–4958 Search PubMed.
- W.-Y. Lai, Q.-Y. He, R. Zhu, Q.-Q. Chen and W. Huang, Adv. Funct. Mater., 2008, 18, 265–276 Search PubMed.
- A. Babaei, K. Rakstys, S. Guelen, V. Fallah Hamidabadi, M.-G. La-Placa, L. Martínez-Sarti, M. Sessolo, H. A. Joel, O. P. M. Gaudin, V. Schanen, M. K. Nazeeruddin and H. J. Bolink, RSC Adv., 2018, 8, 35719–35723 Search PubMed.
- B. Zhao, B. Liu, R. Q. Png, K. Zhang, K. A. Lim, J. Luo, J. Shao, P. K. H. Ho, C. Chi and J. Wu, Chem. Mater., 2010, 22, 435–449 Search PubMed.
- G.-L. Feng, W.-Y. Lai, S.-J. Ji and W. Huang, Tetrahedron Lett., 2006, 47, 7089–7092 Search PubMed.
- W.-Y. Lai, Q.-Y. He, D.-Y. Chen and W. Huang, Chem. Lett., 2008, 37, 986–987 Search PubMed.
- W.-Y. Lai, R. Zhu, Q.-L. Fan, L.-T. Hou, Y. Cao and W. Huang, Macromolecules, 2006, 39, 3707–3709 Search PubMed.
- M. Sang, S. Cao, J. Yi, J. Huang, W.-Y. Lai and W. Huang, RSC Adv., 2016, 6, 6266–6275 Search PubMed.
- Z. Yao, X. Liao, Y. Guo, H. Zhao, Y. Guo, F. Zhang, L. Zhang, Z. Zhu, L. Kloo, W. Ma, Y. Chen and L. Sun, Chem. Mater., 2019, 31, 8810–8819 Search PubMed.
- B. Gómez-Lor, B. Alonso, A. Omenat and J. L. Serrano, Chem. Commun., 2006, 5012–5014 Search PubMed.
-
E. M. García-Frutos and B. Gómez-Lor, Organic Field-Effect Transistors VIII, Proc. of SPIE, 2009, vol. 7417, pp. 74170J1 Search PubMed.
- J. Luo, B. Zhao, J. Shao, K. A. Lim, H. S. On Chan and C. Chi, J. Mater. Chem., 2009, 19, 8327–8334 Search PubMed.
- Y.-C. Hu, Z.-L. Lin, T.-C. Huang, J.-W. Lee, W.-C. Wei, T.-Y. Ko, C.-Y. Lo, D.-G. Chen, P.-T. Chou, W.-Y. Hung and K.-T. Wong, Mater. Chem. Front., 2020, 4, 2029–2039 Search PubMed.
- S. Zhang, W. Chen, G. Dai, F. Yang and L. Chen, Asian J. Org. Chem., 2018, 7, 2223–2227 Search PubMed.
- B. Gómez-Lor, G. Hennrich, B. Alonso, M. Á. Monge, E. Gutiérrez-Puebla and A. M. Echavarren, Angew. Chem., Int. Ed., 2006, 45, 4491–4494 Search PubMed.
- E. M. García-Frutos, B. Gómez-Lor, M. Á. Monge, E. Gutiérrez-Puebla, I. Alkorta and J. Elguero, Chem. – Eur. J., 2008, 14, 8555–8561 Search PubMed.
- Y. Chen, X. Wu, Y. Liu, L. Chen, H. Li, W. Wang, S. Wang, H. Tian, H. Tong and L. Wang, J. Mater. Chem. C, 2019, 7, 7900–7905 Search PubMed.
- G. Otero, G. Biddau, C. Sánchez-Sánchez, R. Caillard, M. F. López, C. Rogero, F. J. Palomares, N. Cabello, M. A. Basanta, J. Ortega, J. Méndez, A. M. Echavarren, R. Pérez, B. Gómez-Lor and J. A. Martín-Gago, Nature, 2008, 454, 865–868 Search PubMed.
- C. Ruiz, E. M. García-Frutos, D. A. da Silva Filho, J. T. López Navarrete, M. C. Ruiz Delgado and B. Gómez-Lor, J. Phys. Chem. C, 2014, 118, 5470–5477 Search PubMed.
- E. M. García-Frutos, E. Gutierrez-Puebla, M. A. Monge, R. Ramírez, P. de Andrés, A. de Andrés, R. Ramírez and B. Gómez-Lor, Org. Electron., 2009, 10, 643–652 Search PubMed.
- F. Gallego-Gómez, E. M. García-Frutos, J. M. Villalvilla, J. A. Quintana, E. Gutiérrez-Puebla, M. Á. Monge, M. A. Díaz-García and B. Gómez-Lor, Adv. Funct. Mater., 2011, 21, 738–745 Search PubMed.
- T. Han, I. Bulut, S. Méry, B. Heinrich, P. Lévêque, N. Leclerc and T. Heiser, J. Mater. Chem. C, 2017, 5, 10794–10800 Search PubMed.
- A. Benito-Hernández, U. K. Pandey, E. Cavero, R. Termine, E. M. García-Frutos, J. L. Serrano, A. Golemme and B. Gómez-Lor, Chem. Mater., 2013, 25, 117–121 Search PubMed.
- Z. Li, S. Liu, Q. Zhang and D. Liu, Integr. Ferroelectr., 2013, 144, 112–117 Search PubMed.
- F. Zhao, C. Liu, Y. Sun, Q. Li, J. Zhao, Z. Li, B. Zhang, C. Lu, Q. Li, S. Qiao and Y. S. Zhao, Org. Electron., 2019, 74, 276–281 Search PubMed.
- M. Reig, J. Puigdollers and D. Velasco, J. Mater. Chem. C, 2015, 3, 506–513 Search PubMed.
- M. Reig, G. Bagdziunas, A. Ramanavicius, J. Puigdollers and D. Velasco, Phys. Chem. Chem. Phys., 2018, 20, 17889–17898 Search PubMed.
- C. Li, Y. Wang, Y. Zou, X. Zhang, H. Dong and W. Hu, Angew. Chem., Int. Ed., 2020, 59, 9403–9407 Search PubMed.
- S. W. Shelton, T. L. Chen, D. E. Barclay and B. Ma, ACS Appl. Mater. Interfaces, 2012, 4, 2534–2540 Search PubMed.
- C. Kulshreshtha, G. W. Kim, R. Lampande, D. H. Huh, M. Chae and J. H. Kwon, J. Mater. Chem. A, 2013, 1, 4077–4082 Search PubMed.
- F. Zhang, X. Yang, M. Cheng, J. Li, W. Wang, H. Wang and L. Sun, J. Mater. Chem. A, 2015, 3, 24272–24280 Search PubMed.
- L. Calió, C. Momblona, L. Gil-Escrig, S. Kazim, M. Sessolo, Á. Sastre-Santos, H. J. Bolink and S. Ahmad, Sol. Energy Mater. Sol. Cells, 2017, 163, 237–241 Search PubMed.
- D. R. Kil, C. Lu, J.-M. Ji, C. H. Kim and H. K. Kim, Nanomaterials, 2020, 10, 936 Search PubMed.
- T. Techajaroonjit, S. Namuangruk, N. Prachumrak, V. Promarak, M. Sukwattanasinitt and P. Rashatasakhon, RSC Adv., 2016, 6, 56392–56398 Search PubMed.
- Q. Ye, J. Chang, J. Shao and C. Chi, J. Mater. Chem., 2012, 22, 13180 Search PubMed.
- L. A. Illicachi, J. Urieta-Mora, J. Calbo, J. Aragó, C. Igci, I. García-Benito, C. Momblona, B. Insuasty, A. Ortiz, C. Roldán-Carmona, A. Molina-Ontoria, E. Ortí, N. Martín and M. K. Nazeeruddin, Chem. – Eur. J., 2020, 26, 11039–11047 Search PubMed.
- F. J. Ramos, K. Rakstys, S. Kazim, M. Grätzel, M. K. Nazeeruddin and S. Ahmad, RSC Adv., 2015, 5, 53426–53432 Search PubMed.
- K. Rakstys, A. Abate, M. I. Dar, P. Gao, V. Jankauskas, G. Jacopin, E. Kamarauskas, S. Kazim, S. Ahmad, M. Grätzel and M. K. Nazeeruddin, J. Am. Chem. Soc., 2015, 137, 16172–16178 Search PubMed.
- I. Petrikyte, I. Zimmermann, K. Rakstys, M. Daskeviciene, T. Malinauskas, V. Jankauskas, V. Getautis and M. K. Nazeeruddin, Nanoscale, 2016, 8, 8530–8535 Search PubMed.
- A. Connell, Z. Wang, Y.-H. Lin, P. C. Greenwood, A. A. Wiles, E. W. Jones, L. Furnell, R. Anthony, C. P. Kershaw, G. Cooke, H. J. Snaith and P. J. Holliman, J. Mater. Chem. C, 2019, 7, 5235–5243 Search PubMed.
- P.-Y. Su, L.-B. Huang, J.-M. Liu, Y.-F. Chen, L.-M. Xiao, D.-B. Kuang, M. Mayor and C.-Y. Su, J. Mater. Chem. A, 2017, 5, 1913–1918 Search PubMed.
- L. Shen, X. Yang, J. An, L. Zhang, K. Yang and Z. Deng, ChemistrySelect, 2021, 6, 4645–4650 Search PubMed.
- X. Qian, Y.-Z. Zhu, J. Song, X.-P. Gao and J.-Y. Zheng, Org. Lett., 2013, 15, 6034–6037 Search PubMed.
- X. Qian, L. Lu, Y.-Z. Zhu, H.-H. Gao and J.-Y. Zheng, Dyes Pigm., 2015, 113, 737–742 Search PubMed.
- P. Qin, P. Sanghyun, M. I. Dar, K. Rakstys, H. ElBatal, S. A. Al-Muhtaseb, C. Ludwig and M. K. Nazeeruddin, Adv. Funct. Mater., 2016, 26, 5550–5559 Search PubMed.
- K. Rakstys, S. Paek, P. Gao, P. Gratia, T. Marszalek, G. Grancini, K. T. Cho, K. Genevicius, V. Jankauskas, W. Pisula and M. K. Nazeeruddin, J. Mater. Chem. A, 2017, 5, 7811–7815 Search PubMed.
- R. R. Reghu, D. Volyniuk, N. Kostiv, K. Norvaisa and J. V. Grazulevicius, Dyes Pigm., 2016, 125, 159–168 Search PubMed.
- Z.-L. Zhang, L.-Y. Zou, A.-M. Ren, Y.-F. Liu, J.-K. Feng and C.-C. Sun, Dyes Pigm., 2013, 96, 349–363 Search PubMed.
- Y. Liu, X. Wu, Y. Chen, L. Chen, H. Li, W. Wang, S. Wang, H. Tian, H. Tong and L. Wang, J. Mater. Chem. C, 2019, 7, 9719–9725 Search PubMed.
- Y. Chen, S. Wang, X. Wu, Y. Xu, H. Li, Y. Liu, H. Tong and L. Wang, J. Mater. Chem. C, 2018, 6, 12503–12508 Search PubMed.
- K. J. Kim, G. H. Kim, R. Lampande, D. H. Ahn, J. B. Im, J. S. Moon, J. K. Lee, J. Y. Lee, J. Y. Lee and J. H. Kwon, J. Mater. Chem. C, 2018, 6, 1343–1348 Search PubMed.
- S. J. Yoon, J. H. Kim, W. J. Chung and Y. J. Lee, Chem. – Eur. J., 2021, 27, 3065–3073 Search PubMed.
- J. Eng, J. Hagon and T. J. Penfold, J. Mater. Chem. C, 2019, 7, 12942–12952 Search PubMed.
- L. Ji, Q. Fang, M.-S. Yuan, Z.-Q. Liu, Y.-X. Shen and H.-F. Chen, Org. Lett., 2010, 12, 5192–5195 Search PubMed.
- J. Zhang, L. Gong, X. Zhang, M. Zhu, C. Su, Q. Ma, D. Qi, Y. Bian, H. Du and J. Jiang, Chem. – Eur. J., 2020, 26, 13842–13848 Search PubMed.
- Y.-F. Xie, S.-Y. Ding, J.-M. Liu, W. Wang and Q.-Y. Zheng, J. Mater. Chem. C, 2015, 3, 10066–10069 Search PubMed.
- A. Alkaş, J. Cornelio and S. G. Telfer, Chem. – Asian J., 2018, 14, 1167–1174 Search PubMed.
- A. Alkas and S. G. Telfer, Aust. J. Chem., 2019, 72, 786–796 Search PubMed.
- W. Lai, D. Liu and W. Huang, Sci. China: Chem., 2010, 53, 2472–2480 Search PubMed.
- X.-C. Li, Y. Zhang, C.-Y. Wang, Y. Wan, W.-Y. Lai, H. Pang and W. Huang, Chem. Sci., 2017, 8, 2959–2965 Search PubMed.
- A. E. Sadak, E. Karakuş, Y. M. Chumakov, N. A. Dogan and C. T. Yavuz, ACS Appl. Energy Mater., 2020, 3, 4983–4994 Search PubMed.
- Y. Xu, X. Wu, Y. Chen, H. Hang, H. Tong and L. Wang, RSC Adv., 2016, 6, 31915–31918 Search PubMed.
- Y. Xu, X. Wu, Y. Chen, H. Hang, H. Tong and L. Wang, Polym. Chem., 2016, 7, 4542–4548 Search PubMed.
- X. Li, C. Wang, W. Song, C. Meng, C. Zuo, Y. Xue, W. Lai and W. Huang, ChemPlusChem, 2019, 84, 1623–1629 Search PubMed.
- X.-C. Li, C.-Y. Wang, Y. Wan, W.-Y. Lai, L. Zhao, M.-F. Yin and W. Huang, Chem. Commun., 2016, 52, 2748–2751 Search PubMed.
- Y. Xu, H. Li, X. Wu, Y. Chen, H. Hang, H. Tong and L. Wang, Polym. Chem., 2017, 8, 2484–2489 Search PubMed.
- E. Andrikaityte, J. Simokaitiene, A. Tomkeviciene, J. V. Grazulevicius and V. Jankauskas, Mol.
Cryst. Liq. Cryst., 2014, 590, 121–129 Search PubMed.
- N. Li, Y. Chen, S. Duan, G. Chen, Y. Xu, H. Tong, Y. Sanehira, T. Miyasaka, A. Li and X.-F. Wang, J. Photochem. Photobiol., A, 2020, 389, 112228 Search PubMed.
- G. Hu, S. P. Kitney, S. M. Kelly, W. Harrison, B. Lambert and M. O’Neill, RSC Adv., 2018, 8, 8580–8585 Search PubMed.
- Q. Wang, R. Li, X. Ouyang and G. Wang, RSC Adv., 2019, 9, 40531–40535 Search PubMed.
- D. Zhang, T. K. Ronson, J. L. Greenfield, T. Brotin, P. Berthault, E. Léonce, J.-L. Zhu, L. Xu and J. R. Nitschke, J. Am. Chem. Soc., 2019, 141, 8339–8345 Search PubMed.
- D. Zhang, T. K. Ronson, S. Güryel, J. D. Thoburn, D. J. Wales and J. R. Nitschke, J. Am. Chem. Soc., 2019, 141, 14534–14538 Search PubMed.
- A. E. Sadak and E. Karakuş, J. Fluoresc., 2020, 30, 213–220 Search PubMed.
- N. N. Ghosh, M. Habib, A. Pramanik, P. Sarkar and S. Pal, New J. Chem., 2019, 43, 6480–6491 Search PubMed.
- K.-R. Wang, H.-W. An, D. Han, F. Qian and X.-L. Li, Chin. Chem. Lett., 2013, 24, 467–470 Search PubMed.
- M. Franceschin, L. Ginnari-Satriani, A. Alvino, G. Ortaggi and A. Bianco, Eur. J. Org. Chem., 2010, 134–141 Search PubMed.
- A. Cummaro, I. Fotticchia, M. Franceschin, C. Giancola and L. Petraccone, Biochimie, 2011, 93, 1392–1400 Search PubMed.
- L. Petraccone, I. Fotticchia, A. Cummaro, B. Pagano, L. Ginnari-Satriani, S. Haider, A. Randazzo, E. Novellino, S. Neidle and C. Giancola, Biochimie, 2011, 93, 1318–1327 Search PubMed.
- T. Kojima, S. Furukawa, H. Tsuji and E. Nakamura, Chem. Lett., 2014, 43, 676–677 Search PubMed.
- D. Christian, T. H. Nguyen, M. Lonka and M. Jacques, Mol. Cryst. Liq. Cryst., 1984, 114, 139–150 Search PubMed.
- J. Bergman and B. Egestad, Chem. Scr., 1986, 26, 287–292 Search PubMed.
-
JP 08146221 A, 1996 Search PubMed.
-
JP 10324661 A, 1998 Search PubMed.
- H. Kaida, T. Satoh, Y. Nishii, K. Hirano and M. Miura, Chem. Lett., 2016, 45, 1069–1071 Search PubMed.
- S. Nakamura, M. Okamoto, N. Tohnai, K. Nakayama, Y. Nishii and M. Miura, Bull. Chem. Soc. Jpn., 2020, 93, 99–108 Search PubMed.
-
JP 11092427 A, 1999, 1–17 Search PubMed.
- T. H. Nguyen, R. Cayuela, C. Destrade and J. Malthete, Mol. Cryst. Liq. Cryst., 1985, 122, 141–149 Search PubMed.
- J. Bergman and B. Egestad, Tetrahedron, 1986, 42, 763–773 Search PubMed.
- R. Cayuela, T. H. Nguyen, C. Destrade and A. M. Levelut, Mol. Cryst. Liq. Cryst., 1989, 177, 81–91 Search PubMed.
-
US 5637669 A, 1997 Search PubMed.
-
KR 20140000611 A, 2014 Search PubMed.
-
WO 201411400 7A1, 2014 Search PubMed.
-
KR 20110005476 A, 2011 Search PubMed.
-
US 2016/0233427 A1, 2016 Search PubMed.
- Q. Xiao, T. Sakurai, T. Fukino, K. Akaike, Y. Honsho, A. Saeki, S. Seki, K. Kato, M. Takata and T. Aida, J. Am. Chem. Soc., 2013, 135, 18268–18271 Search PubMed.
- S. Limberti, L. Emmett, A. Trandafir, G. Kociok-Köhn and G. D. Pantoş, Chem. Sci., 2019, 10, 9565–9570 Search PubMed.
- K. Górski, J. Mech-Piskorz, K. Noworyta, B. Leśniewska and M. Pietraszkiewicz, New J. Chem., 2018, 42, 5844–5852 Search PubMed.
- K. Górski, J. Mech-Piskorz, B. Leśniewska, O. Pietraszkiewicz and M. Pietraszkiewicz, J. Org. Chem., 2019, 84, 11553–11561 Search PubMed.
- M. R. Maciejczyk, J. A. G. Williams, N. Robertson and M. Pietraszkiewicz, RSC Adv., 2017, 7, 49532–49535 Search PubMed.
- K. Górski, J. Mech-Piskorz, B. Leśniewska, O. Pietraszkiewicz and M. Pietraszkiewicz, J. Org. Chem., 2020, 85, 4672–4681 Search PubMed.
- M. T. El Sayed, L. Wessjohann, A. Porzel and A. Hilgeroth, J. Heterocycl. Chem., 2016, 54, 1077–1083 Search PubMed.
- A. Benito-Hernández, M. T. El Sayed, J. T. López Navarrete, M. C. Ruiz Delgado and B. Gómez-Lor, Org. Chem. Front., 2018, 5, 1748–1755 Search PubMed.
- M. T. El Sayed, S. N. Shabaan, A. E. Sarhan, S. M. El-Messery, S. M. El-Sayed and G. S. Hassan, Bioorg. Chem., 2020, 104, 104323 Search PubMed.
- K. Kobayashi, N. Suzue, R. Ishii, R. Kamiya and H. Wakabayashi, Heterocycles, 2009, 78, 2467–2475 Search PubMed.
|
This journal is © The Royal Society of Chemistry and the Centre National de la Recherche Scientifique 2022 |
Click here to see how this site uses Cookies. View our privacy policy here.