DOI:
10.1039/D2OB01509A
(Communication)
Org. Biomol. Chem., 2022,
20, 9593-9599
Divergent cyclodimerizations of styrylnaphthols under aerobic visible-light irradiation and Brønsted acid catalysis†
Received
23rd August 2022
, Accepted 8th November 2022
First published on 11th November 2022
Abstract
Dimeric cyclization reactions show great potential to rapidly form highly substituted complex cyclic molecules from simple starting materials. However, such an appealing process is often hampered by the lack of selectivity. Herein we report two divergent cyclodimerization reactions of 1-styrylnaphthalen-2-ol derivatives under simple and very mild reaction conditions. A stereoselective visible light-induced oxidative (1 + 1 + 4 + 4) homodimerization gave rise to highly substituted 1,5-dioxocanes in moderate yields. This transformation harnessed singlet oxygen as a safe and mild oxidant under photocatalyst-free reaction conditions. Additionally, we demonstrated that the same substrates undergo a (4 + 2) heterodimerization under Brønsted-acid catalysis to produce chromane derivatives featuring 3 contiguous tertiary stereocenters in good to high yields with excellent diastereoselectivities.
Introduction
The development of efficient divergent synthetic methods for the construction of structurally diverse molecules from common, readily accessible starting materials is of great interest to the synthetic community.1 In addition, the cyclodimerizations of olefins are one of the most attractive approaches to rapidly access divergent complex cyclic frameworks. For instance, these processes are often proposed as biosynthetic pathways leading to a variety of biologically active natural homodimers and heterodimers.2,3 Furthermore, they are valuable synthetic methods for the synthesis of structurally appealing synthetic complex cyclic dimeric molecules.4 Stilbene derivatives are relevant compounds in cyclodimerizations. However, their reactions often result in the formation of mixtures of compounds due to their high reactivity and tendency to oligomerize. For example, the oligomerization of resveratrol (trihydroxystilbene) and its natural analogues provided more than one hundred architecturally-diverse biologically-active natural oligomeric products.5 Generally, a biomimetic synthetic approach based on one-electron oxidative dimerization of resveratrol derivatives led to low yields6 and/or a complex mixture of products.7,8 Similar difficulties have also been encountered during the synthesis of synthetic dimeric stilbenes.9 For instance, Faizal Weber et al. have revealed that the oxidative dimerization of resveratrol analogues was dependent on the choice of solvent and a metal oxidant providing diverse synthetic dimeric compounds in low yields.9a Similarly, Kam et al. have demonstrated that the electrochemical oxidative dimerization of 1,2-diarylethylenes furnished oligomers featuring varied carbon skeletons depending on the aromatic substitution.9b As such, the dimerization of 1,2-diarylethylene derivatives into a single dimeric product is still a challenging issue. In this communication, we report unexpected stereoselective and divergent cyclodimerizations of styrylnaphthols that selectively produce two different complex O-containing heterocyclic compounds depending on the conditions used. The first photoinduced procedure harnessed molecular oxygen as an abundant, green, and safe oxidant to promote a highly stereoselective (1 + 1 + 4 + 4) homodimerization reaction affording 1,5-dioxocanes. The second protocol employed a simple Brønsted acid to catalyze an unprecedented (4 + 2) heterodimerization leading to relevant chromane derivatives featuring three contiguous stereocenters in good to excellent yields and in a diastereomerically pure form.
Results and discussion
As part of our continuing interest in the construction of cyclic compounds through either photocatalysis10 or Brønsted acid catalysis,11 we initially envisioned the catalytic enantioselective synthesis of polysubstituted chromane 3avia the reaction of 1-styrylnaphthalen-2-ol 1a with 2-hydroxybenzylthioether 2 using our recently designed chimeric photo-Brønsted acid catalyst PC (Scheme 1a).12 Our original working hypothesis relied on the oxidative photocatalytic C–S bond cleavage of 2a10c,13 to in situ generate ortho-quinone methide 4 which could further undergo a Brønsted acid-catalyzed oxa-Diels Alder type reaction with 1a.14 Surprisingly, under visible light irradiation (405 nm) and an oxygen atmosphere in dichloromethane, 3a did not form and only the diastereomerically pure product 5a was isolated in 32% yield (Scheme 1b). Its structure was established to be a homodimer having an interesting dioxo-eight-membered ring, i.e. 1,5-dioxocane, as the central motif. Such a framework has been found in several naturally occurring products such as Luisol A15 and withametelinol-A and B16 or in bioactive compounds like various penicillide derivatives.17 Recently, Zhou and Chen et al. reported an efficient synthesis of these complex products 5 through a copper-catalyzed oxidative (1 + 1 + 4 + 4) dimerization of 1-styrylnaphthols using a stoichiometric amount of Selectfluor as the terminal oxidant.18 To our knowledge, such photoinduced aerobic oxidative (1 + 1 + 4 + 4) homodimerization of 1-styrylnaphthalen-2-ol derivatives has not been reported. This, together with the mild conditions and operational simplicity, prompted us to examine this new homodimerization methodology in more detail.
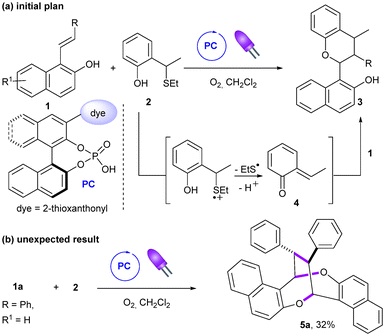 |
| Scheme 1 Serendipitous discoveries on the dimerization of styrylnaphthols. | |
Initial control experiments revealed that the photocatalyst PC was not required, although irradiation at 405 nm and the presence of oxygen were both essential for this homodimerization (Table 1, entries 1–4). Pleasingly, shortening the reaction time from 10 to 6 hours improved the chemical yield to 50% (entry 5). This result indicates a slight decomposition of the product 5a over time under the reaction conditions and that the reaction progress needs to be carefully monitored. However, a shorter reaction time (4 hours) led to a lower yield of 5a, along with the formation of (Z)-1a (entry 6). This experiment shows a partial photoisomerization of the E-isomer to the Z-isomer19 and that product 5a can also be obtained from (Z)-1a (entry 6 vs. entry 5).20 The concentration of the reaction mixture had a significant impact on the yields. A more concentrated or diluted reaction mixture decreased the yield (entries 7 and 8). Screening of solvents showed that dichloromethane was optimal (entries 9–12). To further improve the yield, various additives were also investigated (entries 13–15). A lower yield was obtained in the presence of an inorganic base (entry 13). Addition of two equivalents of triethylamine totally suppressed the homodimerization reaction and the isomer (Z)-1a was predominantly formed instead (entry 14). To our surprise, when the reaction was conducted with two equivalents of p-toluenesulfonic acid (TsOH), we isolated chromane derivative 6a in 15% yield in addition to the desired homodimer 5a (17%, entry 15). Finally, it was found that the homodimerization reaction could be simply conducted under air instead of a balloon of oxygen (entry 16).
Table 1 Optimization of the parameters for the (1 + 1 + 4 + 4) homodimerization of 1aa
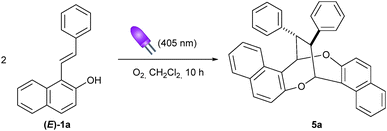
|
Entry |
Deviation/additive (equiv.) |
RSMb,c |
5a
|
(Z)-1a
|
Reaction conditions: 1a (0.1 mmol), CH2Cl2 (0.07 M), O2, 405 nm, rt (internal temperature: 28 °C).
Recovered starting material.
NMR yield using 1,2-dibromoethane as an internal standard.
17% of 5a along with 15% of 6a were obtained.
Base additive was added to facilitate oxidation via the deprotonation of the phenol.
Acid additive was added to promote the cycloaddition step.
|
1 |
With PC (0.1) |
0% |
32% |
0% |
2 |
None |
0 |
31% |
0% |
3 |
Ar instead of O2 |
100% |
0% |
0% |
4 |
420 nm instead of 405 nm |
100% |
0% |
0% |
5 |
6 h instead of 10 h |
0% |
50% |
0% |
6 |
4 h instead of 10 h |
0% |
38% |
27% |
7 |
0.03 M instead of 0.07 M |
16% |
23% |
28% |
8 |
0.13 M instead of 0.07 M |
0% |
33% |
14% |
9 |
Entry 5 in toluene |
20% |
22% |
28% |
10 |
Entry 5 in dioxane |
16% |
5% |
74% |
11 |
Entry 5 in DMSO |
0% |
5% |
60% |
12 |
Entry 5 in EtOH |
0% |
35% |
0% |
13 |
Entry 5, with Na2CO3 (2)e |
18% |
26% |
35% |
14 |
Entry 5, with Et3N (2)e |
12% |
0% |
88% |
15 |
Entry 5, with TsOH (2)f |
0% |
17%d |
0% |
16 |
Entry 5, air instead of O2 |
0% |
50% |
0% |
Having established the suitable reaction conditions for the oxidative homodimerization of 1-styrylnaphthalen-2-ol derivatives 1, we next examined the substrate scope (Table 2). In all the cases, the desired 1,5-dioxocine derivatives 5 were obtained in moderate yields with excellent diastereoselectivities. Phenyl rings with either electron-withdrawing or electron-donating groups at the para position were compatible to our reaction conditions (5b–5e). As observed by Zhou and Chen et al.,18 a lower yield was nevertheless obtained with a strong electron-withdrawing substituent (5b). Notably, ortho-substituted phenyl rings were well tolerated furnishing the corresponding homodimer in up to 58% yield (5g and 5h, 5l and 5m). In addition, substrates having a bromo-substituent at either the 3- or 6-positions of the naphthalene ring were also suitable (5i–5m). Unfortunately, the reaction did not proceed from styrylphenol.21 Finally, the homodimerization of 1a was conducted on a 1 mmol scale affording the corresponding cyclic compound 5a in similar yields (46%).
Table 2 Scope of the photomediated aerobic oxidative homodimerization of styrylnaphthol derivatives 1a,b
Reaction conditions: 1 (0.1 mmol), CH2Cl2 (0.07 M), O2, 405 nm, 6 h, rt (internal temperature: 28 °C).
Isolated yield.
Reaction conducted on a 1 mmol scale.
|
|
The unexpected formation of chromane 6a in the presence of an acid inspired us to investigate its formation in detail. The exact structure of 6 was established to be a chromane scaffold containing three contiguous stereocenters in trans–trans configuration. Although chromanes are privileged heterocyclic compounds in medicinal chemistry, this scaffold 6 is unprecedented. Therefore, we decided to study the synthesis of novel chromanes via the (4 + 2) heterodimerization reaction of styrylnaphthols. Close examination of the reaction showed that light irradiation was not necessary for heterodimerization and the desired product 6a was obtained with encouraging 40% yield (Table 3, entry 2). Interestingly, the reaction could be carried out with a catalytic amount (10 mol%) of acid (entry 3). Gratefully, variation of the reaction temperature (entries 4 and 5) resulted in a dramatic improvement in the yield (85%) at 50 °C. Rapid screening of acid catalysts led to the decomposition of the starting material with either a stronger Brønsted acid catalyst (triflic acid, entry 6) or a Lewis acid catalyst (BF3·OEt2, entry 7), while the starting material was totally recovered with a weaker Brønsted acid catalyst (trifluoroacetic acid, entry 8). To our delight, a final variation of the solvent (entries 9–11) revealed that toluene was optimal for delivering the desired heterodimer adduct in 99% isolated yield.22
Table 3 Optimization of the parameters for the (4 + 2) heterodimerization of 1aa
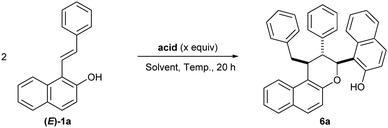
|
Entry |
Acid (equiv.) |
Solvent |
Temp. |
6a
,
|
Reaction conditions: 1a (0.1 mmol), acid (x equiv.), solvent (0.07 M), 20 h.
NMR yield using 1,2-dibromoethane as an internal standard; isolated between brackets.
The structure and relative configurations were established by analogy with the X-ray crystal structure analysis of 6b (CCDC 2082935, see the ESI†).
6 h instead of 20 h.
Strict argon atmosphere.
|
1d |
TsOH (2) |
CH2Cl2 |
rt |
15% |
2 |
TsOH (2) |
CH2Cl2 |
rt |
40% |
3 |
TsOH (0.1) |
CH2Cl2 |
rt |
30% |
4 |
TsOH (0.1) |
DCE |
50 °C |
85% |
5 |
TsOH (0.1) |
DCE |
70 °C |
85% |
6 |
CF3SO3H (0.1) |
DCE |
50 °C |
0% |
7 |
BF3·OEt2 (0.1) |
DCE |
50 °C |
0% |
8 |
TFA (0.1) |
DCE |
50 °C |
0% |
9 |
TsOH (0.1) |
THF |
50 °C |
24% |
10 |
TsOH (0.1) |
CH3CN |
50 °C |
23% |
11 |
TsOH (0.1) |
Toluene |
50 °C |
100% (99%) |
12 |
— |
DCE |
50 °C |
0% |
13e |
TsOH (0.1) |
DCE |
50 °C |
85% |
With the best reaction conditions in hand, the scope of this catalytic heterodimerization reaction was explored as depicted in Table 4. Substrates bearing various substituents on either the phenyl or the naphthyl rings were tested affording in all cases the corresponding trisubstituted chromane derivative as a single diastereoisomer. Good to excellent yields were achieved with substrates having an electron-withdrawing group on the phenyl ring (6b–6d, 6h–6k, 6m–6o, 64–94%). Cycloadducts 6e and 6f substituted with an electron donating group (methoxy and tert-butyl respectively) were obtained with slightly lower yields (32–60%). It is worth mentioning that substrates with ortho- as well as meta-substituted phenyl rings were also well tolerated furnishing the corresponding heterodimers (6g–6k) in high to excellent yields (75–98%). The heterodimerization of 6-bromo-1-styrylnaphthalen-2-ol derivatives also proceeded smoothly, providing products 6l–6o in 43–94% yields. Nevertheless, styrylphenol was unreactive under these reaction conditions.23 Finally, heterodimer 6a was produced in 61% yield when the reaction was carried out on a 1 mmol scale.
Table 4 Scope of the Brønsted acid-catalyzed heterodimerization of styrylnaphthol derivatives 1a,b
Reaction conditions: 1 (0.1 mmol), toluene (0.07 M), p-TsOH (0.01 mmol), 20 h.
Isolated yield.
Reaction conducted on a 1 mmol scale.
DCE instead of toluene.
|
|
Finally, we focused our attention on the mechanism of these dimerizations and control experiments were conducted. For the visible-light mediated aerobic oxidative homodimerization reaction, the addition of 2,2,6,6-tetramethylpiperidine-1-oxyl (TEMPO) to the standard reaction conditions totally inhibited the formation of 5a, which supported a radical mechanism. Moreover, in the presence of the known singlet oxygen quencher 1,4-diazabicycle[2.2.2]octane (DABCO),24 the yield significantly decreased suggesting the involvement of singlet oxygen in the process (Scheme 2). Additionally, ultraviolet–visible (UV-vis) spectroscopic analysis of 1a unveiled an overlap between the absorption spectrum of 1a and the emission spectrum of the purple light.25 For the (4 + 2) heterodimerization reaction, no reaction occurred in the absence of TsOH demonstrating the crucial role of the Brønsted acid catalyst (Table 3, entry 12). However, no significant effect on the reaction outcome was observed under a strict argon atmosphere showing that no special precaution was required (Table 3, entry 13 vs. 4).
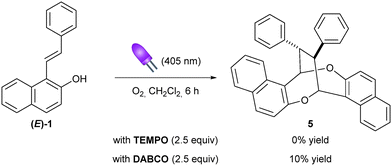 |
| Scheme 2 Control experiments. | |
On the basis of these observations and literature reports, plausible mechanisms are depicted in Scheme 3. Upon irradiation at 405 nm, 1 is converted into a high-energy excited singlet 11*, which undergoes intersystem crossing (ISC) to give triplet 31*. Intermolecular energy transfer to molecular oxygen generates the highly oxidant singlet oxygen species. Subsequent single electron transfer to 1 produces a superoxide radical anion O2˙− along with radical cation 7. Further deprotonation would form the key persistent quinone methyl radical 8. According to the works of Zhou and Chen et al., the latter selectively dimerizes into bis-quinone methide 9. Further intramolecular [4 + 4] cycloaddition eventually delivered 1,5-dioxocane 5. As for the Brønsted acid-catalyzed heterodimerization, 1 would tautomerize into ortho-quinone methide 10 in the presence of p-TsOH. Subsequent endo-selective intermolecular inverse electron demanding oxa-Diels Alder reaction with 1 furnishes trisubstituted chromanes 6 in trans–trans configurations.
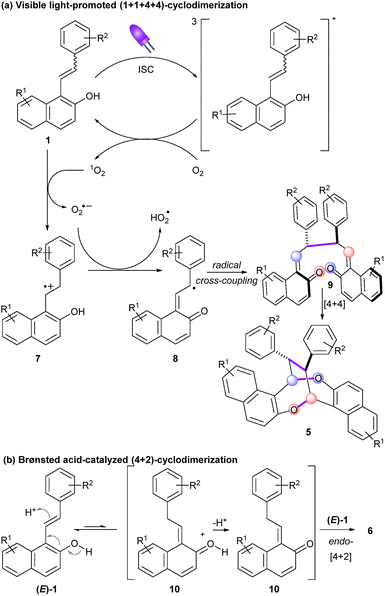 |
| Scheme 3 Proposed mechanisms. | |
Conclusions
In conclusion, we have developed two cyclodimerizations of 1-styrylnaphtol derivatives under environmentally benign reaction conditions. While a (1 + 1 + 4 + 4) cyclodimerization was observed under aerobic visible light irradiation, a (4 + 2) cycloaddition occurred in the presence of a catalytic amount of Brønsted acid. These transformations allowed the diastereoselective construction of highly substituted 1,5-dioxocanes and chromane derivatives featuring 3 contiguous tertiary stereocenters in moderate yields. Further use of styrylnaphthol derivatives for the construction of heterocyclic compounds is under investigation in our laboratory.
Conflicts of interest
There are no conflicts to declare.
Acknowledgements
We gratefully acknowledge CNRS, ICSN for financial support. J. L. thanks the China Scholarship Council for the doctoral fellowship. Arnaud Chevalier is warmly thanked for the UV-vis spectroscopic measurement of 1a.
Notes and references
-
(a) S. L. Schreiber, Science, 2000, 287, 1964 CrossRef CAS PubMed;
(b) M. D. Burke and S. L. Schreiber, Angew. Chem., Int. Ed., 2004, 43, 46 CrossRef PubMed;
(c) E. Lenci, A. Guarna and A. Trabocchi, Molecules, 2014, 19, 16506 CrossRef PubMed.
- Reviews:
(a) S. A. Snyder, A. M. ElSohlya and F. Kontesa, Nat. Prod. Rep., 2011, 28, 897 RSC;
(b) J. Sun and W. Tang, Chem. Soc. Rev., 2021, 50, 2320 RSC; selected examples.
(c) A. Sib and T. A. M. Gulder, Angew. Chem., Int. Ed., 2017, 56, 12888 CrossRef CAS PubMed;
(d) P. A. Peixoto, M. El Assal, I. Chataigner, F. Castet, A. Cornu, R. Coffinier, C. Bosset, D. Deffieux, L. Pouységu and S. Quideau, Angew. Chem., Int. Ed., 2021, 60, 14967 CrossRef CAS PubMed;
(e) N. Lebrasseur, J. Gagnepain, A. Ozanne-Beaudenon, J.-M. Léger and S. Quideau, J. Org. Chem., 2007, 72, 6280 CrossRef CAS PubMed;
(f) S. Hanessian, J. P. Maianti, V. L. Lya and B. Deschênes-Simarda, Chem. Sci., 2012, 3, 249 RSC;
(g) C. Li and J. A. Porco, J. Am. Chem. Soc., 2004, 126, 1310 CrossRef CAS PubMed.
-
(a) A. Paquin, C. Reyes-Moreno and G. Bérubé, Molecules, 2021, 26, 2340 CrossRef CAS PubMed;
(b) J. C. J. M. D. S. Menezes and M. F. Diederich, Eur. J. Med. Chem., 2019, 182, 111637 CrossRef CAS;
(c) J. C. Lee, G. A. Strobel, E. Lobkovsky and J. Clardy, J. Org. Chem., 1996, 61, 3232 CrossRef CAS;
(d) T. Zhu, Z. Lu, J. Fan, L. Wang, G. Zhu, Y. Wang, X. Li, K. Hong, P. Piyachaturawat, A. Chairoungdua and W. Zhu, J. Nat. Prod., 2018, 81, 254 CrossRef PubMed.
- Selected examples:
(a) Y. Tan, Z. Zhao, Z. Chen, S. Huang, S. Jia, L. Peng, D. Xu, W. Qin and H. Yan, Org. Lett., 2020, 22, 4461 CrossRef CAS PubMed;
(b) V. Claus, M. Schukin, S. Harrer, M. Rudolph, F. Rominger, A. M. Asiri, J. Xie and A. S. K. Hashmi, Angew. Chem., Int. Ed., 2018, 57, 12966 CrossRef CAS PubMed;
(c) C. Peng, T. Kusakabe, S. Kikkawa, T. Mochida, I. Azumaya, Y. Daulat Dhage, K. Takahashi, H. Sasai and K. Kato, Chem. – Eur. J., 2019, 25, 733 CrossRef CAS PubMed;
(d) R. Jia, B. Li, R. Liang, X. Zhang and X. Fan, Org. Lett., 2019, 21, 4996 CrossRef CAS PubMed;
(e) B. Kurpil, Y. Markushyna and A. Savateev, ACS Catal., 2019, 9, 1531 CrossRef CAS;
(f) M.-A. Constantin, J. Conrad, E. Merişor, K. Koschorreck, V. B. Urlacher and U. Beifuss, J. Org. Chem., 2012, 77, 4528 CrossRef CAS PubMed;
(g) M. Álvarez-Pérez, M. Frutos, A. Viso, R. Fernández de la Pradilla, M. C. de la Torre, M. A. Sierra, H. Gornitzka and C. Hemmert, J. Org. Chem., 2017, 82, 7546 CrossRef PubMed.
-
(a) M. H. Keylor, B. S. Matsuura and C. R. J. Stephenson, Chem. Rev., 2015, 115, 8976 CrossRef CAS PubMed;
(b) X. Su, D. Zhou and N. Li, Stud. Nat. Prod. Chem., 2022, 73, 265 CAS;
(c) T. Shen, X.-N. Wang and H.-X. Lou, Nat. Prod. Rep., 2009, 26, 916 RSC.
- Notable exceptions:
(a) Y. Takaya, K. Terashima, J. Ito, Y.-H. He, M. Tateoka, N. Yamaguchi and M. Niwa, Tetrahedron, 2005, 61, 10285 CrossRef CAS;
(b) M. Sako, H. Hosokawa, T. Ito and M. Iinuma, J. Org. Chem., 2004, 69, 2598 CrossRef CAS PubMed;
(c) T. Song, B. Zhou, G.-W. Peng, Q.-B. Zhang, L.-Z. Wu, Q. Liu and Y. Wang, Chem. – Eur. J., 2014, 20, 678 CrossRef CAS PubMed.
-
(a) B.-B. Yu, X.-Z. Han and H.-X. Lou, J. Agric. Food Chem., 2007, 55, 7753 CrossRef CAS PubMed;
(b) C.-S. Yao, L.-X. Zhou and M. Lin, Chem. Pharm. Bull., 2004, 52, 238 CrossRef CAS PubMed;
(c) C. Li, J. Lu, X. Xu, R. Hu and Y. Pan, Green Chem., 2012, 14, 3281 RSC.
- This has thus motivated synthetic chemists to develop alternative routes from more controllable building blocks. For a review:
(a) S. Quideau, D. Deffieux, C. Douat-Casassus and L. Pouységu, Angew. Chem., Int. Ed., 2011, 50, 586 CrossRef CAS PubMed For selected examples:
(b) W. Li, H. Li, Y. Li and Z. Hou, Angew. Chem., Int. Ed., 2006, 45, 7609 CrossRef CAS PubMed;
(c) W. Li, H. Li, Y. Luo, Y. Yang and N. Wang, Synlett, 2010, 1247 CrossRef CAS;
(d) B. S. Matsuura, M. H. Keylor, B. Li, Y. X. Lin, S. Allison, D. A. Pratt and C. R. J. Stephenson, Angew. Chem., Int. Ed., 2015, 54, 3754 CrossRef CAS PubMed;
(e) W. Li, T. Dong, P. Chen, X. Liu, M. Liu and X. Han, Tetrahedron, 2017, 73, 3056 CrossRef CAS;
(f) K. J. Romero, M. H. Keylor, M. Griesser, X. Zhu, E. J. Strobel, D. A. Pratt and C. R. J. Stephenson, J. Am. Chem. Soc., 2020, 142, 6499 CrossRef CAS PubMed;
(g) S. A. Snyder, T. C. Sherwood and A. G. Ross, Angew. Chem., Int. Ed., 2010, 49, 5146 CrossRef CAS PubMed;
(h) S. A. Snyder, N. E. Wright, J. J. Pflueger and S. P. Breazzano, Angew. Chem., Int. Ed., 2011, 50, 8629 CrossRef CAS PubMed;
(i) S. A. Snyder, A. Gollner and M. I. Chiriac, Nature, 2011, 474, 461 CrossRef CAS PubMed;
(j) S. A. Snyder, A. L. Zografos and Y. Lin, Angew. Chem., Int. Ed., 2007, 46, 8186 CrossRef CAS PubMed;
(k) F. Klotter and A. Studer, Angew. Chem., Int. Ed., 2014, 53, 2473 CrossRef CAS PubMed.
- Selected examples:
(a) S. S. Velu, I. Buniyamin, L. Kiew Ching, F. Feroz, I. Noorbatcha, L. C. Gee, K. Awang, I. A. Wahab and J.-F. F. Weber, Chem. – Eur. J., 2008, 14, 11376 CrossRef CAS PubMed;
(b) F.-J. Hong, Y.-Y. Low, K.-W. Chong, N. F. Thomas and T.-S. Kam, J. Org. Chem., 2014, 79, 4528 CrossRef CAS PubMed;
(c) K. J. Romero, M. S. Galliher, M. A. R. Raycroft, J.-P. R. Chauvin, I. Bosque, D. A. Pratt and C. R. J. Stephenson, Angew. Chem., Int. Ed., 2018, 57, 17125 CrossRef CAS PubMed;
(d) N. F. Thomas, K. C. Leeb, T. Paraidathathub, J. F. F. Weber, K. Awang, D. Rondeaud and P. Richommed, Tetrahedron, 2002, 58, 7201 CrossRef CAS;
(e) J.-S. Xie, J. Wen, X.-F. Wang, J.-Q. Zhang, J.-F. Zhang, Y.-L. Kang, Y.-W. Hui, W.-S. Zheng and C.-S. Yao, Molecules, 2015, 20, 22662 CrossRef CAS PubMed.
-
(a) V. Santacroce, R. Duboc, M. Malacria, G. Maestri and G. Masson, Eur. J. Org. Chem., 2017, 2095 CrossRef CAS;
(b) L. Jarrige, A. Carboni, G. Dagousset, G. Levitre, E. Magnier and G. Masson, Org. Lett., 2016, 18, 2906 CrossRef CAS PubMed;
(c) T. Le, L. Galmiche, G. Masson, C. Allain and P. Audebert, Chem. Commun., 2020, 56, 10742 RSC.
- Selected examples:
(a) T. Varlet, M. Matišić, E. Van Elslande, L. Neuville, V. Gandon and G. Masson, J. Am. Chem. Soc., 2021, 143, 11611 CrossRef CAS PubMed;
(b) W.-Y. Ma, C. Gelis, D. Bouchet, P. Retailleau, X. Moreau, L. Neuville and G. Masson, Org. Lett., 2021, 23, 442 CrossRef CAS PubMed;
(c) T. Varlet, C. Gelis, P. Retailleau, G. Bernadat, L. Neuville and G. Masson, Angew. Chem., Int. Ed., 2020, 59, 8491 CrossRef CAS PubMed;
(d) L. Jarrige, V. Gandon and G. Masson, Chem. – Eur. J., 2020, 26, 1406 CrossRef CAS PubMed;
(e) L. Jarrige, D. Glavač, G. Levitre, P. Retailleau, G. Bernadat, L. Neuville and G. Masson, Chem. Sci., 2019, 10, 3765 RSC;
(f) L. Jarrige, F. Blanchard and G. Masson, Angew. Chem., Int. Ed., 2017, 56, 10573 CrossRef CAS PubMed;
(g) C. Gelis, M. Bekkaye, C. Lebée, F. Blanchard and G. Masson, Org. Lett., 2016, 18, 3422 CrossRef CAS PubMed.
-
(a) J. Y. Lyu, A. Claraz, M. R. Vitale, C. Allain and G. Masson, J. Org. Chem., 2020, 85, 12843 CrossRef CAS PubMed;
(b) J. Y. Lyu, M. Leone, A. Claraz, C. Allain, L. Neuville and G. Masson, RSC Adv., 2021, 11, 36663 RSC.
-
(a) T. Inoue, S. Inoue and K. Sato, Chem. Lett., 1989, 4, 653 CrossRef;
(b) I. Tsutomu, I. Seiichi and S. Kikumasa, Bull. Chem. Soc. Jpn., 1990, 63, 1062 CrossRef;
(c) K. Chiba, J. Sonoyama and M. Tada, J. Chem. Soc., Chem. Commun., 1995, 1381 RSC;
(d) K. Chiba, Y. Yamaguchi and M. Tada, Tetrahedron Lett., 1998, 39, 9035 CrossRef CAS;
(e) M. Lanzi, J. Merad, D. V. Boyarskaya, G. Maestri, C. Allain and G. Masson, Org. Lett., 2018, 20, 5247 CrossRef CAS PubMed;
(f) C. Lebée, M. Languet, C. Allain and G. Masson, Org. Lett., 2016, 18, 1478 CrossRef PubMed;
(g) L. Jarrige, G. Levitre and G. Masson, J. Org. Chem., 2016, 81, 7230 CrossRef CAS PubMed See also:
(h) T. Courant, M. Lombard, D. V. Boyarskaya, L. Neuville and G. Masson, Org. Biomol. Chem., 2020, 18, 6502 RSC.
-
(a) S. Feng, B. Yang, T. Chen, R. Wang, Y.-H. Deng and Z. Shao, J. Org. Chem., 2020, 85, 5231 CrossRef CAS PubMed;
(b) Y. You, T.-T. Li, S.-P. Yuan, K.-X. Xie, Z.-H. Wang, J.-Q. Zhao, M.-Q. Zhoub and W.-C. Yuan, Chem. Commun., 2020, 56, 439 RSC;
(c) H. Zhang, G. Liu, X. Guan, J. Gao, X. Qin, G. Jiang, D. Sun, G. Zhang and S. Zhang, Eur. J. Org. Chem., 2019, 7264 CrossRef CAS.
- X. C. Cheng, P. R. Jensen and W. Fenical, J. Nat. Prod., 1999, 62, 608 CrossRef CAS PubMed.
- B. S. Siddiqui, I. A. Hashmi and S. Begum, Heterocycles, 2002, 57, 715 CrossRef CAS.
-
(a) H. Nishida, H. Tomoda, S. Okuda and S. Omura, J. Org. Chem., 1992, 57, 1271 CrossRef CAS;
(b) H. Tomoda, H. Nishida, R. Masuma, J. Cao, S. Okuda and S. i. Omura, J. Antibiot., 1990, 44, 136 CrossRef PubMed;
(c) H. Nishida, H. Tomoda, J. Cao, S. Okuda and S. Omura, J. Antibiot., 1990, 44, 144 CrossRef PubMed;
(d) G. M. Salituro, D. J. Pettibone, B. V. Clineschmidt, J. M. Williamson and D. L. Zink, Bioorg. Med. Chem. Lett., 1993, 3, 337 CrossRef CAS;
(e) D. Brückner, F.-T. Hafner, V. Li, C. Schmeck, J. Telser, A. Vakalopoulos and G. Wirtz, Bioorg. Med. Chem. Lett., 2005, 15, 3611 CrossRef PubMed.
- H. Yang, H.-R. Sun, R.-D. Xue, Z.-B. Wu, B.-B. Gou, Y. Lei, J. Chen and L. Zhou, Org. Lett., 2019, 21, 9829 CrossRef CAS PubMed.
-
(a) G. S. Hammond and J. Saltiel, J. Am. Chem. Soc., 1962, 84, 4983 CrossRef CAS;
(b) G. S. Hammond, J. Saltiel, A. A. Lamola, N. J. Turro, J. S. Bradshaw, D. O. Cowan, R. C. Counsell, V. Vogt and C. Dalton, J. Am. Chem. Soc., 1964, 86, 3197 CrossRef CAS.
- Although the concentration effect on photoisomerization is not completely understood, E- to Z-photoisomerization can be favoured, thanks to close proximity between the exited styrylnaphthol and styrylnaphthol at higher concentrations, see: A. A. Zimmerman, C. M. Orlando Jr., M. H. Gianni and K. Weiss, J. Org. Chem., 1969, 34, 73 CrossRef CAS.
- The starting material was totally recovered. The higher oxidation potential of phenol (Eox = 1.63 V vs. SCE) compared to that of naphthol (Eox = 1.40 V vs. SCE) might explain this difference in reactivity. H. G. Roth, N. A. Romero and D. A. Nicewicz, Synlett, 2016, 714 CAS.
- To develop an enantioselective version, few tests have been realized with chiral Brønsted acid catalysts but were unsuccessful; see the ESI† for more details.
- Compared to 2-naphthol, phenol features higher aromaticity and more difficult dearomatized tautomerization between enolate and ketone. Such a difference might explain the fact that styrylphenol was unreactive (see also ref. 14b).
- C. Ouannes and T. Wilson, J. Am. Chem. Soc., 1968, 90, 6527 CrossRef CAS.
- The position of the long wavelength tail of the absorption spectrum of 1a is around λtail(1a) = 385 nm while the short wavelength of the Hepatochem lamp (405 nm) is around λtail(lamp) = 380 nm. See the ESI† for more details.
|
This journal is © The Royal Society of Chemistry 2022 |
Click here to see how this site uses Cookies. View our privacy policy here.