DOI:
10.1039/D1QI01184G
(Research Article)
Inorg. Chem. Front., 2022,
9, 90-102
UiO-67-derived bithiophene and bithiazole MIXMOFs for luminescence sensing and removal of contaminants of emerging concern in wastewater†
Received
20th September 2021
, Accepted 9th November 2021
First published on 10th November 2021
Abstract
Solvent-assisted ligand exchange in the zirconium metal–organic framework (MOF) UiO-67 [Zr6O4(OH)4(PhPh)6; H2PhPh = biphenyl-4,4′-dicarboxylic acid] with the heterocyclic linkers H2TpTp (2,2′-bithiophene-5,5′-dicarboxylic acid) and H2TzTz (2,2′-bithiazole-5,5′-dicarboxylic acid) in an equimolar [Zr6]
:
linker ratio led to the corresponding MIXMOFs UiO-67-TpTp [Zr6O4(OH)4(PhPh)5(TpTp)] and UiO-67-TzTz [Zr6O4(OH)4(PhPh)5(TzTz)]. Both MOFs have been thoroughly characterized in the solid state. They show the same structural topology and crystal structure of UiO-67, as confirmed by powder X-ray diffraction. Their BET specific surface area is lower than that of their parent material (1700 and 1310 m2 g−1 for UiO-67-TpTp and UiO-67-TzTz, respectively, vs. 1877 m2 g−1 for UiO-67), while the thermal stability of UiO-67 (Tdec = 819 K) is maintained. The “diluted” inclusion, in the UiO-67 3D framework, of luminescent heterocyclic linkers emitting in the blue-green visible region induces luminescence in the final MOFs (emission λmax = 442 and 420 nm for UiO-67-TpTp and UiO-67-TzTz, respectively), preventing self-quenching effects caused by the proximity of the emitters in the crystal structure. Both MOFs have been tested as sensors for contaminants of emerging concern (CECs) in water: an emission intensity increase has been observed when suspended in contact with diclofenac sodium, fluoxetine, ibuprofen and sulfamethoxazole. In particular, UiO-67-TzTz has shown a linearly increasing trend for a specific concentration range (up to 30–40 μM for diclofenac, fluoxetine and ibuprofen; up to 100 μM for sulfamethoxazole). UiO-67-TzTz also behaves as an adsorbent vs. diclofenac, with a maximum adsorption capacity (Xm) of 62.5 mg g−1. These findings pave the way to the practical utilization of UiO-67-TzTz as a multifunctional material (i.e. CECs luminescent sensor and adsorbent at one time) for environmental remediation.
Introduction
Contaminants of emerging concern (CECs) are among the serious issues that our society and environment must presently face. CECs include different types of compounds, such as pharmaceuticals, personal care products and endocrine disrupting compounds.1 In recent years, CECs residues were detected in many different environments, including surface and ground waters as well as wastewater treatment plants, in almost every region of the World.2 In addition to their intrinsic toxicity, degradation of CECs may also lead to the formation of toxic by-products or metabolites. Pharmaceuticals, in particular, are a huge class of compounds with very different chemical and biological properties. Many of them are not fully metabolized by the human body, passing through to contaminate our water systems.3 Antibiotics are among the most used pharmaceuticals.4 Their consumption has grown significantly in recent years, due to the increase of both World population and wealth of developing countries. Several studies have shown that antibiotics are not completely degraded by conventional wastewater treatment processes,5 leading to increasing concentrations of these compounds in the environment.2 Hence, it is essential to develop and implement water treatment processes which are effective in detecting and/or removing from (waste)waters water-soluble antibiotics and anti-inflammatory drugs. The importance of this issue is witnessed by the fact that it is included in one of the seventeen Sustainable Development Goals of the United Nations for 2030, namely: Goal 6, Clean water and sanitation (https://sdgs.un.org/goals), and it is at the forefront of the upcoming Horizon Europe research topics for the 2020–2023 period. Consequently, it is growing in importance and research efforts destinated to solve it. Being featured by high specific surface area, Metal–organic Frameworks (MOFs) are a promising class of materials that may be exploited in this field. MOFs are 3D coordination polymers with open structures derived from the self-assembly of poly(topic) ligands and metal ions or metal-based clusters.6–8 MOFs can efficiently adsorbe different substances, such as gases or small (bio)molecules. In the field of environmental remediation, several MOFs and MOF composites have been studied for CECs removal in waters.1,9 In addition to the high specific surface area, tailored MOFs may also have other valuable physical properties, such as luminescence. In MOFs, luminescence may have different origin.10–13 Linker-based luminescence is the most common one and it is found in MOFs containing carbo- or heterocyclic linkers with extended π-delocalized electronic density, absorbing in the visible or UV region, combined with metallic nodes containing metal ions in d10 or d0 electronic configuration, like ZnII or ZrIV (i.e., largely electronically inert ions).13 The presence of heteroatoms in this kind of ligands shifts the emission wavelength in the visible region. Thiophene and thiazole, e.g., are among the simplest (N,S)-containing heterocycles and are intrinsically fluorescent.19,20 Hence, in recent years there has been a growing interest in heterocycles as constitutive parts of MOF linkers. In the literature, many luminescent MOFs are exploited as CECs sensors in water solutions.14–16 However, very few studies report on multifunctional MOFs that simultaneously detect and adsorb pollutants.17,18 The use of such advanced materials could lead to a significant progress in the field of environmental remediation.
Starting from our long-lasting interest in the preparation of MOFs and coordination polymers containing polytopic thiazole- and thiophene-based carboxylic acids for different applications,21–29 in this work we present the synthesis and full solid-state characterization of two mixed-ligand MOFs (MIXMOFs) derived from the iconic ZrIV MOF UiO-67 (UiO = University of Oslo)30 upon Solvent-Assisted Ligand Exchange (SALE), namely: [Zr6O4(OH)4(PhPh)5(TpTp)] (UiO-67-TpTp; H2PhPh = biphenyl-4,4′-dicarboxylic acid, H2TpTp = 2,2′-bithiophene-5,5′-dicarboxylic acid) and [Zr6O4(OH)4(PhPh)5(TzTz)] (UiO-67-TzTz; H2TzTz = 2,2′-bithiazole-5,5′-dicarboxylic acid, Scheme 1). This contribution follows our previous work on UiO-67-type single-ligand and mixed-ligand MOFs built exclusively with heterocyclic linkers.21 When excited with electromagnetic radiation belonging to the UV region, these MOFs exhibited solid-state luminescence in the blue-green visible region. Regrettably, the emission intensity was below the limit required for practical applications. This occurrence was ascribed to the formation of linker domains promoting fluorescence emission self-quenching, as already observed in other UiO-67-based MIXMOFs of the literature.31 In the two MIXMOFs presented here, the pristine carbocyclic PhPh2− linker of UiO-67 has been only partially replaced by the heterocyclic bithiophene- or bithiazole-based analogue, yielding two MIXMOFs that maintain the luminescence features of the post-synthetically introduced heteroaromatic ligands. In this respect, SALE has proved to be extremely effective to avoid the formation of domains of the same linker in the MIXMOFs with the consequent undesired self-quenching. The two MIXMOFs have been tested as luminescent sensors for selected CECs, namely: diclofenac sodium (DCF), fluoxetine (FXT), sulfamethoxazole (SMZ) and ibuprofen (IBR, Scheme S1†). Based on the luminescence sensing results, the adsorption properties of UiO-67-TzTz towards DCF have also been assessed.
 |
| Scheme 1 Molecular structure of the three linkers used in this study: the carbocyclic biphenyl-4,4′-dicarboxylic acid H2PhPh (also labeled H2BPDC in the literature) and its two heterocyclic analogues 2,2′-bithiophene-5,5′-dicarboxylic acid (H2TpTp) and 2,2′-bithiazole-5,5′-dicarboxylic acid (H2TzTz). | |
Experimental section
Materials and methods
All the chemicals and reagents employed were used as received from commercial suppliers. Deuterated solvents (Sigma Aldrich) were stored over 4 Å molecular sieves and degassed by three freeze–pump–thaw cycles before use. Literature recipes were followed to prepare UiO-67,32H2TpTp
33 and H2TzTz.231H NMR spectra were recorded on a BRUKER AVANCE 400 MHz spectrometer, with chemical shifts (δ) reported in parts per million (ppm) downfield of tetramethylsilane (TMS) and calibrated against the residual protiated solvent resonance. FT-IR spectra (KBr pellets) were recorded on a PerkinElmer Spectrum BX Series FTIR spectrometer, in the 4000–400 cm−1 range, with a 2 cm−1 resolution. Thermogravimetric analyses (TG-DTG) were performed under a N2 flow (100 mL min−1) at a heating rate of 10 K min−1 with an EXSTAR Thermo Gravimetric Analyzer Seiko 6200. The latter was coupled with a ThermoStarTM GSD 301T for mass analysis of volatile species. The elemental analyses were performed using a Thermo FlashEA 1112 Series CHNS–O elemental analyzer with an accepted tolerance of ±4% on carbon (C), hydrogen (H), nitrogen (N) and sulfur (S). Powder X-ray diffraction (PXRD) qualitative measurements were carried out in the 2.0–50.0° 2θ range with a Panalytical X'PERT PRO powder diffractometer equipped with a sealed X-ray tube (Cu Kα, λ = 1.5418 Å), a filter of nickel in the diffracted beam, and a PIXcel© solid state detector. Slits were used on both the incident (Soller, 0.25°, and divergence, 0.5°) and the diffracted (antiscatter, 7.5 mm height) beam. X-ray fluorescence (XRF) qualitative elemental analysis was performed on a powdered batch (ca. 10 mg) of UiO-67-TzTz and [DCF@UiO-67-TzTz] with a Panalytical MINIPAL 2 instrument equipped with a Cr X-ray source.
Synthesis of [Zr6O4(OH)4(PhPh)5(TpTp)]·5(DMF) (UiO-67-TpTp·DMF)
UiO-67 {[Zr6O4(OH)4(PhPh)6], FW = 2120.63 g mol−1, 213 mg, 0.10 mmol} was suspended in a N,N-dimethylformamide (DMF) solution (7 mL) of H2TpTp (FW = 254.27 g mol−1, 25 mg, 0.10 mmol). The obtained suspension was heated at T = 393 K under gentle magnetic stirring. After 12 h, the mixture was cooled down to ambient temperature and the yellow solid, UiO-67-TpTp·DMF, was filtered over a 0.2 μm PTFE filter, subsequently washed with hot DMF (353 K, 2 × 10 mL), ethanol (3 × 10 mL) and petroleum ether (3 × 10 mL), and finally dried under a N2 flow at ambient temperature. Yield: 213 mg (85% based on zirconium). The extent of biphenyl/bithiophene linker exchange was estimated through signal integration of the 1H NMR spectrum of the solution obtained after digesting the sample in a HF(aq)/DMSO-d6 mixture heated at 343 K for 12 h (see ESI and Fig. S1†). In line with the initial reaction stoichiometry, the loading achieved is one TpTp2− ligand per [Zr6] node. The phase purity of every batch was checked through PXRD. Elemental analysis (%) calcd for UiO-67-TpTp·DMF, C95H83N5O37S2Zr6 (MW = 2498.16 g mol−1): C 45.67, H 3.35, N 2.80, S 2.57; found: C 45.68, H 3.35, N 2.83, S 2.58. IR [ν(C
O)] band (KBr, cm−1, Fig. S2†): 1678 (s), 1605 (s).
Synthesis of [Zr6O4(OH)4(PhPh)5(TzTz)]·5(DMF) (UiO-67-TzTz·DMF)
UiO-67 {[Zr6O4(OH)4(PhPh)6], FW = 2120.63 g mol−1, 268 mg, 0.13 mmol} was suspended in a DMF solution (7 mL) of H2TzTz (FW = 256.24 g mol−1, 32 mg, 0.13 mmol). The suspension was heated at T = 393 K under gentle magnetic stirring. After 12 h, the reaction mixture was cooled down to ambient temperature and the grayish solid, UiO-67-TzTz·DMF, was filtered over a 0.2 μm PTFE filter, subsequently washed with hot DMF (353 K, 2 × 10 mL), ethanol (3 × 10 mL) and petroleum ether (3 × 10 mL), and finally dried under a nitrogen stream at ambient temperature. Yield: 200 mg (61% based on zirconium). The extent of biphenyl/bithiazole linker exchange was estimated through signal integration of the 1H NMR spectrum of the solution obtained after digesting the sample in a HF(aq)/DMSO-d6 mixture heated at 343 K for 12 h (see ESI and Fig. S3†). In line with the initial reagents stoichiometry, the loading achieved is one TzTz2− ligand per [Zr6] node. The phase purity of every batch was checked through PXRD. Elemental analysis (%) calcd for UiO-67-TzTz·DMF, C93H81N7O37S2Zr6 (MW = 2500.13 g mol−1): C 44.68, H 3.27, N 3.92, S 2.57; found: C 44.66, H 3.23, N 3.89, S 2.54. IR [ν(C
O)] band (KBr, cm−1, Fig. S4†): 1683 (w, sh), 1590 (s).
Synthesis of [Zr6O4(OH)4(PhPh)5(TzTz)]·(DCF) (DCF@UiO-67-TzTz)
UiO-67-TzTz·DMF (40 mg, 0.016 mmol) was suspended in an aqueous solution (10 mL) of diclofenac sodium (FW = 318.15 g mol−1, 51 mg, 0.16 mmol, 10 equiv.) at T = 343 K under gentle magnetic stirring. After 15 h, the suspension was cooled to ambient temperature and the solid was filtered off, washed with water (1 × 10 mL), ethanol (2 × 10 mL), petroleum ether (2 × 10 mL), and finally dried under a nitrogen stream at ambient temperature. Yield: 39 mg (quantitative).
Powder X-ray diffraction structure characterization
PXRD data acquisitions were carried out using a Bruker AXS D8 Advance vertical-scan θ:θ diffractometer, equipped with a sealed X-ray tube (Cu Kα, λ = 1.5418 Å), a filter of nickel in the diffracted beam, a Bruker Lynxeye linear position-sensitive detector, and the following optical components: primary beam Soller slits (2.5°), fixed divergence slit (0.5°), antiscatter slit (8 mm). The generator was set at 40 kV and 40 mA. A powdered sample (∼50 mg) of UiO-67-TzTz·DMF was introduced into the cavity of a silicon free-background sample-holder 0.2 mm deep (Assing Srl, Monterotondo, Italy). The purity and crystallinity of the sample were checked carrying out a preliminary PXRD acquisition in the 2θ range 3.0–35.0°, with steps of 0.02° and time per step of 1 s. The PXRD acquisition for the assessment of the crystal structure was performed overnight in the 2θ range 3.0–105.0°, with steps of 0.02° and an approximate scan time of about 12 h. A visual comparison between the PXRD pattern of UiO-67-TzTz·DMF and that of UiO-67
34 suggested that the two MOFs are isostructural. A whole powder pattern profile refinement with the so-called Le Bail approach35 performed with TOPAS-R V336 employing the space group and unit cell parameters of UiO-67 confirmed the suggestion. The crystallographically independent portion of PhPh2−, TzTz2− and DMF were modelled as rigid bodies using the z-matrix formalism, assigning to the bond distances and angles37 idealized values based on a search in the Cambridge Structural Database (v. 2021) for room-temperature and -pressure crystal structures containing the three molecules. The molar ratio between the PhPh2− and TzTz2− ligands was set at the value retrieved from the 1H-NMR signal integration of the digested sample (see the main text and the ESI†). In the initial steps of the structure assessment, both the metal cluster atoms (Zr and O) and the centre of mass of the rigid bodies describing the spacers were located according to the crystal structure of UiO-67
34 and [Zr6O4(OH)4(TzTz)6]·n(DMF),23 adopting an idealized distribution of the two linkers in both independent positions. The linkers orientation was then allowed to refine. Two crystallographically independent DMF molecules and a dummy oxygen atom representing the smeared electron density in the cavities not described by DMF were located using the Simulated Annealing approach,38 implemented in TOPAS-R V3. A Chebyshev-type polynomial function was employed to describe the background. An isotropic thermal factor [Biso(M)] was refined for the ZrIV ion; the isotropic thermal factor of the oxygen atoms belonging to the cluster and the ligands was calculated as Biso(L) = Biso(M) + 2.0 (Å2); the isotropic thermal factor of the atoms describing the chlatrated solvent was calculated as Biso(S) = Biso(M) + 3.0 (Å2). The peak profile was modelled trough the Fundamental Parameters Approach.39 The anisotropic peak broadening was described by means of Gaussian and Lorentzian spherical harmonics. During the final stages of the structural refinement, instrumental and structural parameters were collectively refined by means of the so-called Rietveld refinement.40 The final Rietveld refinement plot is shown in Fig. S5 of the ESI.†
Crystal data for UiO-67-TzTz·DMF, C78H46N2O32S2Zr6·Solv, cubic, Pn-
, a = 26.842(1) Å, V = 19
339(3) Å3, Z = 24, Z′ = 4, ρ = 0.922 g cm−3, F(000) = 5404.8, RBragg = 0.005, Rp = 0.019 and Rwp = 0.024, for 5051 data and 78 parameters in the 4.0–105.0° (2θ) range. CCDC no. 2103802.†
Gas adsorption
Both MIXMOFs (∼40 mg samples) were activated at 453 K under high vacuum (10−6 Torr) for 3 h before each measurement. The textural properties were evaluated through volumetric N2 adsorption at 77 K on an ASAP 2020 Micromeritics instrument. For the Brunauer–Emmett–Teller (BET) specific surface area calculation, the 0.01–0.1 p/p0 pressure range of the isotherm was used. Within this range, all the Rouquerol consistency criteria are satisfied.41,42 The micro- and mesopore size was evaluated through NLDFT methods (Tarazona model for cylindrical pores).
Luminescence sensing experiments
Luminescence experiments were performed on pre-activated MIXMOF samples using a Cary Eclipse fluorescence spectrometer. As a preliminary test of the MIXMOFs water stability, a weighted amount (5 mg) of material was dispersed into 25 mL of distilled water and sonicated for about 30 minutes. After this time, the suspension was transferred into a fluorimetric 1 cm path cuvette and luminescence spectra were registered with a 380 nm excitation source. To test the sensing properties of the materials, the protocol reported by Zhong et al.43 was used, with some modifications. The MIXMOF aqueous suspension (2 mL) was placed in the cuvette, as described above. Subsequently, aliquotes of an aqueous solution (100 mg L−1) of each pollutant [diclofenac sodium (DCF), fluoxetine (FXT), sulfamethoxazole (SMZ) and ibuprofen (IBR), Scheme S1†] were gradually added, and the cuvette was shaken for about 30 seconds. Then, the luminescence spectrum of the MIXMOF-pollutant system was registered. Wavelength shifts and/or signal intensity changes were monitored.
Testing of pollutant adsorption and desorption
Based on the results of luminescence sensing, UiO-67-TzTz was tested for the adsorption of DCF using the following protocol. A not-activated sample (4 mg) of the MIXMOF was suspended in an aqueous solution of the pollutant (2 mL) in a glass vial. The mixture was gently stirred for 6 hours at room temperature; this time interval was chosen as it is considered ideal to reach a plateau in the adsorption profile, according to what is reported in the literature.44 Experiments with different pollutant concentrations (in the range 5–200 mg L−1) were carried out. At the end of the experiments, the suspension was centrifuged to separate the MIXMOF from the solution; the supernatant was analyzed with UV-Vis spectroscopy (λmax = 276 nm) to determine the residual pollutant concentration. The experiments were performed in duplicate; an average value of the two results was adopted for the discussion. The data were fitted with the Langmuir model, according to the equation:
where Ce is the concentration of the contaminant at the equilibrium (mg L−1), qe is the amount of contaminant adsorbed for unit of material (mg g−1), Xm is the maximum adsorption capacity of the material for the specific pollutant (mg g−1) and K is a parameter related to the affinity between the pollutant and the adsorbent. DCF desorption from UiO-67-TzTz was tested for selected pollutant concentrations. At the end of the adsorption process, the supernatant was separated from the solid by centrifugation; the solid was dried, then suspended in distilled water (2 mL) and left under stirring at ambient temperature. After 6 h, the solid was separated by centrifugation and the supernatant was analyzed in terms of its DCF concentration as described above.
Results and discussion
MIXMOFs synthesis and characterization
Following the solvent-assisted ligand exchange (SALE) consolidated approach for the preparation of mixed-ligand zirconium MOFs,45–47 as-prepared UiO-67 (whose identity and purity was previously checked by PXRD) was suspended in a N,N-dimethylformamide (DMF) solution containing the heterocyclic linker H2TpTp or H2TzTz in a 1
:
1 = MOF
:
linker molar ratio. Heating at 393 K for 12 h under gentle magnetic stirring led to the partial replacement of the H2PhPh linker with their heterocyclic analogues, giving two new MIXMOFs with a 5
:
1 ratio of the two spacers, as expected from the initial stoichiometry adopted for the synthesis. A proof of evidence of the occurred ligand exchange came from the 1H NMR analysis of the supernatant (DMF-d7): after the reaction time of 12 h, the two doublets ascribed to the two different proton sets of H2PhPh at δH = 7.95 and 8.17 ppm were clearly visible in solution, along with those of unreacted H2TpTp (δH = 7.60 and 7.80 ppm) or H2TzTz (δH = 8.60 ppm, Fig. S6 and S7†). IR spectroscopy on the recovered solid undoubtedly confirmed the exchange, as witnessed by the appearance of new ν(COO) vibrational modes at 1678 cm−1 (TpTp2−) or 1683 cm−1 (TzTz2−), besides those of PhPh2− at 1590–1600 cm−1.48 There is no evidence of uncoordinated or mono-coordinated carboxylate groups, whose ν(COO) stretching modes would be found at higher wavenumbers.49 The final MIXMOFs composition was assessed through both elemental analysis and digestion of the solid in an acidic solution [HF(aq)/DMSO-d6] followed by 1H NMR spectrum signal integration. The “dilution” of the heterocyclic linker within UiO-67 is of fundamental importance to get crystalline porous materials with a reasonably strong luminescence emission intensity. As anticipated in the Introduction, it has been proved by both our group21 and others in the literature31,50 that a MOF exclusively built with emitting linkers may undergo a severe emission self-quenching caused by the proximity of the emitters in the crystal structure.
UiO-67-TpTp and UiO-67-TzTz have been thoroughly characterized in the solid state. The parent crystallographic symmetry and fcu network topology of UiO-67 and of other UiO-67-type (MIX)MOFs containing heterocyclic spacers such as [Zr6O4(OH)4(L)6]·n(DMF) (L = SpSp2− = [2,2′-biselenophene]-5,5′-dicarboxylate;21 L = TpTp2−;33 L = TzTz2− (ref. 23)) is maintained after SALE, as confirmed by PXRD (Fig. 1a). X-ray diffraction alone cannot assess whether the ligands in the two MIXMOFs adopt a random, alternating or cluster distribution.51,52 As a representative example, we assessed the crystal structure of UiO-67-TzTz, briefly described here for the sake of completeness. UiO-67-TzTz·DMF crystallizes in the cubic space group Pn-
. The unit cell parameter of UiO-67-TzTz·DMF [26.842(1) Å] is comparable with that of UiO-67 [26.8809(3)], but longer than those of the heterocycle-based UiO-67-type MOFs [25.224(3)–25.702(1)], in agreement with the presence of the longer PhPh2− spacer as one of the two mixed ligands. The crystal structure contains {Zr6O4(OH)4} clusters as nodes (Fig. 2a): each ZrIV ion of the node is connected, in a square-antiprismatic fashion, to eight oxygen atoms, four belonging to the carboxylate groups, which build up one square face of the antiprism, and four coming from μ3-O and μ3-OH groups, which form the other square face. The [Zr6] node is connected to twelve others by the linkers. Even if PhPh2− is longer than TzTz2− (ref. 37) also the latter is tetradentate, as preliminarily suggested by IR spectroscopy. The Zr–O distances involving TzTz2− are longer than those involving PhPh2− [Zr–OPhPh 2.024(14)–2.397(27) Å vs. Zr–OTzTz 2.395(59)–2.592(33), respectively]. Nevertheless, they are still compatible with the formation of Zr–O coordination bonds.53 The framework (Fig. 2b) features octahedral and tetrahedral cages (with diameter of ∼12 Å and ∼9 Å, respectively)54 that are occupied by the clathrated solvent. Neglecting the solvent molecules, the empty volume estimated at ambient conditions with the software PLATON55 is ∼66%, in agreement with those found in UiO-67 (64%)34 and in the other heterocycle-based UiO-67-type MOFs (61–65%).21 As in the crystal structure of H2TzTz,23 the heterocycles in TzTz2− adopt a reciprocal trans disposition, even though the two heterocyclic rings are not coplanar (with a deviation of about 9.5°), as in the other heterocycle-based UiO-67-type MOFs.21
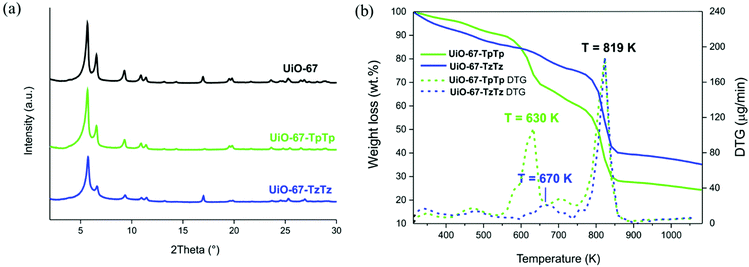 |
| Fig. 1 (a) PXRD patterns of UiO-67, UiO-67-TpTp and UiO-67-TzTz at comparison; (b) TGA–DTG profiles of UiO-67-TpTp and UiO-67-TzTz. | |
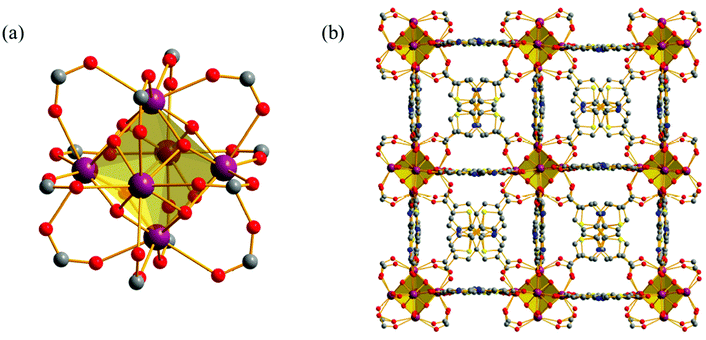 |
| Fig. 2 Representation of the crystal structure of UiO-67-TzTz·DMF: (a) the node; (b) portion of the crystal structure viewed along one of the unit cell axes. Hydrogen and solvent atoms have been omitted for clarity of representation. Color code: carbon, grey; nitrogen, blue; oxygen, red; sulphur, yellow; zirconium, purple. Zr–OPhPh = 2.024(14)–2.397(27) Å; Zr–OTzTz = 2.395(59)–2.592(33) Å; remaining Zr–O = 2.063(97)–2.229(83) Å. | |
The thermogravimetric analysis (TGA, Fig. 1b) showed that both MIXMOFs are as stable as UiO-67
48 (Tdec = 819 K); thus, linker partial substitution does not seem to affect the material thermal stability. For both MOFs, an initial weight loss of ca. 25 wt% with a maximum loss at T ∼630 and 670 K (for UiO-67-TpTp and UiO-67-TzTz, respectively) can be reasonably ascribed to the heterocyclic linker and clathrated DMF loss (calculated loss: 24.7 and 24.8 wt% for UiO-67-TpTp and UiO-67-TzTz, respectively). The presence of weaker (i.e. longer) Zr–OTzTz coordination bonds with respect to the other Zr–O bonds within the [Zr6] cluster (see above) may explain the loss of TzTz2− before decomposition. Further proof of evidence is provided by the MS analysis of the volatiles, where a peak at m/z = 73 a.m.u. typical of DMF appears in the same temperature range, and by the decomposition temperatures of pure H2TpTp and H2TzTz (Tdec = 650 and 570 K, respectively).56 The solid residue at the end of the decomposition at T = 1023 K equals 25.4 and 36.8 wt% for UiO-67-TpTp and UiO-67-TzTz, respectively.
The porosity of UiO-67-TpTp and UiO-67-TzTz was evaluated through volumetric N2 adsorption at 77 K on activated samples (Fig. 3a). The isotherms shape is typical of microporous materials and it is similar to that of UiO-67. The BET specific surface areas are lower than that of pristine UiO-67 (1700 and 1310 m2 g−1, for UiO-67-TpTp and UiO-67-TzTz respectively, vs. 1877 m2 g−1 found in UiO-67
48), with a total pore volume at p/p0 = 0.98 of 0.86 cm3 g−1 (UiO-67-TpTp) and 0.63 cm3 g−1 (UiO-67-TzTz) (vs. 0.95 cm3 g−1 for UiO-67).48 The NLDFT mesopore size (Tarazona model for cylindrical oxide-like pores, Fig. 3b) shows a slight variation when passing from UiO-67 (22 Å)32,48 to UiO-67-TpTp or UiO-67-TzTz (24 Å). This proves that SALE does not alter the mesopores morphology in the exchanged materials.
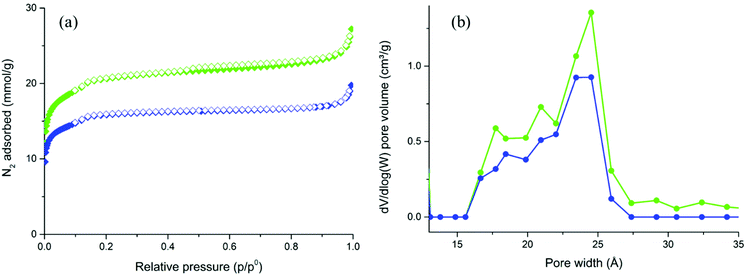 |
| Fig. 3 (a) N2 adsorption isotherms of UiO-67-TpTp (green diamonds) and UiO-67-TzTz (blue diamonds) at comparison. The desorption branch is depicted with empty symbols. (b) NLDFT (Tarazona model) pore size distribution plots for UiO-67-TpTp (green circles) and UiO-67-TzTz (blue circles) at comparison. | |
MIXMOFs luminescence properties and sensing experiments with CECs
The stability of the newly prepared MIXMOFs in aqueous suspensions was preliminarily assessed soaking both of them in distilled water for 12 h at ambient temperature. The PXRD patterns collected for UiO-67-TpTp (Fig. S8†) and UiO-67-TzTz (Fig. S9†) before and after soaking showed that the pristine degree of crystallinity is maintained under the essayed conditions. This behavior is different from that of pure UiO-67, for which a limited tolerance vs. water has been reported.57 The emission spectra of the two MIXMOFs are reported in Fig. 4. Both materials show a clear and defined signal, with λmax at 420 and 442 nm for UiO-67-TzTz and UiO-67-TpTp, respectively. These λmax match well with those of the bare heterocyclic linkers in DMF solution,21 falling in the same spectral range and generating characteristic blue/green colors upon irradiation under a UV lamp. This proves that (i) the luminescence of the MIXMOFs is ligand-centered and stems from their heterocyclic components only (biphenyl-4,4′-dicarboxylate does not emit in the visible region) and (ii) the most likely ligands distribution in the crystal structure is random and not characterized by the formation of single-ligand domains (that would induce a spontaneous emission self-quenching).
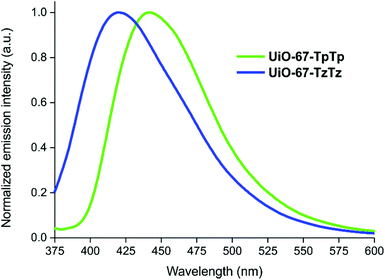 |
| Fig. 4 Emission spectra of UiO-67-TzTz and UiO-67-TpTp powders suspended in distilled water. | |
Luminescence sensing experiments were then performed with four CECs, namely: diclofenac sodium (DCF), fluoxetine (FXT), ibuprofen (IBR) and sulfamethoxazole (SMZ, Scheme S1†). Both MIXMOFs show changes of their luminescence properties when in contact with these contaminants. The changes were monitored upon addition of increasing amounts of pollutant. The results are shown in Fig. S10,† where the changes in the signal intensity (ΔI) are reported as a function of the concentration of the contaminants. The luminescent response of UiO-67-TpTp is particularly good for IBR, while for UiO-67-TzTz is stronger for DCF. For the majority of the studied contaminants, an emission intensity increase was observed with both materials. The increase of the signal intensity is ascribed to the enhanced rigidity of the MOF heterocyclic linkers when a guest is present into the pores, with consequent elimination of non-radiative decay processes responsible for signal quenching,58 as demonstrated by the successful use of this approach for the synthesis of fluorescent MOFs.59 However, for the luminescent sensing of contaminants signal intensity enhancement is not the most commonly observed response; a decrease of the signal intensity is normally observed instead.43,60
Fig. 5 shows a comparison of the response of the two MIXMOFs for each contaminant. Considering DCF (Fig. 5a), a similar intensity increase was observed with both materials for concentrations up to 20 μM (0.15 DCF
:
MOF molar ratio). For higher concentrations, no further increase in intensity was observed with UiO-67-TpTp and the signal reached a plateau. On the other hand, for UiO-67-TzTz a stronger emission intensity was still measured for concentrations up to 30 μM (0.4 DCF
:
MOF ratio) before reaching saturation. The negatively charged carboxylate group in DCF engages in stronger electrostatic interactions with UiO-67-TzTz where the presence of the thiazole N atom increases the heterocycle polarity if compared with thiophene. In UiO-67-TzTz, the emission intensity increase for concentrations up to 30 μM can be fitted with a linear correlation (Fig. S11a†); the material thus acts as a quantitative luminescent sensor in this concentration range. With FXT (Fig. 5b), a comparable intensity trend was observed for both materials, although the highest intensity was registered for different contaminant concentrations – about 40 and 60 μM for UiO-67-TpTp and UiO-67-TzTz, respectively. Moreover, at the signal saturation there is a larger oscillation for UiO-67-TpTp than for UiO-67-TzTz.
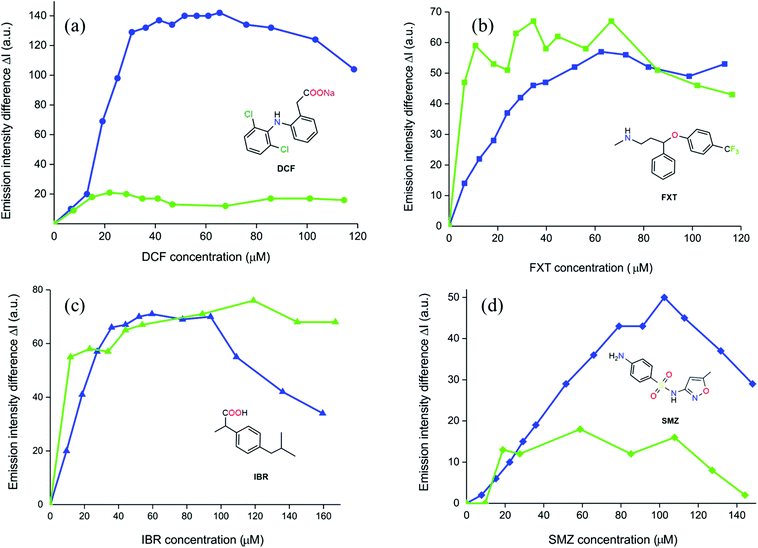 |
| Fig. 5 Emission spectra of the MIXMOF samples as a function of the CECs concentration. (a) DCF; (b) FXT; (c) IBR; (d) SMZ. Green lines: UiO-67-TpTp; blue lines: UiO-67-TzTz. | |
Consequently, only the latter was considered for a linear fitting, as reported in Fig. S11b.† A good correlation was observed for concentrations up to 40 μM, a range comparable to that of DCF. The slope of the FXT curve though is smaller (1.3 vs. 4.4 ΔI/μM−1 for FXT vs. DCF, respectively), indicating that the sensitivity of UiO-67-TzTz for this contaminant is lower. This difference in sensing response can be explained considering the lower FXT polarity in comparison with DCF. FXT does not have an integer charge, although the presence of the C–F bonds still confers polarity to the molecule. On the basis of the experimental data and the aforementioned considerations, we can claim that there are still significant electrostatic interactions between FXT and UiO-67-TzTz, but their strength is lower than those involving DCF. For IBR (Fig. 5c), both MIXMOFs show a similar behaviour for concentrations up to 45 μM (i.e. a linear increase followed by a plateau). Considering the concentration range with a linear increase (up to almost 40 μM), the fitting performed for UiO-67-TzTz showed a good correlation (Fig. S11c†). The sensitivity is slightly higher than that measured for FXT (the slopes of the fitting are 1.9 vs. 1.3 ΔI/μM−1 for IBR vs. FXT, respectively). This difference can again be explained invoking polarity. IBR is more polar than FXT due to the presence of a carboxylic group. Tests with SMZ showed that UiO-67-TzTz has again higher sensitivity than UiO-67-TpTp, as the emission intensity variation is more enhanced in the former case (Fig. 5d). A linear increase for concentrations up to almost 100 μM was measured (1.5 SMZ
:
MOF ratio), the larger value registered among the scrutinized CECs. This reveals that higher amounts of SMZ can be included in the MIXMOF pores before reaching saturation. Since there are no significant size differences among the tested contaminants, the origin of the observed behaviour may be due to the formation of aggregates into larger micelles in solution. This phenomenon has already been described for some pharmaceuticals (including DCF and IBR); the size of the aggregates depends on various factors, including the solution concentration and the presence of other molecules able to form micelles like dodecylphosphocholine.61–63 The formation of these aggregates is more likely for DCF or IBR than for SMZ, limiting the diffusion of the former through the MOF pores. The linear fitting (Fig. S11d†) showed a low sensitivity (0.5 ΔI/μM−1) but the sensing capability extends to a larger concentration range, as stated above. The collected evidence can be summarized as follows: UiO-67-TzTz generally gives better performances and a linear correlation between emission intensity and concentration. For DCF, FXT and IBR, the range of linear emission response extends for concentrations up to 30–40 μM, while for SMZ the quantitative detection limit is almost 100 μM. The highest sensitivity and related capacity to detect lower concentrations in wastewater was observed for DCF. Following these results, UiO-67-TzTz was also tested as DCF “sponge”, to remove the contaminant from the supernatant aqueous solution. To prove the effective drug inclusion into the MOF, a sample of UiO-67-TzTz was suspended on an aqueous DCF solution (with the pollutant in strong excess with respect to the MOF, 10 equivalents) and kept under gentle stirring at T = 343 K for 15 h, to obtain the [DCF@UiO-67-TzTz] derivative. The non-ambient temperature was chosen with the aim of promoting DCF diffusion into the MOF pores after exchange of the DMF solvent present in the pores of the as-synthesised sample. After extensive washing to remove unreacted DCF, the solid sample was examined through IR spectroscopy, TGA–DTG thermal analysis and X-ray fluorescence spectroscopy (XRF). The [DCF@UiO-67-TzTz–UiO-67-TzTz·DMF] IR difference spectrum (Fig. 6a) reveals the presence of the typical DCF vibrational modes at ∼1570 [ν(COO−)], 1450 [ν(C
C)] and 750 cm−1 [γ(CH)]. The TGA–DTG profile recorded on [DCF@UiO-67-TzTz] (Fig. 6b) shows an additional weight loss at T = 545 K with respect to UiO-67-TzTz·DMF (cfr.Fig. 1b), perfectly matching with DCF decomposition temperature (reported at T = 544 K for its first thermal event).64 Furthermore, the weight loss observed for this step (∼13.0 wt%) is in excellent agreement with the hypothetical formula [Zr6O4(OH)4(PhPh)5(TzTz)]·(DCF).
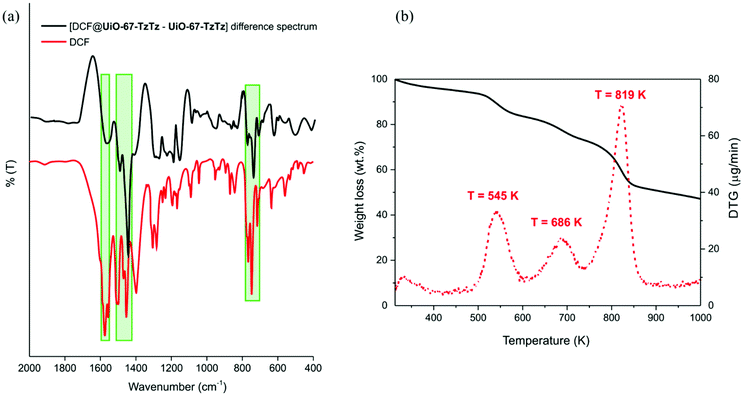 |
| Fig. 6 (a) [DCF@UiO-67-TzTz–UiO-67-TzTz·DMF] IR difference spectrum (KBr pellet) in comparison with the IR spectrum of pure diclofenac sodium. The most typical DCF vibrational modes are highlighted with green boxes. (b) TGA–DTG profile of [DCF@UiO-67-TzTz]. | |
The XRF spectrum of [DCF@UiO-67-TzTz] shows the characteristic lines of sodium and chlorine, absent in the pristine MIXMOF (Fig. S12†). These observations confirm the successful DCF inclusion into UiO-67-TzTz. Unfortunately, the drug-loaded sample showed a low crystallinity degree (Fig. S13†) which enabled us to assess the retention of the parent 3D framework, but was not suitable for structure determination.
Fig. 7a shows the isotherm adsorption of the [DCF@UiO-67-TzTz] system, while Fig. 7b shows the removal efficiency as a function of the initial DCF concentration. DCF is completely removed at low concentrations (∼20 mg L−1); for higher values, a decrease in the removal efficiency was registered (just above 30% for a concentration of 200 mg L−1). These observations confirm that UiO-67-TzTz can be used as an effective DCF remover with high efficiency for concentrations up to 50 mg L−1. At present, DCF concentrations monitored in wastewaters or environment are much lower than this value (about 1–2 mg L−1).65,66 Therefore, UiO-67-TzTz would be exploitable for real water samples remediation.
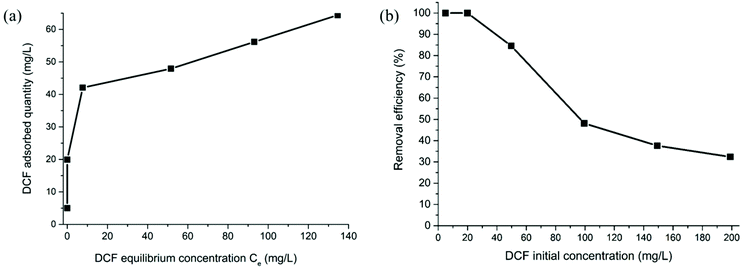 |
| Fig. 7 (a) DCF adsorption isotherm of UiO-67-TzTz; (b) removal efficiency as a function of DCF initial concentration. | |
The data fitting according to a Langmuir model (Fig. S14†) shows a good correlation (R2 = 0.98), indicating that adsorption takes place according to this model; other MOFs showed a similar behaviour in adsorption experiments.67,68 From the fitting, the maximum adsorption capacity (Xm) was calculated as 62.5 mg g−1. This value is lower than that found in variably funtionalized UiO-66-type MOFs (between 106 and 263 mg g−1; BET specific surface areas between 900 and 1000 m2 g−1),67 in Zr–BTC (MOF-808; BTC3− = benzene-1,3,5-tricarboxylate; Xm = 833 mg g−1; BET specific surface area = 1517 m2 g−1)69 and in a zirconium MIXMOF containing open metal sites (PCN-134; Xm ∼650 mg g−1; BET surface area = 756 m2 g−1).70 Nonetheless, the Xm value found is comparable or even higher than that measured for other commercially available porous materials such as activated carbon or carbon nanotubes (76 and 7.5 mg g−1, respectively).67,71 For the sake of completeness, desorption tests were performed for selected DCF concentrations; the results are reported in Table 1. The values collected in Table 1 show that the amount of desorbed DCF increases with increasing the initial concentration of the pollutant in the aqueous solution; however, even for the highest concentration essayed, desorption is lower than 20%. Such low desorption values seem to indicate the formation of relatively strong host–guest interactions, possibly triggered by the highly polar nature of the host (namely, the bithiazole linker) and the guest (DCF), as already highlighted above.67 To the best of our knowledge, there are few studies on pollutants desorption from MOFs. In most of these studies, the adsorbents are simply regenerated by guest exchange, i.e. through extensive washing with solvents,72 while desorption is not thoroughly investigated.
Table 1 Percentage of DCF desorbed from the [DCF@UiO-67-TzTz] system for different initial concentrations
[DCF]0 (mg L−1) |
% |
100 |
10.7 |
150 |
16.9 |
200 |
18.0 |
The adsorption experiments were performed by simply dissolving diclofenac sodium in water; under these conditions, the solution is slightly acidic (pH between 5 and 6). At this pH, the carboxylic groups are almost completely deprotonated [pKa(diclofenac) ∼4]. Further lowering the pH leads to DCF complete protonation followed by precipitation, as the acid is much less soluble in water than the corresponding sodium salt.73 Therefore, performing adsorption experiments at pH lower than 5 is unviable. On the other hand, in highly basic solutions UiO-67-TzTz is not stable, as found for its parent analogue UiO-67.74 Reasonably, the strongly nucleophilic OH− anions tend to replace carboxylates in the zirconium coordination sphere, leading to the MOF framework collapse.
Conclusions
SALE of the biphenyl dicarboxylate linker in UiO-67 with its bithiophene or bithiazole analogues provides the MIXMOFs UiO-67-TpTp and UiO-67-TzTz, featured by a 5
:
1 ratio between their carbocyclic and heterocyclic spacers. The MIXMOFs maintain the same structural properties of their parent MOF with the added value of being emitters in the blue-green visible region, with spectral features that closely resemble those of the heterocyclic linkers. The two MIXMOFs have been tested as luminescent sensors for a series of common water pollutants. UiO-67-TzTz shows a very good sensing capacity toward diclofenac sodium. In addition, this MIXMOF acts as diclofenac sodium “sponge”, with a satisfactory adsorption ability. Overall, this case of study demonstrates that luminescent MOFs can be exploited as multifunctional materials for environmental remediation. Indeed, diclofenac poses an environmental risk for surface and ground waters. Its popularity as one of the most effective non-steroidal anti-inflammatory drugs has led to its excessive consumption with concomitant increase of its concentration in wastewaters from human use, with consequent serious threats to ecosystems. Given the importance of wastewater treatments to detect and remove CECs (especially when present in small quantities), it is essential to design stable and efficient materials for this scope.
Current research activity ongoing in our laboratories is focused on the synthesis of other thiazole and thiophene-containing (MIX)MOFs to be exploited in this context.
Conflicts of interest
The authors have no conflicts of interest to declare.
Acknowledgements
G. G. thanks the Italian MIUR through the PRIN 2017 project MULTI-e (Ref. 20179337R7) “Multielectron transfer for the conversion of small molecules: an enabling technology for the chemical use of renewable energy” and the TRAINER project “Catalysts for Transition to Renewable Energy Future” (Ref. ANR-17-MPGA-0017) for financial support. C. P. thanks Fondazione con il Sud for funding the project HApECOrk (Ref. 2015-0243). S. G. and M. M. acknowledge Università degli Studi dell'Insubria for partial funding and Miss Anna Mauri for experimental help.
References
- L. Joseph, B.-M. Jun, M. Jang, C. M. Park, J. C. Muñoz-Senmache, A. J. Hernández-Maldonado, A. Heyden, M. Yu and Y. Yoon, Removal of Contaminants of Emerging Concern by Metal-Organic Framework Nanoadsorbents: A Review, Chem. Eng. J., 2019, 369, 928–946 CrossRef CAS.
- M. Patel, R. Kumar, K. Kishor, T. Mlsna, C. U. Pittman Jr. and D. Mohan, Pharmaceuticals of Emerging Concern in Aquatic Systems: Chemistry, Occurrence, Effects, and Removal Methods, Chem. Rev., 2019, 119, 3510–3673 CrossRef CAS.
- T. A. Ternes, Occurrence of Drugs in German Sewage Treatment Plants and Rivers, Water Res., 1998, 32, 3245–3260 CrossRef CAS.
- K. Kümmerer, Antibiotics in the aquatic environment – A review – Part I, Chemosphere, 2009, 75, 417–434 CrossRef.
- I. Senta, P. Kostanjevecki, I. Krizman-Matasic, S. Terzic and M. Ahel, Occurrence and Behavior of Macrolide Antibiotics in Municipal Wastewater Treatment: Possible Importance of Metabolites, Synthesis Byproducts, and Transformation Products, Environ. Sci. Technol., 2019, 53, 7463–7472 CrossRef CAS.
-
O. M. Yaghi, M. J. Kalmutzki and C. S. Diercks, Introduction to Reticular Chemistry: Metal–Organic Frameworks and Covalent Organic Frameworks, Wiley VCH Verlag GmbH & Co. KGaA, 2019. ISBN: 9783527821099 Search PubMed.
-
The Chemistry of Metal–Organic Frameworks: Synthesis, Characterization, and Applications, ed. S. Kaskel, Wiley–VCH Verlag GmbH & Co. KGaA, 2016. ISBN: 9783527693078 Search PubMed.
- C. E. Wilmer, M. Leaf, C. Y. Lee, O. K. Farha, B. G. Hauser, J. T. Hupp and R. Q. Snurr, Large-scale Screening of Hypothetical Metal–Organic Frameworks, Nat. Chem., 2012, 4, 83–89 CrossRef CAS.
- S. Rojas and P. Horcajada, Metal–Organic Frameworks for the Removal of Emerging Organic Contaminants in Water, Chem. Rev., 2020, 120, 8378–8415 CrossRef CAS PubMed.
- Y. Zhang, S. Yuan, G. Day, X. Wang, X. Yang and H.-C. Zhou, Luminescent Sensors Based on Metal-Organic Frameworks, Coord. Chem. Rev., 2018, 354, 28–45 CrossRef CAS.
- W. P. Lustig, S. Mukherjee, N. D. Rudd, A. V. Desai, J. Li and S. K. Ghosh, Metal–Organic Frameworks: Functional Luminescent and Photonic Materials for Sensing Applications, Chem. Soc. Rev., 2017, 46, 3242–3285 RSC.
- F. Yi, D. Chen, M. Wu, L. Han and H.-L. Jiang, Chemical Sensors Based on Metal–Organic Frameworks, ChemPlusChem, 2016, 81, 675–690 CrossRef CAS.
- M. D. Allendorf, C. A. Bauer, R. K. Bhakta and R. J. T. Houk, Luminescent Metal–Organic Frameworks, Chem. Rev., 2009, 38, 1330–1352 CAS.
- X. Liu, T. Liang, R. Zhang, Q. Ding, S. Wu, C. Li, Y. Lin, Y. Ye, Z. Zhong and M. Zhou, Iron-Based Metal–Organic Frameworks in Drug Delivery and Biomedicine, ACS Appl. Mater. Interfaces, 2021, 13, 9643–9655 CrossRef CAS.
- H. Bhasin and D. Mishra, Metal Organic Frameworks: A Versatile Class of Hybrid Compounds for Luminescent Detection and Adsorptive Removal of Enviromental Hazards, Comments Inorg. Chem., 2021, 41, 267–315 CrossRef CAS.
- R. Yuan and H. He, State of the Art Methods and Challenges of Luminescent Metal–Organic Frameworks for Antibiotic Detection, Inorg. Chem. Front., 2020, 7, 4293–4319 RSC.
- R. Wu, C. Bi, D. Zhang, C. Fan, L. Wang, B. Zhu, W. Liu, N. Li, X. Zhang and Y. Fan, Highly Selective, Sensitive and Stable Three-Dimensional Luminescent Metal–Organic Framework for Detecting and Removing of the Antibiotic in Aqueous Solution, Microchem. J., 2020, 159, 105349 CrossRef CAS.
- N. D. Rudd, H. Wang, E. M. A. Fuentes-Fernandez, S. J. Teat, F. Chen, G. Hall, Y. J. Chabal and J. Li, Highly Efficient Luminescent Metal-Organic Framework for the Simultaneous Detection and Removal of Heavy Metals from Water, ACS Appl. Mater. Interfaces, 2016, 8, 30294–30303 CrossRef CAS.
- C. I. C. Esteves, A. M. F. Silva, M. M. M. Raposo and S. P. G. Costa, Unnatural Benz-X-Azolyl Asparagine Derivatives as Novel Fluorescent Amino Acids: Synthesis and Photophysical Characterization, Tetrahedron, 2009, 65, 9373–9377 CrossRef CAS.
- R. M. F. Batista, S. P. G. Costa and M. M. M. Raposo, Synthesis of New Fluorescent 2-(2′,2′′-Bithienyl)-1,3-Benzothiazoles, Tetrahedron Lett., 2004, 45, 2825–2828 CrossRef CAS.
- G. Mercuri, M. Moroni, A. Fermi, G. Bergamini, S. Galli, G. Giambastiani and A. Rossin, Zirconium Metal–Organic Frameworks Containing a Biselenophene Linker: Synthesis, Characterization, and Luminescent Properties, Inorg. Chem., 2020, 59, 15832–15841 CrossRef CAS PubMed.
- L. Luconi, G. Mercuri, T. Islamoglu, A. Fermi, G. Bergamini, G. Giambastiani and A. Rossin, Benzothiazolium-Functionalized NU-1000: a Versatile Material for Carbon Dioxide Adsorption and Cyanide Luminescence Sensing, J. Mater. Chem. C, 2020, 8, 7492–7500 RSC.
- P. Müller, B. Bucior, G. Tuci, L. Luconi, J. Getzschmann, S. Kaskel, R. Q. Snurr, G. Giambastiani and A. Rossin, Computational Screening, Synthesis and Testing of Metal–Organic Frameworks with a Bithiazole Linker for Carbon Dioxide Capture and its Green Conversion into Cyclic Carbonates, Mol. Syst. Des. Eng., 2019, 4, 1000–1013 RSC.
- G. Mercuri, G. Giambastiani and A. Rossin, Thiazole- and Thiadiazole-Based Metal–Organic Frameworks and Coordination Polymers for Luminescent Applications, Inorganics, 2019, 7, 144 CrossRef CAS.
- S. Staderini, G. Tuci, L. Luconi, P. Müller, S. Kaskel, A. Eychmüller, F. Eichler, G. Giambastiani and A. Rossin, Zinc Coordination Polymers Containing Isomeric Forms of p-(Thiazolyl)benzoic Acid: Blue-Emitting Materials with a Solvatochromic Response to Water, Eur. J. Inorg. Chem., 2017, 4909–4918 CrossRef CAS.
- S. Staderini, G. Tuci, M. D'Angelantonio, F. Manoli, I. Manet, G. Giambastiani, M. Peruzzini and A. Rossin, Zinc Coordination Polymers Containing the m,-(2-thiazolyl)benzoic Acid Spacer: Synthesis, Characterization and Luminescent Properties in Aqueous Solutions, ChemistrySelect, 2016, 6, 1123–1131 CrossRef.
- G. Tuci, G. Giambastiani, S. Kwon, P. C. Stair, R. Q. Snurr and A. Rossin, Chiral Co(II) Metal–Organic Framework in the Heterogeneous Catalytic Oxidation of Alkenes under Aerobic and Anaerobic Conditions, ACS Catal., 2014, 4, 1032–1039 CrossRef CAS.
- A. Rossin, G. Tuci, G. Giambastiani and M. Peruzzini, 1D and 2D Thiazole-Based Copper(II) Coordination Polymers: Synthesis and Applications in Carbon Dioxide Capture, ChemPlusChem, 2014, 79, 406–412 CrossRef CAS.
- A. Rossin, B. Di Credico, G. Giambastiani, A. Peruzzini, G. Pescitelli, G. Reginato, E. Borfecchia, D. Gianolio, C. Lamberti and S. Bordiga, Synthesis, Characterization and CO2 Uptake of a Chiral Co(II) Metal–Organic Framework Containing a Thiazolidine-Based Spacer, J. Mater. Chem., 2012, 22, 10335–10344 RSC.
- J. H. Cavka, S. Jakobsen, U. Olsbye, N. Guillou, C. Lamberti, S. Bordiga and K. P. Lillerud, A New Zirconium Inorganic Building Brick Forming Metal Organic Frameworks with Exceptional Stability, J. Am. Chem. Soc., 2008, 130, 13850–13851 CrossRef PubMed.
- J. Li, S. Yuan, J.-S. Qin, L. Huang, R. Bose, J. Pang, P. Zhang, Z. Xiao, K. Tan, A. V. Malko, T. Cagin and H.-C. Zhou, Fluorescence Enhancement in the Solid State by Isolating Perylene Fluorophores in Metal–Organic Frameworks, ACS Appl. Mater. Interfaces, 2020, 12, 26727–26732 CrossRef CAS PubMed.
- M. J. Katz, Z. J. Brown, Y. J. Colon, P. W. Siu, K. A. Scheidt, R. Q. Snurr, J. T. Hupp and O. K. Farha, A Facile Synthesis of UiO-66, UiO-67 and Their Derivatives, Chem. Commun., 2013, 49, 9449–9451 RSC.
- M. Yoon and D. Moon, New Zr(IV) Based Metal-Organic Framework Comprising a Sulfur-Containing Ligand: Enhancement of CO2 and H2 Storage Capacity, Microporous Mesoporous Mater., 2015, 215, 116–122 CrossRef CAS.
- S. Øien, D. Wragg, H. Reinsch, S. Svelle, S. Bordiga, C. Lamberti and K. P. Lillerud, Detailed Structure Analysis of Atomic Positions and Defects in Zirconium Metal–Organic Frameworks, Cryst. Growth Des., 2014, 14, 5370–5372 CrossRef.
- A. Le Bail, Whole Powder Pattern Decomposition Methods and Applications: A Retrospection, Powder Diffr., 2005, 20, 316–326 CrossRef CAS.
-
Topas, V. 3.0, Bruker AXS, Karlsruhe, Germany, 2005 Search PubMed.
- Bond distances and angles for the rigid body describing: (a) the ligands: PhPh2− aromatic C–C, 1.39 Å; TzTz2− aromatic C–C and C–N, 1.39 Å; C–S, 1.75 Å; single C–C, 1.54 Å; carboxylic C–O, 1.25 Å; C–H, 0.95 Å; PhPh2− C–C–C internal bond angles, 60.0°; C–C–C and C–C–H external bond angles, 120.0°; TzTz2− C–C–C and C–C–S internal bond angles, 113.2° and 112.1°, respectively; C–C–H external bond angles, 123.4°; C–C–C external bond angles, 128.0°; (b) the DMF molecule: C
O, 1.25 Å; C–N, 1.35 Å; C–H, 0.95 Å; bond angles for sp2 and sp3, atoms, 120.0° and 109.5°, respectively.
- A. A. Coelho, Whole-Profile Structure Solution from Powder Diffraction using Simulated Annealing, J. Appl. Crystallogr., 2000, 33, 899–908 CrossRef CAS.
- R. W. Cheary and A. A. Coelho, A Fundamental Parameters Approach to X-Ray Line-Profile Fitting, J. Appl. Crystallogr., 1992, 25, 109–121 CrossRef CAS.
-
IUCr Monograph N. 5, ed. R. A. Young, Oxford University Press, 1981. ISBN: 0-19-855912-7 Search PubMed.
- D. A. Gómez-Gualdrón, P. Z. Moghadam, J. T. Hupp, O. K. Farha and R. Q. Snurr, Application of Consistency Criteria To Calculate BET Areas of Micro-And Mesoporous Metal–Organic Frameworks, J. Am. Chem. Soc., 2016, 138, 215–224 CrossRef.
-
J. Rouquerol, P. Llewellyn and F. Rouquerol, in Studies in Surface Science and Catalysis, ed. P. L. Llewellyn, F. Rodriquez-Reinoso, J. Rouquerol and N. Seaton, Elsevier, Amsterdam, 2007, vol. 160, p. 49 Search PubMed.
- W. B. Zhong, R. X. Li, J. Ly, T. He, M. M. Xu, B. Wang, L. H. Xie and J. R. Li, Two Isomeric In(III)-MOFs: Unexpected Stability Difference and Selective Fluorescence Detection of Fluoroquinolone Antibiotics in Water, Inorg. Chem. Front., 2020, 7, 1161–1171 RSC.
- Z. Yang, Y. Gu, B. Yuan, Y. Tian, J. Shang, D. C. W. Tsang, M. Liu, L. Gan, S. Mao and L. Li, Thio-Groups Decorated Covalent Triazine Frameworks for Selective Mercury Removal, J. Hazard. Mater., 2021, 403, 123702 CrossRef CAS.
- W. Bury, D. Fairen-Jimenez, M. B. Lalonde, R. Q. Snurr, O. K. Farha and J. T. Hupp, Control over Catenation in Pillared Paddlewheel Metal–Organic Framework Materials via Solvent-Assisted Linker Exchange, Chem. Mater., 2013, 25, 739–744 CrossRef CAS.
- T. Li, M. T. Kozlowski, E. A. Doud, M. N. Blakely and N. L. Rosi, Stepwise Ligand Exchange for the Preparation of a Family of Mesoporous MOFs, J. Am. Chem. Soc., 2013, 135, 11688–11691 CrossRef CAS PubMed.
- M. Kim, J. F. Cahill, Y. Su, K. A. Prather and S. M. Cohen, Postsynthetic Ligand Exchange as a Route to Functionalization of ‘Inert’ Metal–Organic Frameworks, Chem. Sci., 2012, 3, 126–130 RSC.
- S. Chavan, J. G. Vitillo, D. Gianolio, O. Zavorotynska, B. Civalleri, S. Jakobsen, M. H. Nilsen, L. Valenzano, C. Lamberti, K. P. Lillerud and S. Bordiga, H2 Storage in Isostructural UiO-67 and UiO-66 MOFs, Phys. Chem. Chem. Phys., 2012, 14, 1614–1626 RSC.
-
K. Nakamoto, Infrared and Raman Spectra of Inorganic and Coordination Compounds, Wiley Press, NJ, 6th edn, 2009. ISBN: 9780470405840 Search PubMed.
- J. Cornelio, T.-Y. Zhou, A. Alkaş and S. G. Telfer, Systematic Tuning of the Luminescence Output of Multicomponent Metal–Organic Frameworks, J. Am. Chem. Soc., 2018, 140, 15470–15476 CrossRef CAS PubMed.
- M. Taddei, D. Tiana, N. Casati, J. A. van Bokhoven, B. Smit and M. Ranocchiari, Mixed-linker UiO-66: Structure–Property Relationships Revealed by a Combination of High-Resolution Powder X-Ray Diffraction and Density Functional Theory Calculations, Phys. Chem. Chem. Phys., 2017, 19, 1551–1559 RSC.
- X. Kong, H. Deng, F. Yan, J. Kim, J. A. Swisher, B. Smit, O. M. Yaghi and J. A. Reimer, Mapping of Functional Groups in Metal-Organic Frameworks, Science, 2013, 341, 882–885 CrossRef CAS PubMed.
- A search in the Cambridge Structural Database (v.2021) for single-crystal structures determined at ambient conditions containing eight-coordinated ZrIV ions yielded 1.930 Å < d(Zr–O) < 2.584 Å, with an average value of 2.192(63) Å.
- The diameter of the cages was estimated by measuring the distance among the nearest atoms of ligands belonging to opposite cage walls and subtracting the van der Waals radii of the two atoms.
- A. L. Spek, Structure Validation in Chemical Crystallography, Acta Crystallogr., Sect. D: Biol. Crystallogr., 2009, 65, 148–155 CrossRef CAS PubMed.
- The decomposition temperatures of the bare H2TzTz and H2TpTp linkers have been assessed through an independent TGA-MS experiment.
- N. Ko, J. Hong, S. Sung, K. E. Cordova, H. J. Park, J. K. Yang and J. Kim, A Significant Enhancement of Water Vapour Uptake at Low Pressure by Amine-Functionalization of UiO-67, Dalton Trans., 2015, 44, 2047–2051 RSC.
- X. Y. Liu, W. P. Lustig and J. Li, Functionalizing Luminescent Metal–Organic Frameworks for Enhanced Photoluminescence, ACS Energy Lett., 2020, 5, 2671–2680 CrossRef CAS.
- V. Glembockyte, M. Frenette, A. M. Durantini, J. Gostick, V. Strukil, T. Frisic and G. Cosa, Highly Photostable and Fluorescent Microporous Solids Prepared via Solid-State Entrapment of Boron Dipyrromethene Dyes in a Nascent Metal–Organic Framework, J. Am. Chem. Soc., 2018, 140, 16882–16887 CrossRef CAS.
- J. Wang, Q. Zha, G. Qin and Y. Ni, A Novel Zn(II)-Based Metal-Organic Framework as a High Selective Sensor for Fluorescent Detections of Aromatic Nitrophenols and Antibiotic Metronidazole, Talanta, 2020, 211, 120742 CrossRef CAS.
- M. Kozlowska, P. Rodziewicz, T. Utesch, M. A. Mroginski and A. Kaczmarek-Kedziera, Solvation of Diclofenac in Water from Atomistic Molecular Dynamics Simulations – Interplay between Solute–Solute and Solute–Solvent Interactions, Phys. Chem. Chem. Phys., 2018, 20, 8629–8639 RSC.
- L. Zhao, J. Liu, L. Zhang, Y. Gao, Z. Zhang and Y. Luan, Self-Assembly Properties, Aggregation Behaviour and Prospective Application for Sustained Drug Delivery of a Drug-Participating Catanionic System, Int. J. Pharm., 2013, 452, 108–115 CrossRef CAS.
- P. Prakash, A. Sayyed-Ahmad, Y. Zhou, D. E. Volk, D. G. Gorenstein, E. Dial, L. M. Lichtenberger and A. A. Gorfe, Aggregation Behavior of Ibuprofen, Cholic Acid and Dodecylphosphocholine Micelles, Biochim. Biophys. Acta, 2012, 1818, 3040–3047 CrossRef CAS PubMed.
- P. Tudja, M. Zarhiul, I. Khan, E. Meštrovic, M. Horvat and P. Golja, Thermal Behaviour of Diclofenac Sodium: Decomposition and Melting Characteristics, Chem. Pharm. Bull., 2001, 49, 1245–1250 CrossRef CAS.
- H. Zindi, L. Mondamert, Q. Blancart Remaury, A. Cleon, N. Karpel Vel Leitner and J. Labanowski, Occurrence of Carbamazepine, Diclofenac, and their Related Metabolites and Transformation Products in a French Aquatic Environment and Preliminary Risk Assessment, Water Res., 2021, 196, 117052 CrossRef PubMed.
- O. Solaun, J. Germán-Rodríguez, I. Menchaca, E. López-García, E. Martínez, B. Zonja, C. Postigo, M. López de Alda, D. Barceló, A. Borja, A. Manzanos and J. Larreta, Contaminants of Emerging Concern in the Basque Coast (N Spain): Occurrence and Risk Assessment for a Better Monitoring and Management Decision, Sci. Total Environ., 2021, 765, 142765 CrossRef CAS.
- Z. Hasan, N. A. Khan and S. H. Jhung, Adsorptive Removal of Diclofenac Sodium from Water with Zr-Based Metal-Organic Frameworks, Chem. Eng. J., 2016, 284, 1406–1413 CrossRef CAS.
- P. W. Seo, B. N. Bhadra, I. Ahmed, N. A. Khan and S. H. Jhung, Adsorptive Removal of Pharmaceuticals and Personal Care Products from Water with Functionalized Metal-organic Frameworks: Remarkable Adsorbents with Hydrogen-bonding Abilities, Sci. Rep., 2016, 6, 34462 CrossRef CAS PubMed.
- N. Prasetya and K. Li, MOF-808 and its Hollow Fibre Adsorbents for Efficient Diclofenac Removal, Chem. Eng. J., 2021, 417, 129216 CrossRef CAS.
- Y. Gao, J. Xia, D. Liu, R. Kang, G. Yu and S. Deng, Synthesis of Mixed-Linker Zr-MOFs for Emerging Contaminant Adsorption and Photodegradation under Visible Light, Chem. Eng. J., 2019, 378, 122118 CrossRef CAS.
- A. Gil, L. Santamaria and S. A. Korili, Removal of Caffeine and Diclofenac from Aqueous Solution by Adsorption on Multiwalled Carbon Nanotubes, Colloid Interface Sci. Commun., 2018, 22, 25–28 CrossRef CAS.
- B. M. Jun, J. Heo, C. M. Park and Y. Yoon, Comprehensive Evaluation of the Removal Mechanism of Carbamazepine and Ibuprofen by Metal Organic Framework, Chemosphere, 2019, 235, 527–537 CrossRef CAS PubMed.
- T. Yoshikawa, J. Oki, N. Ichikawa, S. Yamashita and K. Sugano, Small Differences in Acidic pH Condition Significantly Affect Dissolution Equivalence between Drug Products of Acidic Drug Salt, J. Drug Delivery Sci. Technol., 2021, 63, 102546 CrossRef CAS.
- Y. Bai, Y. Dou, L. H. Xie, W. Rutledge, J. R. Li and H.-C. Zhou, Zr-Based Metal-Organic Frameworks: Design, Synthesis, Structure, and Applications, Chem. Soc. Rev., 2016, 45, 2327–2367 RSC.
Footnote |
† Electronic supplementary information (ESI) available: Ligand quantification through 1H NMR signal integration; IR spectra of UiO-67-TpTp·DMF and UiO-67-TzTz·DMF; PXRD final structure refinement for UiO-67-TzTz·DMF; 1H NMR monitoring of the SALE process in DMF-d7 solution; water stability assessment for UiO-67-TpTp·DMF and UiO-67-TzTz·DMF through PXRD; X-ray fluorescence spectra of UiO-67-TzTz·DMF and [DCF@UiO-67-TzTz]; additional luminescence data and fitting curves. CCDC 2103802. For ESI and crystallographic data in CIF or other electronic format see DOI: 10.1039/d1qi01184g |
|
This journal is © the Partner Organisations 2022 |
Click here to see how this site uses Cookies. View our privacy policy here.