DOI:
10.1039/D2QI00816E
(Research Article)
Inorg. Chem. Front., 2022,
9, 4232-4243
Organic–inorganic hybrid phosphite-participating S-shaped penta-CeIII-incorporated tellurotungstate as an electrochemical enzymatic hydrogen peroxide sensor for β-D-glucose detection†
Received
15th April 2022
, Accepted 22nd June 2022
First published on 24th June 2022
Abstract
Polyoxometalate chemistry has made rapid advances in innovative structural chemistry. The lower valence state and lone electron pair effect of the subgroup-valence heteroatom Te(IV) can be introduced into tungsten–oxygen systems to construct complicated tellurotungstate (TT) aggregates. Thus, for the first time, we synthesized a phosphite-participating S-shaped penta-CeIII-incorporated tetrameric TT aggregate, Na4K3H6[Ce5W4(Hpica)6H2P2O12(H2O)4]{[B-β-TeW7O28]2[B-β-TeW8O30]2}·94H2O (1) (Hpica = 2-picolinic acid). The molecular structure of 1 consists of one tetrameric [Ce5W4(Hpica)6H2P2O12(H2O)4]{[B-β-TeW7O28]2[B-β-TeW8O30]2}13− (1a) hybrid polyanion constructed from two symmetrical sandwich-like {[Ce2W2(Hpica)3 HPIIIO6(H2O)2][B-β-TeW7O28][B-β-TeW8O30]}8− ({Ce2W2PIIITe2}) moieties linked by a Ce3+ cation. Interestingly, the sandwich-like {Ce2W2PIIITe2} moiety can be viewed as a heterometal cluster [Ce2W2(Hpica)3HPIIIO6(H2O)2]10+{Ce2W2PIII} group integrating a tetravacant [B-β-TeW8O30]8− fragment and a pentavacant [B-β-TeW7O28]10− fragment. Furthermore, 1 was converted into nano-1 with the help of ultrasonication in organic solution. Nano-1 was then complexed with NH2-graphene (NH2-G), and a bi-component nano-1/NH2-G nanocomposite was prepared. Without further assembly and modification, the as-prepared nano-1/NH2-G nanocomposite was first used as a sensor to detect hydrogen peroxide. This nano-1/NH2-G-based H2O2 sensor shows excellent reproducibility, stability, and anti-interference ability. In quick succession, with the assistance of glucose oxide, an electrochemical enzymatic method based on the nano-1/NH2-G-based H2O2 sensor was developed and further applied for the specific detection of β-D-glucose. This enzymatic sensor also displays good detection performance. The present work provides new insight into electrochemical sensors based on the nano-1/NH2-G nanocomposite and their potential application in enzymatic glucose sensing.
Introduction
The development of synthetic strategies has promoted important advances in the design of complex cluster architectures such as polyoxometalates (POMs).1–8 These metal–oxygen clusters with unmatched physical and chemical properties not only represent a tremendous range of crystalline inorganic clusters but also have been employed as fundamental building blocks to construct novel gigantic cluster-based functional materials with special electronic characteristics,9–12 nano-sized structures,13–16 and unique photoelectric or magnetic properties.17–21
In the realm of POMs, heteropolyoxotungstates (HPOTs) are of great importance due to their high structural stability, diverse vacant building blocks and multiple application potentials, and have been extensively used and systematically explored for novel structural design purposes and application considerations.22–25 Due to the lower valence state and lone electron pair effect of the subgroup-valence heteroatom Te(IV) with a triangular pyramidal coordination configuration, it has come to be considered an important member to generate lacunary HPOTs, known as tellurotungstates (TTs), which can be further employed to capture lanthanide (Ln) ions to fabricate high-nuclearity Ln-incorporated TTs (HNLITTs). The introduction of the Te(IV) heteroatom can enhance the feasibility of obtaining excellent HNLITTs with amazing structural features. For instance, an outstanding octameric HNLITT [{(TeO3)W10O34}8{Ce8(H2O)20}(WO2)4(W4O12)]48− (Fig. S1a†) was obtained through a versatile one-step approach by Su's group,26 which was constructed by eight {(TeO3)W9Ce} segments and other bridging {WO6} groups. Later, they also reported a larger HNLITT, [Ce10Te8W88O298(OH)12(H2O)40]18− (Fig. S1b†), which is different from the former and consists of two triangle {W2O5Ce(H2O)7} linking units and two [Te4W42O144(OH)6Ce4(H2O)13]14− fragments.27 Three years later, Hu et al. reported a tetrameric HNLITT, [{Ln(H2O)5(TeW18O64)}4]44− (Ln = Eu3+, Gd3+) (Fig. S1c†), as the first HNLITT containing four Dawson-type building blocks.28 Importantly, TT segments can also function as good inorganic mono- or polydentate ligands to combine with Ln cations in the participation of N/O-included organic components to construct organic–inorganic hybrid HNLITTs (OIHHNLITTs). At present, OIHHNLITTs, as an important branch of HNLITTs, are gradually becoming a research hotpot due to their diverse components, eye-catching topologies and broad application prospects. For example, in 2017, a series of Hpica-decorated tetrameric OIHHNLITTs, [Ln2(H2O)4(pica)2W2O5][(Ln(H2O)W2(Hpica)2O4)(B-β-TeW8O30H2)2]24− (Ln = La3+, Ce3+, Nd3+, Sm3+, and Eu3+) (Fig. S1d†), was isolated by our group,29 which is made up of two Ln–W-heterometal sandwiched [(Ln(H2O)W2(Hpica)2O4)(B-β-TeW8O30H2)2]5− moieties linked via one {Ln2(H2O)4(pica)2W2O5}6+ cluster. Later, the introduction of the flexible ligand gluconic acid into the system led to the formation of a multi-D-gluconic-acid-bridging OIHHNLITT [Eu4(H2O)4W6(H2glu)4O12(B-α-TeW9 O33)4]24− (Fig. S1e†), which is the first polyhydroxycarboxylic-bridging HNLITT.30 This tetrameric polyanion can be viewed as the aggregation of an innovative [Eu4(H2O)4W6(H2glu)4O12]8+ core and four [B-α-TeW9O33]8− fragments. These studies open the door to the design of new-type OIHHNLITTs with amazing structure features. However, all the above-mentioned OIHHNLITTs are almost totally made up of the trivacant [TeW9O33]8− building blocks, limiting structural innovation and breakthrough in the design of novel structures.
To further break the bottleneck in the development of the above-mentioned OIHHNLITTs, another phosphite [HPO3]2− anion was chosen to introduce in the reaction system. Because the phosphite anion is composed of one H and three O atoms, it presents an approximate tetrahedral geometry. Although coexisting triangular pyramidal TeO32− and tetrahedral HPO32− groups in the reaction system may be competitive, they also open up more possibilities for reactions and construction of structures. If the tetrahedral [HPO3]2− anion can be incorporated into OIHHNLITTs, it will be conducive to introduce more Ln ions in these structures, which greatly enriches the types of OIHHNLITT structures and promotes the innovation and sustainable development of HPOTs. Based on these ideas, an Hpica-functionalized phosphite-participating S-shaped HNLITT, Na4K3H6 [Ce5W4(Hpica)6H2P2O12(H2O)4]{[B-β-TeW7O28]2[B-β-TeW8O30]2}·94H2O (1), was synthesized. It is noteworthy that the [HPO3]2− anionic units in this structure act as bridging functional groups, connecting two Ce3+ cations and two pentavacant [B-β-TeW7O28]10− segments. Furthermore, 1 was further prepared as nano-1 with the aid of ultrasonication in organic solution. Moreover, nano-1 was further modified with NH2-graphene (NH2-G), resulting in the formation of a bi-component nano-1/NH2-G nano-composite. Subsequently, the as-prepared nano-1/NH2-G nano-composite was used as a sensor for the detection of hydrogen peroxide. Furthermore, with the assistance of glucose oxidase (GOD), an electrochemical enzymatic sensor was created based on the nano-1/NH2-G-based H2O2 sensor for the specific detection of β-D-glucose in NaH2PO4–Na2HPO4 phosphate buffer (pH = 6.5). The present work provides new insight into electrochemical sensors based on the nano-1/NH2-G nanocomposite and their potential application in enzymatic GOD sensing.
Results and discussion
Synthesis of 1
Na2WO4·2H2O (3.402 g, 10.314 mmol), dimethylamine hydrochloride (0.701 g, 8.601 mmol), K2TeO3 (0.130 g, 0.512 mmol) and H3PO3 (5.000 mL, 0.01 g mL−1) were dissolved in distilled water (20 mL). The pH value was adjusted to 5 using 6 M HCl. The solution was heated to 60 °C for 30 min. Ce(NO3)3·6H2O (0.402 g, 0.923 mmol) and Hpica (0.201 g, 1.203 mmol) were consecutively added to the solution and the final pH of the solution was kept at 5 using 4.0 M NaOH. The solution was heated at 90 °C for 2 h, cooled and filtered. Slow evaporation led to yellow block crystals after about one week. Yield: 11.8% (based on K2TeO3). Elemental analysis (calcd %): C, 3.68 (3.52); H, 1.68 (1.92); N, 0.65 (0.68); P, 0.47 (0.50); Na, 0.66 (0.75); K, 0.86 (0.95); Ce, 6.0 (5.70); Te, 4.26 (4.15); W, 51.20 (50.85). In the synthetic process, the effects of the amount (0.691, 0.923, 1.152, and 1.382 mmol) of Ce(NO3)3·6H2O and pH value (2.00, 3.00, 4.00, 5.00, and 6.00) on the crystal growth were explored. Firstly, with an increase in the Ce(NO3)3·6H2O dosage from 0.691 mmol to 0.923 mmol, the growth rate of the crystals was accelerated, but an amorphous precipitate was formed when the Ce(NO3)3·6H2O dosage was greater than 0.923 mmol. The best-quality crystals were obtained when the Ce(NO3)3·6H2O dosage was 0.923 mmol. Moreover, when the pH was 2 or 6, an amorphous precipitate was produced. 1 was isolated in the pH range of 3–5. However, when the pH was 3 or 4, crystals of 1 were formed, accompanied by some precipitate. When the pH was 5, only crystals of 1 were obtained and no precipitate was observed. Therefore, the optimum pH is 5 for crystal growth of 1.
Structural description
1 belongs to the monoclinic space group C2/c (Table S1†), where the results were also certified by IR spectroscopy (Fig. S2†) and PXRD (Fig. S3†). Its structure consists of a novel [Ce5W4(Hpica)6H2P2O12 (H2O)4]{[B-β-TeW7O28]2[B-β-TeW8O30]2}13− (1a) hybrid polyanion (Fig. 1a), four Na+, three K+, six H+ and eighteen crystal water molecules. The S-shaped 1a anion can be further viewed as two sandwich-like {[Ce2W2(Hpica)3 HPIIIO6(H2O)2][B-β-TeW7O28][B-β-TeW8O30]}8− ({Ce2W2PIIITe2}) (Fig. 1b and d) moieties linked by a Ce33+ cation. The Ce33+ ion located at the symmetrical center exhibits a twisted square antiprism (Fig. 1c), where four coordination oxygen atoms (O16, O32, O39, and O64) on the same plane come from the [B-β-TeW7O28]10− subunit [Ce3–O16: 2.500 Å, Ce3–O32: 2.447 Å, Ce3–O39: 2.480 Å and Ce3–O64: 2.415 Å]. Furthermore, the sandwich-like {Ce2W2PIIITe2} moiety could be deemed as one tetralacunary [B-β-Te1W8O30]8− (Fig. 1e) subunit and a pentalacunary [B-β-Te2W7O28]10− (Fig. 1f) subunit connected by a heterometallic [Ce2W2(Hpica)3HPIIIO6(H2O)2]10+{Ce2W2PIII} (Fig. 1g) cluster. The [B-β-Te1W8O30]8− (Fig. 1e) subunit stems from the [B-β-Te1W9O33]8− fragment (Fig. 1h) by losing a {WO6} octahedron in the {W3O13} trimetallic cluster. Similarly, the [B-β-Te2W7O28]11− (Fig. 1f) subunit can be regarded as being obtained by the trivacant [B-β-Te2W9O33]8− (Fig. 1h) fragment, losing two {WO6} octahedra in two different trimetallic clusters, one of which is the rotated 60° trimetallic cluster, resulting from the α configuration to the β configuration, whereas the other is a trimetallic cluster that links to another trimetallic cluster through a corner-sharing motif. In the {Ce2W2PIII} cluster, a twisted quadrilateral is formed by Ce1, Ce2, W4 and W5 atoms through four bridging oxygen atoms (Fig. 1i). The dihedral angle between the surface formed by the Ce1, W4, and W5 atoms and the surface defined by the Ce2, W4, and W5 atoms is 103.4° (Fig. 1j). From another point of view, 1a can be considered as a combination of two [B-β-Te1W8O30]8− (Fig. 1e) subunits, two heterometallic {Ce2W2PIII} (Fig. 1g) clusters and a 1
:
2-type {Ce3[B-β-Te2W7O28]2}17− fragment (Fig. 1k). This 1
:
2-type {Ce3[B-β-Te2W7O28]2}17− fragment can be further simplified to the simple snippets (Fig. 1l), and the half of the simple snippet (Fig. 1l) can be seen as a bowl formed by oxygen atoms on the edge of the pentavacant Keggin [B-β-TeW7O28]10− subunit (Fig. 1m). It is worth mentioning that three 2-Hpica ligands adopt two different coordination environments in the {Ce2W2PIII} cluster (Fig. 2a). However, there is no 2-Hpica ligand in the Ce13+ cation. The Ce13+ cation occupies the eight-coordinate distorted square antiprism established by one water ligand (O1W), three O (O11, O17 and O44) atoms from the tetravacant [B-β-Te1W8O30]8− segment and two O atoms (O4 and O14) from the pentavacant [B-β-Te2W7O28]10− segment, and one μ2-O7 atom and one μ2-O21 atom (Fig. 2b). The Ce23+ cation also adopts a distorted square antiprism geometry (Fig. 2c), in which the N2 and O56 atoms are from the same 2-Hpica ligand and μ2-O20 is from the {HPIIIO3} group (Fig. 2d). The remaining coordination atoms are O2 W, μ2-O23, μ2-O31, O35 and O52, respectively, where the O35 and O52 atoms are from the tetravacant [B-β-Te1W8O30]8− subunit. The other two 2-Hpica ligands coordinate with the W4 and W5 atoms via a carboxyl oxygen atom and a pyridine nitrogen atom, respectively, producing two five-membered rings, [W5–O29–C18–C13–N3 (Fig. 2e) and W4–O15–C6–C1–N1 (Fig. 2f)].
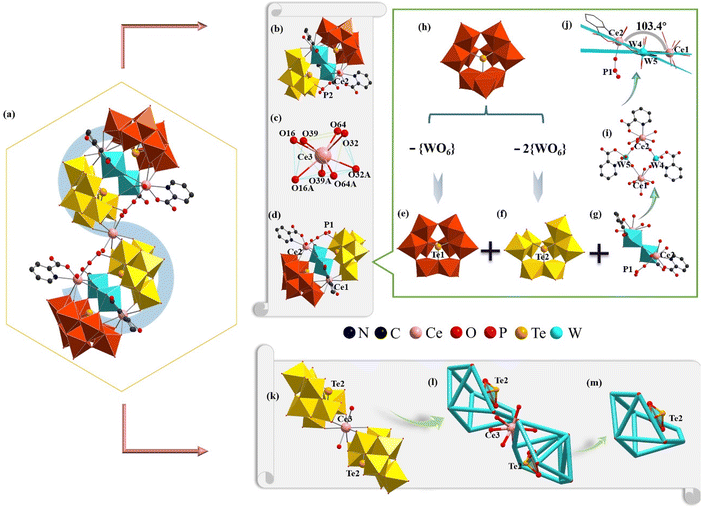 |
| Fig. 1 (a) S-shaped 1a anion. (b and d) Sandwich-like {Ce2W2PIIITe2} moiety. (c) Coordination environment of Ce33+. (e) Tetravacant [B-β-TeW8O30]8− subunit. (f) Pentavacant [B-β-TeW7O28]10− subunit. (g) The {Ce2W2PIII} cluster. (h) Trivacant [B-β-TeW9O33]8− fragment. (i) Twisted parallelogram formed by Ce1, Ce2, W4 and W5 atoms. (j) The surface defined by W4, W5, Ce13+ and Ce23+ atoms. (k) The 1 : 2 type {Ce3[B-β-Te2W7O28]2}17− fragment. (l) Simplified view of the 1 : 2 type {Ce3[B-β-Te2W7O28]2}17− fragment. (m) Simplified view of the pentavacant [B-β-TeW7O28]10− subunit. Color code: W, turquoise; Te, light orange; O, red; P, pink; Ce, rose; C, black; N, blue; {TeW8}, brick red; {TeW7}, gold. | |
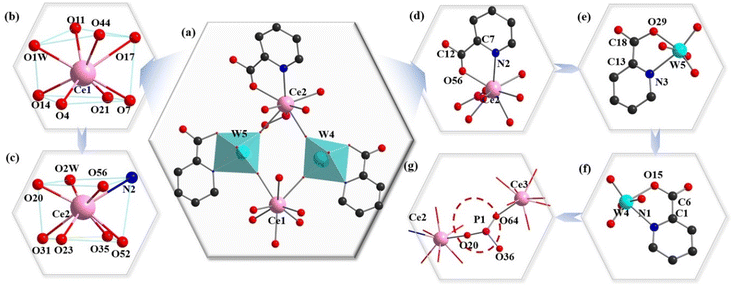 |
| Fig. 2 (a) The [Ce2W2(Hpica)3O4(H2O)2]10+ connectome. (b and c) Square antiprism geometries of Ce13+ and Ce23+ ions. (d–f) Coordination environments of the Ce23+, W5 and W4 centers, respectively. (g) Connection of the {HPIIIO3} group and Ce23+ and Ce33+ ions. Color codes: W, turquoise; Te, light orange; O, red; P, pink; Ce, rose; C, black; N, blue; {TeW8}, brick red; {TeW7}, gold. | |
The W4 and W5 atoms display the octahedral configuration (Fig. 2a). This coordination mode and type of 2-Hpica greatly improve the stability of 1a. It is surprising that the tetrahedral {HPIIIO3} group (Fig. 2g) (this proton is omitted) links Ce23+, Ce33+ and [B-β-TeW7O28]10−. The tetrameric 1a is the first example of a phosphite-participating OIHHNLITT. This type of HNLITT with high-vacancy mixed building TT blocks is very rare in the system of POM structures, which may be related to the higher pH synthetic conditions and offers a new idea for preparing HPOT-based materials in the future.
To maintain charge balance and enhance the structural stability of 1a, some charge compensation ions (Na+ and K+) and lattice water molecules are required, which interact with 1a through electrostatic and H-bond interactions to give birth to the 3-D supramolecular architecture. To better comprehend the stacking motifs, each 1a anion is simplified as two diagonally connected triangles along the a, b and c axes (Fig. 3a–h). In the bc plane (Fig. 3a–d), the tetrameric 1a anions present the –ABAB– stacking pattern. To easily observe the stacking pattern of 1a anions, it was split into layers A (Fig. 3b) and B (Fig. 3c). The simplification of the –ABAB– stacking mode of 1a anions is further shown in Fig. 4d on the bc plane. From the perspective of the b axis (Fig. 3e and f), the 1a anions are distributed in an –AAA– fashion, while the Ce atoms of each 1a anions are arranged in an Arabic number “8” pattern (Fig. 3f). Additionally, in the ab plane (Fig. 3g and h), the 1a anions present a more complex stacking pattern. Specifically, the 1a anions are placed in an –ABAB– pattern along the a axis and in the –ABCD– stacking pattern along the b axis. However, a special stacking mode is formed among adjacent 1a anions. Each 1a anion is surrounded by three polyanions. Through this staggered mode, every six 1a anions form a lantern-shaped hexagon (Fig. 3h). In addition, it is worth mentioning that 1 shows high stability up to 300 °C in an N2 atmosphere (Fig. S4†), which provides a foundation for its further application and functionalization.
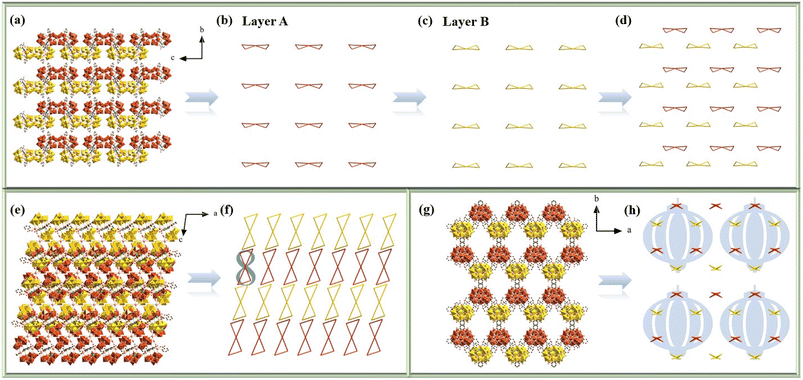 |
| Fig. 3 (a) 3D stacking of 1a anions in the bc plane. (b and c) Simplified layer A and layer B, displaying two spatial orientations of 1a anions in the bc plane. (d) Simplified stacking of 1a anions in the bc plane. (e and f) 3D stacking and simplified stacking of 1a anions in the ac plane. (g and h) 3D stacking and simplified stacking of 1a anions in the ab plane. | |
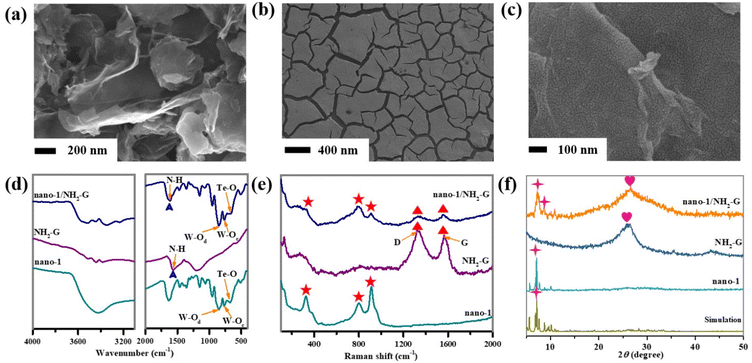 |
| Fig. 4 (a–c) SEM images of NH2-G, nano-1 and nano-1/NH2-G. (d) IR spectra, (e) Raman spectra and (f) PXRD patterns of simulation of 1, nano-1, NH2-G, and nano-1/NH2-G. | |
Design strategy and characterization of nano-1 and nano-1/NH2-G composite
The crystal structure of nano-1 obtained by ultrasonication is consistent with that of 1, which can be confirmed by the good agreement of the IR spectra (Fig. S2†) and PXRD patterns (Fig. S3†) of 1 and nano-1. The process for the fabrication of the nano-1/NH2-G composite is schematically represented in Scheme 1a–c. As is known, the introduction of an active substrate possessing a high-specific surface area and electroconductibility is a useful method to promote the electrochemical application of POM-based materials.31,32NH2-G is an ideal conductive substrate due to its low cost, high electrical conductivity, amino-functionalized surface and huge surface area. Also, NH2-G possesses abundant active sites to combine with POMs based on supramolecular interactions and electrostatic attraction. Thus, NH2-G acts as a conductive substrate. Alternatively, nano-POMs can work as functional precursors to produce composite materials owing to the exposure of the abundant active sites of nanosized POMs with stable morphologies.33,34 Here, nano-1 was utilized as a precursor to composite with NH2-G to prepare the nano-1/NH2-G composite. The above-mentioned preparation strategy has the advantages of NH2-G and nano-POMs, compensating for the disadvantage of the single species and adapting to the requirements of electrochemical sensors. Considering the superior properties and prospective potential of nanosized POMs, nano-1 was prepared with the help of ultrasonication in EtOH solution. The UV reflection spectrum (Fig. S5†) of nano-1 was applied to obtain its optical bandgap (2.88 eV) using the Tauc plot method, which proved that it is an optical semiconductor. Specifically, the effective combination of semiconductor nano-1 and highly electrically conductive NH2-G can facilitate electron transfer and improve the sensing performance of the nano-1/NH2-G composite. To find the optimal proportion of nano-1/NH2-G, nano-1/NH2-G composites with proportions of 0.5
:
1, 1
:
1, 1.5
:
1, 2
:
1 and 2.5
:
1 were prepared and their successful preparation was also proved by IR spectra (Fig. S6a†) and PXRD patterns (Fig. S6b†). Through CV measurements (Fig. S7†) of nano-1/NH2-G modified glassy carbon electrodes (GCEs) with different proportions of nano-1/NH2-G in 0.1 M PBS (pH = 6.5) with 6.0 mM H2O2, it could be distinctly found that the CV peak current of nano-1/NH2-G-GCE is the strongest when the proportion of nano-1/NH2-G is 1
:
1. Therefore, the optimal proportion of nano-1/NH2-G was determined to be 1
:
1. Furthermore, SEM was used to characterize the morphologies of the as-synthesized materials (NH2-G
:
nano-1 = 1
:
1). Fig. 4a illustrates the leaflike nanosheet morphology of NH2-G with a smooth surface. The morphology of nano-1 shows a cracked film (Fig. 4b), presenting the successful construction of the nano-1 film, which was strongly supported by SEM-EDS elemental mapping analysis (Fig. S8†). The nano-1/NH2-G nanomorphology is exhibited in Fig. 4c, in which the smooth surface of NH2-G is fully covered by the cracked film of nano-1, verifying the successfully preparation of the nano-1/NH2-G composite. It is obvious that the ultrathin nanosheet morphology of NH2-G was maintained, which acts as the soft highly electrically conducting support of nano-1.
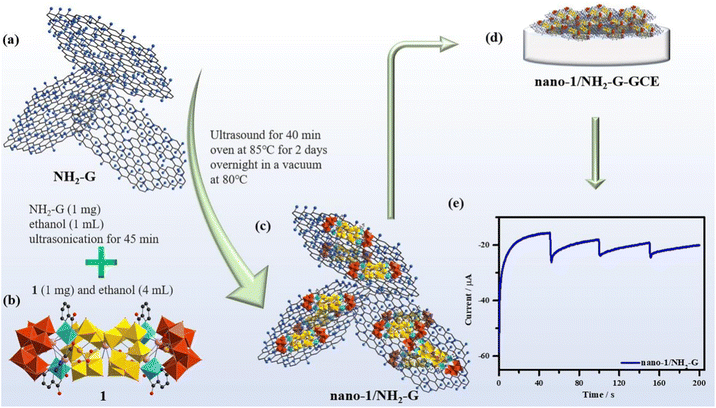 |
| Scheme 1 Schematic preparation process of nano-1/NH2-G-GCE. (a) Schematic structure of NH2-G. (b) Crystal structure of 1. (c) Schematic structure of nano-1/NH2-G. (d) The nano-1/NH2-G-GCE. (e) Schematic detection curve. | |
To confirm the structures of all the nanosized samples, the IR spectra, Raman spectra and PXRD patterns of nano-1, NH2-G and nano-1/NH2-G were measured. The functional groups of nano-1, NH2-G and nano-1/NH2-G were observed in their FT-IR spectra (Fig. 4d). Three groups of characteristic peaks at 852, 759 and 667 cm−1 were observed for nano-1, which correspond to the ν(W–Od), ν(W–Oc) and ν(Te–Oa) stretching vibrations, respectively. In the spectrum of NH2-G, the intensity of the signals at 3437 and 1571 cm−1 was greatly reduced. The signal at 1571 cm−1 is attributed to the N–H bond. These observations indicate the successful preparation of nano-1/NH2-G.35 Furthermore, as revealed in Fig. 4e, the characteristic Raman peaks for nano-1 were observed at 324 (W–O), 793 (Te–O), 920 (W–O) and 966 (P–O) cm−1 (Fig. S9†), whereas the main Raman peaks for NH2-G were seen at 1328 and 1568 cm−1, which are consistent with that in the literature.36 For nano-1/NH2-G, its Raman peaks (330, 791, 915, 1330, and 1563 cm−1) are very similar to that of each of its component. Moreover, the crystalline structures of nano-1, NH2-G and nano-1/NH2-G were further characterized by PXRD tests (Fig. 4f). For nano-1, its PXRD peaks match well with its single-crystal XRD mode (Fig. S2†). The PXRD peaks of NH2-G match that in the literature.36 For nano-1/NH2-G, its PXRD peaks are made up of that of nano-1 and NH2-G. In addition, all the samples showed good crystallinity. Meanwhile, the elemental composition of nano-1/NH2-G was investigated by SEM-EDS mapping measurements. As shown in Fig. 5, the SEM-EDS elemental mapping data of nano-1/NH2-G confirm the existence and a homogeneous distribution of C (Fig. 5b), W (Fig. 5c), O (Fig. 5d), N (Fig. 5e), P (Fig. 5f), Te (Fig. 5g) and Ce (Fig. 5h) elements, further verifying the successful composition of nano-1 and NH2-G.
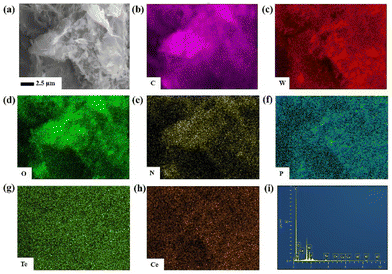 |
| Fig. 5 (a) SEM image of nano-1/NH2-G at the 2.5 μm scale. (b–h) SEM-EDS C, W, O, N, P, Te and Ce elemental mapping images of nano-1/NH2-G on a silicon substrate. (i) EDS of nano-1/NH2-G. | |
Electrochemical properties of the nano-1/NH2-G-based biosensor
H2O2 is closely involved in many important physiological and biochemical reactions in organisms.37,38 H2O2 is produced to mediate a variety of physiological responses, including cell proliferation, differentiation and migration. In addition, H2O2 also plays an important role in food processing, disinfection and drug production.39,40 In the dairy industry, H2O2 is widely used as a preservative to prevent spoilage of dairy products and extend their shelf life.41 However, excessive H2O2 can lead to neurodegenerative diseases, cancer, diabetes and other health problems.42,43 Therefore, the accurate measurement of the H2O2 concentration is very important. The traditional methods for the determination of H2O2 include colorimetry, fluorescence, flow injection and electrochemical luminescence.44,45 However, the applications of these methods are limited by their complex and time-consuming procedures and expensive equipment. Hence, the development of economical, efficient and sensitive methods for the detection of H2O2 is vital. According to the literature,46–48 the electrochemical method has the advantages of quick response, simple operation, high sensitivity and good selectivity.
POMs can be used as an electronic container that can receive or lose one or more electrons.49 This, the application of the catalytic activity and biocompatibility of POMs in electrochemical sensing has aroused the interest of researchers. Previously, some investigations on POM-based sensors have been reported for the electrochemical sensing of myricetin,50 clenbuterol and ractopamine,51 cholesterol,52 dopamine34 and uric acid.53 At present, in the related research on electrochemical sensors, the introduction of carbon nanomaterials in POM systems endows them with some better electrochemical performances such as improved stability and reproducibility, a wider detection range, lower detection limit and good selectivity.54 Among the carbon materials, NH2-G bearing good electroconductibility and biocompatibility can accelerate electron transfer to improve the signal intensity. Also, the positively charged NH2-G can be combined with the negatively charged POMs by electrostatic attractions. Based on these considerations, the nano-1/NH2-G composite was utilized to modify GCE (Scheme 1d and e) for further electrochemically detecting H2O2.
Fig. 6a depicts the cyclic voltammetry (CV) curves of GCE (dotted line), NH2-G-GCE (dash line) and nano-1/NH2-G-GCE (solid line) in 0.1 M PBS (pH = 6.5) in the presence (navy blue) or absence (pink) of 6.0 mM H2O2 (scan rate 100 mV s−1) in the potential window of −0.5–0.6 V. Apparently, when H2O2 was present, only nano-1/NH2-G-GCE showed a dramatic increase in current response at about −0.3 V, which is derived from the reduction procedure of tungsten atoms in nano-1, indicating the effective electrocatalytic reduction of H2O2 by nano-1/NH2-G-GCE. This observation indicates that nano-1/NH2-G-GCE could immensely enhance the electroconductibility and catalytic capability by rapid electron transfer. In contrast, the bare GCE did not display a variation in current intensity in the presence or absence of H2O2, demonstrating almost no electrocatalytic reduction activity for H2O2.
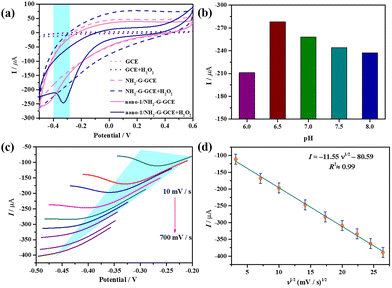 |
| Fig. 6 (a) CV curves of GCE, NH2-G-GCE and nano-1/NH2-G-GCE in 0.1 M PBS (pH = 6.5) with 6.0 mM H2O2. (b) Evolution of cathode peak current derived from W centers in nano-1 with pH for nano-1/NH2-G-GCE in 0.1 M PBS (pH = 6.5) with 6.0 mM H2O2. (c) CV curves of nano-1/NH2-G-GCE in 0.1 M PBS (pH = 6.5) with 6.0 mM H2O2 at various scan rates. (d) Plot of cathode peak current derived from the W centers in nano-1vs. the square root of the scan rate. | |
Afterwards, to explore the optimum H2O2 detection conditions, CV measurements of nano-1/NH2-G-GCE under different pH values in 0.1 M PBS with 6.0 mM H2O2 (scan rate 100 mV s−1) were performed (Fig. 6b and Fig. S10†). The results indicate that the catalytic activity was affected by pH. The optimal pH was found to be 6.5. In addition, the CV curves of nano-1/NH2-G-GCE under various scan rates were also studied. The influence of scan rate (ν) on the electrocatalytic reduction of H2O2 was evaluated in the range of 10–700 mV s−1 (Fig. 6c and Fig. S11†). It is glaringly obvious that the cathode peak current is proportional to the square root of the scan rate, which is fitted using the equation I = −11.55 (ν)1/2 − 80.59 (R2 = 0.99) (Fig. 6d). This phenomenon manifests that the electrochemical interface reaction between nano-1/NH2-G-GCE and solution is a diffusion-controlled process.55
A suitable working potential is crucial for the electrochemical detection of H2O2. This is because a too low working potential will decrease the sensitivity of the sensor to effectively detect H2O2. As presented in Fig. 7a, the amperometric response curves (ARCs) were measured under different working potentials in 0.1 M PBS (pH = 6.5) with 6.0 mM H2O2, which indicate that the response current gradually decreased when the potential increased in the range of −0.30–0 V. Obviously, the maximum response current appeared at the potential of −0.20 V, and consequently −0.20 V was chosen as the best potential for the detection of H2O2. Consequently, the potential of −0.20 V was applied for testing the ARCs of nano-1/NH2-G-GCE by successively adding H2O2 to 0.1 M PBS (pH = 6.5). To exclude the effect of the baseline current under different applied potentials, the amperometric current response values under different potentials are provided in Fig. 7b, further confirming that the optimal potential is −0.20 V. To quantitatively probe the determination property of nano-1/NH2-G-GCE, the amperometric current response changes were recorded with the continuous addition of different concentrations of H2O2 (0.1–5.9 mM) to 0.1 M PBS (pH = 6.5) at −0.2 V (Fig. 7c). A noteworthy variation in the response current was observed after each addition of H2O2. When the concentration of H2O2 was less than 5.9 mM, nano-1/NH2-G-GCE generated a rapid response signal, revealing that nano-1/NH2-G-GCE has high sensitivity for the detection of H2O2 in the range of 0.1–5.9 mM. Fig. 7d reveals the plot of the response current versus H2O2 concentration (0.1–5.9 mM), which obeys the function I = −6.7cH2O2 − 1.3 with a correlation coefficient of 0.99. The reproducibility of the as-prepared nano-1/NH2-G-GCE as an H2O2 sensor was also tested. Herein, six independent nano-1/NH2-G-GCEs were tested in 0.1 M PBS (pH = 6.5) solution with 6.0 mM H2O2 at −0.20 V (Fig. 7e). Their relative standard deviation (RSD) was 1.2%, demonstrating the outstanding reproducibility of nano-1/NH2-G-GCEs for the detection of H2O2. These results demonstrate that nano-1/NH2-G-GCE is a good electrochemical sensor for the detection of H2O2, which may be derived from the synergistic effect of nano-1 and NH2-G.
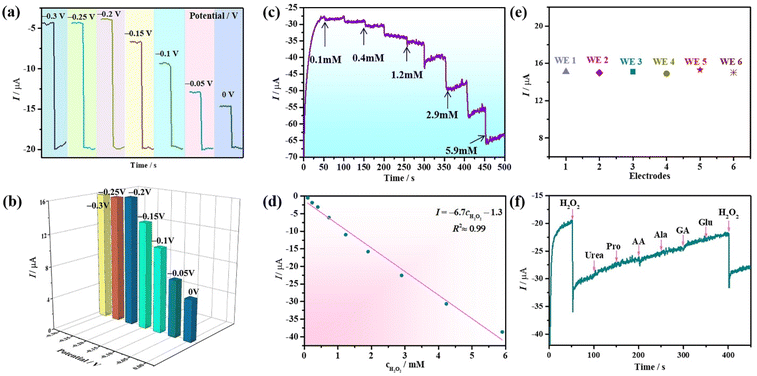 |
| Fig. 7 (a) ARCs of nano-1/NH2-G-GCE under different applied potentials in 0.1 M PBS (pH = 6.5) with 6.0 mM H2O2. (b) Comparison of amperometric responses (I) at different potentials to obtain the optimal applied potential. (c) ARC of nano-1/NH2-G-GCE with the successive addition of diverse concentrations of H2O2 in 0.1 M PBS (pH = 6.5) at −0.20 V. (d) Plot of I vs. cH2O2. (e) Comparison of peak currents of six independent nano-1/NH2-G-GCEs in 0.1 M PBS (pH = 6.5) with 6.0 mM H2O2 at −0.20 V. (f) ARC of nano-1/NH2-G-GCE in 0.1 M PBS (pH = 6.5) with the successive addition of 1.0 mM of H2O2 and 1.0 mM interferents (urea, Pro, AA, Ala, GA, and glu) in 0.1 M PBS (pH = 6.5) at −0.20 V. | |
Furthermore, antijamming capability, reproducibility and stability are vital parameters affecting the sensing properties. The antijamming capability immediately influences the precision of recognition. Some interferents in the real environment readily generate interference signals in the detection of H2O2. Hence, several common interferents such as urea, proline (Pro), ascorbic acid (AA), glucose (Glu), alanine (Ala) and gluconic acid (GA) were selected to evaluate the antijamming capability of nano-1/NH2-G-GCE. Fig. 7f shows ARC of nano-1/NH2-G-GCE after the successive addition of 1.0 mM urea, 1.0 mM Pro, 1.0 mM AA, 1.0 mM Ala, 1.0 mM GA, 1.0 mM Glu, and 1.0 mM H2O2 in 0.1 M PBS (pH = 6.5). Obviously, the addition of urea, Pro, AA, Ala, GA, and Glu produced a negligible current response compared to H2O2, manifesting the preeminent anti-interference ability and selectivity for nano-1/NH2-G-GCE in the detection of H2O2. In addition, a time-dependent stability test on nano-1/NH2-G-GCE was also carried out. After one week of storage at 4 °C, the current response of nano-1/NH2-G-GCE still maintained 93% of the initial value (Fig. S12†), illustrating its good time-dependent stability.
Electrochemical glucose detection based on nano-1/NH2-G-GCE H2O2 biosensor
Although the first enzyme electrode was invented in 1962,56 much more endeavours have been dedicated to enhancing blood glucose monitoring techniques in the past five decades. Most commercial glucose monitors utilize the enzyme-based electrochemical method. GOD is a commonly used enzyme, which can specifically catalyze β-D-glucose (β-D-Glu) under aerobic conditions. The optimum working temperature of GOD is 0–30 °C and the best pH is 5.5–6.5.57 The optimum pH of nano-1/NH2-G-GCE in this work is also in this range. Therefore, an enzyme electrochemical glucose sensing system was designed (Fig. 8). 10 mg GOD, 0.1 g β-D-Glu and 10 mg GOD + 0.1 g β-D-Glu were added to 2.0 mL PBS (0.1 M, pH 6.5), respectively, and incubated at 30 °C for 30 min. Then, 100 μL of these solutions were injected into 15.0 mL PBS (0.1 M, pH = 6.5), respectively, and their current response measured. As illustrated in Fig. 8, the nano-1/NH2-G-GCE biosensor showed little or no significant amperometric current response for only the presence of GOD or β-D-Glu in 0.1 M PBS (pH = 6.5) at −0.2 V. In contrast, the GOD + β-D-Glu system for the nano-1/NH2-G-GCE biosensor exhibited a significant amperometric current response. The enzyme-catalysed reaction is as follows:
β-D-Glu + oxygen + H2O + GOD → gluconic acid + H2O2. |
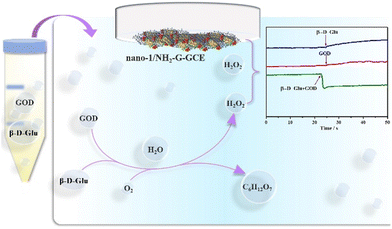 |
| Fig. 8 Schematic enzyme electrochemical glucose sensing system and ARCs of the nano-1/NH2-G-GCE sensor with the addition of β-D-Glu, GOD and β-D-Glu + GOD in 0.1 M PBS (pH = 6.5) at −0.20 V. | |
Thus, with the help of GOD, β-D-Glu can be detected by detecting H2O2 using our nano-1/NH2-G-GCE biosensor.58 To explore the effect of heating time of β-D-Glu and GOD on the current response, we chose four time points to perform the experiments. As shown in Fig. 9, the heating time was the best at 30 min. After 30 min, the current response decreased slightly with an increase in time. This may be due to the decomposition of trace H2O2. Therefore, we chose the current response value to calculate the concentration of H2O2 produced by the reaction at a heating time of 30 min. Using the measured the current response value in the above-mentioned equation I = −6.7cH2O2 − 1.3 led to the concentration of produced H2O2 being 250.74 mM in the reaction system consisting of 10 mg GOD and 0.1 g β-D-Glu. This result reveals that 250.74 mM β-D-Glu was detected, while the actual added β-D-Glu concentration was 277.7 mM, and thus the relative error was 9.7%. Therefore, this electrochemical enzymatic method can effectively detect β-D-Glu.
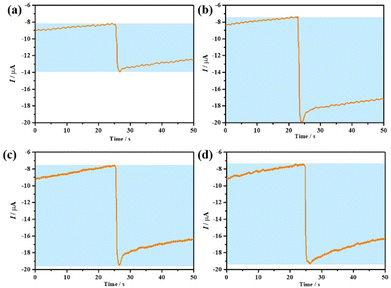 |
| Fig. 9 (a–d) ARCs of nano-1/NH2-G-GCE towards a β-D-Glu + GOD solution at heating times of 0, 30, 60 and 90 min, respectively. The tests were carried out in 0.1 M PBS (pH = 6.5) at −0.20 V. | |
Conclusions
In conclusion, the introduction of the tetrahedral [HPO3]2− anion in the OIHHNLITT system led to the formation of the fascinating Hpica-functionalized phosphite-participating S-shaped HNLITT 1. This result indicates that the [HPO3]2− anion can be used as an anion template to tune the structural assembly of HNLITTs, demonstrating a feasible synthetic route to generate new HPOTs with different types of heteroatoms. In addition, the nano-1/NH2-G composite was successfully prepared using a simple method, which then was used to develop an electrochemical sensor platform for the detection of H2O2 and β-D-Glu, respectively. The electrochemical studies showed that this HNLITT may be a good candidate for developing promising electrochemical sensing applications.
Author contributions
Nizi Song and Yanzhou Li performed syntheses, characterization, electrochemical properties and wrote the manuscript. Yanying Wang, Menglu Wang participated in PXRD, IR and TG characterization. Lijuan Chen and Junwei Zhao provided research ideas, determined crystal structures and revised all over the manuscript.
Conflicts of interest
There are no conflicts to declare.
Acknowledgements
This work was supported by the National Natural Science Foundation of China (21871077, 22071042, 22001058, 22171070, 21771052), the Program for Innovation Teams in Science and Technology in Universities of Henan Province (20IRTSTHN004), and the Program of First-Class Discipline Cultivation Project of Henan University (2019YLZDYJ02, CJ1205A0240019).
References
- C. F. Li, K. Yamaguchi and K. Suzuki, Ligand-directed approach in polyoxometalate synthesis: formation of a new divacant lacunary polyoxomolybdate [γ-PMo10O36]7−, Angew. Chem., Int. Ed., 2021, 60, 6960–6964 CrossRef CAS PubMed.
- L. L. Liu, J. Jiang, G. P. Liu, X. D. Jia, J. W. Zhao, L. J. Chen and P. Yang, Hexameric to trimeric lanthanide-included selenotungstates and their 2D honeycomb organic−inorganic hybrid films used for detecting ochratoxin A, ACS Appl. Mater. Interfaces, 2021, 13, 35997–36010 CrossRef CAS PubMed.
- Z. H. Pan, Z. Z. Weng, X. J. Kong, L. S. Long and L. S. Zheng, Lanthanide-containing clusters for catalytic water splitting and CO2 conversion, Coord. Chem. Rev., 2022, 457, 214419 CrossRef CAS.
- X. Chen, Z. Wang, R. Zhang, L. Xu and D. Sun, A novel polyoxometalate-based hybrid containing a 2D [CoMo8O26]∞ structure as the anode for lithium-ion batteries, Chem. Commun., 2017, 53, 10560–10563 RSC.
- S. Omwoma, C. T. Gore, Y. C. Ji, C. W. Hu and Y. F. Song, Environmentally benign polyoxometalate materials, Coord. Chem. Rev., 2015, 286, 17–29 CrossRef CAS.
- X. F. Yi, N. V. Lzarova, M. Stuckart, D. Guerin, L. Thomas, S. Lenfant, D. Vuillaume, J. V. Leusen, T. Duchoň, S. Nemšák, S. D. M. Bourone, S. Schmitz and P. Kögerler, Probing frontier orbital energies of {Co9(P2W15)3} polyoxometalate clusters at molecule-metal and molecule-water interfaces, J. Am. Chem. Soc., 2017, 139, 14501–14510 CrossRef CAS PubMed.
- T. Wang, T. Ji, W. L. Chen, X. H. Li, W. Guan, Y. Geng, X. L. Wang, Y. G. Li and Z. H. Kang, Polyoxometalate film simultaneously converts multiple low-value all-weather environmental energy to electricity, Nano Energy, 2020, 68, 104349 CrossRef CAS.
- A. Bijelic, A. Manuel and A. Rompel, Polyoxometalates as potential next-generation metallodrugs in the combat against cancer, Angew. Chem., Int. Ed., 2019, 58, 2980–2999 CrossRef CAS PubMed.
- S. R. Li, H. Y. Wang, H. F. Su, H. J. Chen, M. H. Du, L. S. Long, X. J. Kong and L. S. Zheng, A giant 3d-4f polyoxometalate super-tetrahedron with high proton conductivity, Small Methods, 2021, 5, 2000777 CrossRef CAS PubMed.
- B. Huang, D. H. Yang and B. H. Han, Application of polyoxometalate derivatives in rechargeable batteries, J. Mater. Chem. A, 2020, 8, 4593–4628 RSC.
- L. B. Ni, G. Yang, Y. Liu, Z. Wu, Z. Y. Ma, C. Shen, Z. X. Lv, Q. Wang, X. X. Gong, J. Xie, G. W. Diao and Y. G. Wei, Self-assembled polyoxometalate nanodots as bidirectional cluster catalysts for polysulfide/sulfide redox conversion in lithium-sulfur batteries, ACS Nano, 2021, 15, 12222–12236 CrossRef CAS PubMed.
- L. Y. Wu, L. Y. Guo, H. F. Su, M. Jagodič, M. Luo, X. Q. Zhou, S. Y. Zeng, C. H. Tung, D. Sun and L. S. Zheng, Two unprecedented POM-based inorganic-organic hybrids with concomitant heteropolytungstate and molybdate, Inorg. Chem., 2017, 56, 2481–2489 CrossRef PubMed.
- S. J. Li, Y. Zhao, S. Knoll, R. J. Liu, G. Li, Q. P. Peng, P. T. Qiu, D. F. He, C. Streb and X. N. Chen, High proton-conductivity in covalently Linked polyoxometalate organoboronic acid-polymers, Angew. Chem., Int. Ed., 2021, 60, 16953–16957 CrossRef CAS PubMed.
- L.-Y. Guo, M. Jagodič, S.-Y. Zeng, Z. Wang, Z.-Q. Shi, X.-P. Wang, C.-H. Tung and D. Sun, pH-Controlled assembly of two novel Dawson-sandwiched clusters involving the in situ reorganization of trivacant α-[P2W15O56]12− into divacant α-[P2W16O57]8−, Dalton Trans., 2016, 45, 8404–8411 RSC.
- J. C. Liu, J. F. Wang, Q. Han, P. Shangguan, L. L. Liu, L. J. Chen, J. W. Zhao, C. Streb and Y. F. Song, Multicomponent self-assembly of a giant heterometallic polyoxotungstate supercluster with antitumor activity, Angew. Chem., Int. Ed., 2021, 60, 11153–11157 CrossRef CAS PubMed.
- S. S. Zhang, J. Y. Chen, K. Li, J. D. Yuan, H. F. Su, Z. Wang, M. Kurmoo, Y. Z. Li, Z. Y. Gao, C. H. Tung, D. Sun and L. S. Zheng, Janus cluster: asymmetric coverage of a Ag43 cluster on the symmetric Preyssler P5W30 polyoxometalate, Chem. Mater., 2021, 33, 9708–9714 CrossRef CAS.
- K. Yang, Y. Y. Hu, L. Y. Li, L. L. Cui, L. He, S. J. Wang, J. W. Zhao and Y. F. Song, First high-nuclearity mixed-valence polyoxometalate with hierarchical interconnected Zn2+ migration channels as an advanced cathode material in aqueous zinc-ion battery, Nano Energy, 2020, 74, 104851 CrossRef CAS.
- C. Zou, Z. J. Zhang, X. Xu, Q. H. Gong, J. Li and C. D. Wu, A multifunctional organic-inorganic hybrid structure based on MnIII-porphyrin and polyoxometalate as a highly effective dye scavenger and heterogenous catalyst, J. Am. Chem. Soc., 2012, 134, 87–90 CrossRef CAS PubMed.
- W. J. Luo, J. Hu, H. L. Diao, B. Schwarz, C. Streb and Y. F. Song, Robust polyoxometalate/nickel foam composite electrodes for sustained electrochemical oxygen evolution at high pH, Angew. Chem., Int. Ed., 2017, 56, 4941–4944 CrossRef CAS.
- S. Y. Lai, K. H. Ng, C. K. Cheng, H. Nur, M. Nurhadi and M. Arumugam, Photocatalytic remediation of organic waste over Keggin-based polyoxometalate materials: A review, Chemosphere, 2021, 263, 128244 CrossRef CAS.
- Y. J. Dong, Q. Han, Q. Y. Hu, C. J. Xu, C. Z. Dong, Y. Peng, Y. Ding and Y. Q. Lan, Carbon quantum dots enriching molecular nickel polyoxometalate over CdS semiconductor for photocatalytic water splitting, Appl. Catal., B, 2021, 293, 120214 CrossRef CAS.
- S. T. Zheng, J. Zhang and G. Y. Yang, Designed synthesis of POM-organic frameworks from {Ni6PW9} building blocks under hydrothermal conditions, Angew. Chem., Int. Ed., 2008, 47, 3909–3913 CrossRef CAS PubMed.
- D. Wang, J. Jiang, M. Y. Cao, S. S. Xie, Y. M. Li, L. J. Chen, J. W. Zhao and G. Y. Yang, An unprecedented dumbbell-shaped pentadeca-nuclear W–Er heterometal cluster stabilizing nanoscale hexameric arsenotungstate aggregate and electrochemical sensing properties of its conductive hybrid film-modified electrode, Nano Res., 2022, 15, 3628–3637 CrossRef CAS.
- A. J. Surman, P. J. Robbins, J. Ujma, Q. Zheng, P. E. Barran and L. Cronin, Sizing and discovery of nanosized polyoxometalate clusters by mass spectrometry, J. Am. Chem. Soc., 2016, 138, 3824–3830 CrossRef CAS PubMed.
- K. Yonesato, S. Yamazoe, D. Yokogawa, K. Yamaguchi and K. Suzuki, A molecular hybrid of an atomically precise silver nanocluster and polyoxometalates for H2 cleavage into protons and electrons, Angew. Chem., Int. Ed., 2021, 60, 16994–16998 CrossRef CAS PubMed.
- W. C. Chen, H. L. Li, X. L. Wang, K. Z. Shao, Z. M. Su and E. B. Wang, Assembly of cerium(III)-stabilized polyoxotungstate nanoclusters with SeO32−/TeO32− templates: from single polyoxoanions to inorganic hollow spheres in dilute solution, Chem. – Eur. J., 2013, 19, 11007–11015 CrossRef CAS PubMed.
- W. C. Chen, C. Qin, X. L. Wang, Y. G. Li, H. Y. Zang, K. Z. Shao, Z. M. Su and E. B. Wang, Assembly of a large Cerium(III)-containing tungstotellurites(IV) nanocluster: [Ce10Te8W88O298 (OH)12(H2O)40]18−, Dalton Trans., 2015, 44, 11290–11293 RSC.
- S. X. Shang, Z. G. Lin, A. X. Yin, S. Yang, Y. N. Chi, Y. Wang, J. Dong, B. Liu, N. Zhen, C. L. Hill and C. W. Hu, Self-Assembly of Ln(III)-containing tungstotellurates(VI): correlation of structure and photoluminescence, Inorg. Chem., 2018, 57, 8831–8840 CrossRef CAS PubMed.
- Q. Han, Y. Wen, J. C. Liu, W. Zhang, L. J. Chen and J. W. Zhao, Rare-earth-incorporated tellurotungstate hybrids functionalized by 2-picolinic acid ligands: syntheses, structures, and properties, Inorg. Chem., 2017, 56, 13228–13240 CrossRef CAS PubMed.
- Y. Zhang, D. Wang, B. X. Zeng, L. J. Chen, J. W. Zhao and G. Y. Yang, An unprecedented polyhydroxycarboxylic acid ligand bridged multi-EuIII incorporated tellurotungstate and its luminescence properties, Dalton Trans., 2020, 49, 8833–8948 Search PubMed.
- J. Jiang, L. L. Liu, G. P. Liu, D. Wang, Y. Zhang, L. J. Chen and J. W. Zhao, Organic-inorganic hybrid cerium-encapsulated selenotungstate including three building blocks and its electrochemical detection of dopamine and paracetamol, Inorg. Chem., 2020, 59, 15355–15364 CrossRef CAS.
- S. S. Xie, J. Jiang, D. Wang, Z. G. Tang, R. F. Mi, L. J. Chen and J. W. Zhao, Tricarboxylic-ligand-decorated lanthanoid-inserted heteropolyoxometalates built by mixed-heteroatom-directing polyoxotungstate units: syntheses, structures, and electrochemical sensing for 17β-estradiol, Inorg. Chem., 2021, 60, 7536–7544 CrossRef CAS PubMed.
- X. R. Li, H. G. Xue and H. Pang, Facile synthesis and shape evolution of well-defined phosphotungstic acid potassium nanocrystals as a highly efficient visible-light-driven photocatalyst, Nanoscale, 2017, 9, 216–222 RSC.
- B. X. Zeng, Y. Zhang, Y. H. Chen, G. P. Liu, Y. Z. Li, L. J. Chen and J. W. Zhao, 3-D antimonotungstate framework based on 2,6-H2pdca connecting iron-cerium heterometallic Krebs-type polyoxotungstates for detecting small biomolecules, Inorg. Chem., 2021, 60, 2663–2671 CrossRef CAS PubMed.
- J. Yu, Y. Y. Zhang, H. Li, Q. J. Wan, Y. W. Li and N. J. Yang, Electrochemical properties and sensing applications of nanocarbons: A comparative study, Carbon, 2018, 129, 301–309 CrossRef CAS.
- R. Kumar, K. Jahan, R. Nagarale and A. Sharma, Nongassing long-lasting electroosmotic pump with polyanilinewrapped aminated graphene electrodes, ACS Appl. Mater. Interfaces, 2015, 7, 593–601 CrossRef CAS PubMed.
- Y. Zhao, Z. Zhang, Z. Pan and Y. Liu, Advanced bioactive nanomaterials for biomedical applications, Exploration, 2021, 1, 20210089 CrossRef.
- Z. Wu, M. M. Liu, Z. C. Liu and Y. Tian, Real-time imaging and simultaneous quantification of mitochondrial H2O2 and ATP in neurons with a single two-photon fluorescence-lifetime-based probe, J. Am. Chem. Soc., 2020, 142, 7532–7541 CrossRef CAS PubMed.
- Y. Yang, Z. T. Zeng, G. M. Zeng, D. L. Huang, R. Xiao, C. Zhang, C. Y. Zhou, W. P. Xiong, W. J. Wang, M. Cheng, W. J. Xue, H. Guo, X. Tang and D. H. He, Ti3C2 mxene/porous γ-C3N4 interfacial Schottky junction for boosting spatial charge separation in photocatalytic H2O2 production, Appl. Catal., B, 2019, 258, 117956 CrossRef CAS.
- C. Samanta, Direct synthesis of hydrogen peroxide from hydrogen and oxygen: An overview of recent developments in the process, Appl. Catal., A, 2008, 350, 133–149 CrossRef CAS.
- M. Jose, C. Martin, G. B. Brieva and L. G. F. Jose, Hydrogen peroxide synthesis: An outlook beyond the anthraquinone process, Angew. Chem., Int. Ed., 2006, 45, 6962–6984 CrossRef PubMed.
- J. X. Fan, M. Y. Peng, H. Wang, H. R. Zheng, Z. L. Liu, C. X. Li, X. N. Wang, X. H. Liu, S. X. Cheng and X. Z. Zhang, Engineered bacterial bioreactor for tumor therapy via fenton-like reaction with localized H2O2 generation, Adv. Mater., 2019, 31, 1808278 CrossRef PubMed.
- Y. Zhang, H. Yang, D. Wei, X. Zhang, J. Wang, X. Wu and J. Chang, Mitochondria-targeted nanoparticles in treatment of neurodegenerative diseases, Exploration, 2021, 1, 20210115 CrossRef.
- Z. J. Zhang, Y. B. Liu, X. H. Zhang and J. W. Liu, A cell-mimicking structure converting analog volume changes to digital colorimetric output with molecular selectivity, Nano Lett., 2017, 17, 7926–7931 CrossRef CAS PubMed.
- L. Li, Z. Y. Xing, Q. R. Tang, L. Yang, L. Dai, H. Wang, T. Yan, W. Y. Xu, H. M. Ma and Q. Wei, Enzyme-free colorimetric immunoassay for protein biomarker enabled by loading and disassembly behaviors of polydopamine nanoparticles, ACS Appl. Bio Mater., 2020, 3, 8841–8848 CrossRef CAS PubMed.
- C. L. Huang, Y. Chai, Y. F. Jiang, J. Forth, P. D. Ashby, M. M. L. Arras, K. L. Hong, G. S. Smith, P. C. Yin and T. P. Russell, The interfacial assembly of polyoxometalate nanoparticle surfactants, Nano Lett., 2018, 18, 2525–2529 CrossRef CAS PubMed.
- E. B. Bahadır and M. K. Sezgintürk, Electrochemical biosensors for hormone analyses, Biosens. Bioelectron., 2015, 68, 62–71 CrossRef PubMed.
- Z. H. Su, X. L. Xu, Y. B. Cheng, Y. M. Tan, L. T. Xiao, D. L. Tang, H. M. Jiang, X. L. Qin and H. X. Wang, Chemical pre-reduction and electro-reduction guided preparation of a porous graphene bionanocomposite for indole-3-acetic acid detection, Nanoscale, 2019, 11, 962–967 RSC.
- D. F. Chaia, J. Carlos, G. Garcíab, B. Lia, H. J. Pang, H. Y. Ma, X. M. Wang and L. C. Tan, Polyoxometalate-based metal-organic frameworks for boosting electrochemical capacitor performance, Chem. Eng. J., 2019, 373, 587–597 CrossRef.
- R. M. Xing, L. Y. Tong, X. Y. Zhao, H. L. Liu, P. T. Ma, J. W. Zhao, X. Q. Liu and S. H. Liu, Rapid and sensitive electrochemical detection of myricetin based on polyoxometalates/SnO2/gold nanoparticles ternary nanocomposite film electrode, Sens. Actuators, B, 2019, 283, 35–41 CrossRef CAS.
- L. H. Zhang, Q. W. Wang, Y. Qi, L. Li, S. T. Wang and X. H. Wang, An ultrasensitive sensor based on polyoxometalate and zirconium dioxide nanocomposites hybrids material for simultaneous detection of toxic clenbuterol and ractopamine, Sens. Actuators, B, 2019, 288, 347–355 CrossRef CAS.
- N. Thakur, M. Kumar, S. D. Adhikary, D. Mandal and T. C. Nagaiah, PVIM–Co5POM/MNC composite as a flexible electrode for the ultrasensitive and highly selective non-enzymatic electrochemical detection of cholesterol, Chem. Commun., 2019, 55, 5021–5024 RSC.
- Z. Y. Bai, C. L. Zhou, H. B. Xu, G. L. Wang, H. J. Pang and H. Y. Ma, Polyoxometalates-doped Au nanoparticles and reduced graphene oxide: A new material for the detection of uric acid in urine, Sens. Actuators, B, 2017, 243, 361–371 CrossRef CAS.
- Y. C. Ji, L. J. Huang, J. Hu, C. Streb and Y. F. Song, Polyoxometalate-functionalized nanocarbon materials for energy conversion, energy storage and sensor systems, Energy Environ. Sci., 2015, 8, 776–789 RSC.
- M. H. Yang, S. B. Hong, J. H. Yoon, D. S. Kim, S. W. Jeong, D. E. T. Yoo, J. Lee, K. G. Lee, S. J. Lee and B. G. Choi, Fabrication of flexible, redoxable, and conductive nanopillar arrays with enhanced electrochemical performance, ACS Appl. Mater. Interfaces, 2016, 8, 22220–22226 CrossRef CAS PubMed.
- L. C. Clark and C. Lyons, Electrode systems for continuous monitoring in cardiovascular surgery, Ann. N. Y. Acad. Sci., 1962, 102, 29–45 CrossRef CAS PubMed.
- A. Heller and J. Ulstrup, Detlev Müller's discovery of glucose oxidase in 1925, Anal. Chem., 2021, 93, 7148–7149 CrossRef CAS PubMed.
- X. G. Luo, J. Xia, X. Y. Jiang, M. R. Yang and S. L. Liu, Cellulose-Based strips designed based on a sensitive enzyme colorimetric assay for the low concentration of glucose detection, Anal. Chem., 2019, 91, 15461–15468 CrossRef CAS PubMed.
Footnote |
† Electronic supplementary information (ESI) available: Experimental details, PXRD, IR, TG curves, related structure and property figures and tables. Crystallographic information for 1. CCDC 2163291 (1). For ESI and crystallographic data in CIF or other electronic format see DOI: https://doi.org/10.1039/d2qi00816e |
|
This journal is © the Partner Organisations 2022 |
Click here to see how this site uses Cookies. View our privacy policy here.