DOI:
10.1039/D1RA07946H
(Paper)
RSC Adv., 2022,
12, 2310-2318
Enzymatic synthesis of 2-phenethyl acetate in water catalyzed by an immobilized acyltransferase from Mycobacterium smegmatis
Received
28th October 2021
, Accepted 7th January 2022
First published on 14th January 2022
Abstract
Although water is an ideal green solvent for organic synthesis, it is difficult for most biocatalysts to carry out transesterification reactions in water because of the reversible hydrolysis reaction. 3D structural characteristics and the microenvironment of an enzyme has an important effect on its selectivity for the transesterification reaction over the hydrolysis reaction. A novel 2-phenethyl acetate synthesis technology was developed using acyltransferase (EC 3.1.1.2) from Mycobacterium smegmatis (MsAcT) in water. Firstly, MsAcT was entrapped in a tetramethoxysilane gel network and the immobilization process of MsAcT increased its selectivity for the transesterification reaction over the hydrolysis reaction by 6.33-fold. Then, the synthesis technology of 2-phenethyl acetate using the immobilized MsAcT in water was optimized as follows: vinyl acetate was used as acyl donor, the molar ratio of vinyl acetate to 2-phenylethyl alcohol was 2
:
1, and the water content was 80% (w/w). The reaction was carried out at 40 °C for 30 min and conversion rate reached 99.17%. The immobilized MsAcT could be recycled for 10 batches. The synthesis method of 2-phenethyl acetate using MsAcT as a biocatalyst in water is a prospective green process technology.
1. Introduction
Biocatalysts are playing an increasingly important role in the field of modern industrial biotechnology. With the approaching of the fourth wave of biocatalysis, a series of versatile enzymes are mined from various metagenomics, redesigned using bioinformatics tools, and then developed; or novel enzyme functions that are not present in nature are created. Multienzyme-cascade processes are applied in vivo as part of novel artificial metabolic routes in whole cells and various cell factories are constructed. More and more synthetic technologies of bulk chemicals, including chiral alcohols and chiral amines, are shifting from traditional chemical synthesis processes to biosynthesis processes.1–4 Principles for a green chemistry future are becoming increasingly more concrete, including low toxicity, recyclable, and easily separable green solvents or solventless,5 and have been used to guide the synthesis of new organic molecules.6
The commonly used reaction media mainly include various organic solvents, ionic liquids, and deep eutectic solvents for the enzymatic synthesis reaction.7,8 The pros and cons of the above media for enzyme and enzymatic catalytic reaction include the increase of the substrate solubility, active activation on the oil–water interface, conformational damage, structural unfolding, and so on.9–12 To improve the operational stability and the tolerance of enzymes to the reaction media, much tedious work has to be done on enzymes such as chemical modification,13,14 redesign using protein engineering,15,16 or the immobilization process.7 Water is also one kind of cheap and green solvents for enzymes. Moreover, water networks in enzymes enhance binding affinity and accelerate catalytic efficiency.17–19 However, most enzymes cannot catalyze synthesis reactions in water. Lipases and proteases catalyze the hydrolysis reactions in water, and catalyze the transesterification reactions only in the systems with very low water content.20,21 However, a novel acyl transferase (EC 3.1.1.2) from Mycobacterium smegmatis (MsAcT) is reported to catalyze transesterification reactions in water.22 Acyl transferase from Mycobacterium sp. in vivo catalyzes triacylglycerol synthesis using long-chain acyl-CoA as acyl donors, which is then stored in the form of intracellular lipid droplets. Lipid body accumulation is conducive to the establishment of Mycobacterium sp. and the maintenance of a persistent tuberculosis infection.23 Moreover, acyl transferase from Mycobacterium sp. is also involved in the biosynthesis of sulfolipid in vivo, a kind of virulence factor.24 As industrial biocatalyst, MsAcT is firstly identified for its perhydrolysis activity, a kind of promiscuous activity, the reversible formation of peroxycarboxylic acids from carboxylic acids and hydrogen peroxide.25 In recent years, transesterification activity in water from MsAcT is widely used for organic synthesis. N-Acetylation of primary arylalkyl amine and various flavor esters are successfully synthesized in water using MsAcT as a biocatalyst.26–28
When the forward transesterification reaction is carried out in water, the reverse hydrolysis reaction also proceeds at the same time as the side reaction. Considering the reversibility of the transesterification reaction in water, MsAcT must display excellent selectivity for the transesterification reaction. The ratio of catalytic efficiency for the transesterification reaction over the hydrolysis reaction is an important parameter to evaluate the reaction direction and reaction rate. Multiple factors, including water content, water activity, and acyl donor, affect the ratio of catalytic efficiency for the synthesis reaction over the hydrolysis reaction.29,30 Protein engineering and immobilization technology is always used to modify (or change) the enzymes' microenvironment, which then results in the selectivity increase for the synthesis reaction (transesterification reaction) over the hydrolysis reaction.31–33 Esterase mutants with a high preference for the transesterification reaction over the hydrolysis reaction in water are obtained by redesign of its substrate-binding pocket to improve its hydrophobicity.34,35
2-Phenylethanol acetate is the colorless transparent oily liquid, insoluble in water, but soluble in ethanol and ether. 2-Phenylethyl acetate has a rose-/raspberry-like flavor and is often used as the food additive or for perfume production.27 In this research, MsAcT was immobilized using tetramethoxysilane gel, which significantly improved its selectivity for transesterification reaction over hydrolysis reaction. 2-Phenethyl acetate was then successfully synthesized with high yield using the immobilized MsAcT as a biocatalyst in water.
2. Experimental
2.1 Strain, biochemistry reagents, and chemicals
Escherichia coli BL21(DE3)/pET28a-act was constructed as described by Shu et al. and conserved in our laboratory.36 In brief, the gene sequence for the mature acyltransferase from M. smegmatis (named as act gene) was artificially synthesized by Sangon Biotech Shanghai Co. Ltd (Shanghai, China) according to the known genomic DNA sequence of M. smegmatis NCTC8159 (gene locus in the GenBank database: LN831039.1). The act gene fragment was incorporated into the Nco I/Xho I restriction site at its 5′-terminal and 3′-terminal, respectively. The Nco I/Xho I-digested act gene fragment was then ligated into the Nco I/Xho I-digested plasmid pET28a, which resulted in the recombinant plasmid pET28a-act. Plasmid pET28a-act was then transformed into E. coli BL21(DE3).
Protein markers were purchased from Takara Biotechnology Co. Ltd (Dalian, China). Isopropyl-beta-D-thiogalactopyranoside (IPTG) and kanamycin were purchased from Sangon Biotechnology Co. Ltd (Shanghai, China). HisTrap HP affinity chromatography column (5 mL) was purchased from GE Healthcare Life Sciences (Beijing, China). p-Nitrophenyl acetate (pNPA) was purchased from Alfa-Aesar Company. Tetramethoxysilane (TMOS) was purchased from WD silicone Co. Ltd (Wuhan, China). 2-Phenylethyl alcohol, acetic anhydride, ethyl acetate, vinyl acetate, and other chemicals were of analytical grade and purchased from Sinopharm Chemical reagent Co. Ltd (Shanghai, China).
2.2 Expression and purification of MsAcT
Induction expression of Escherichia coli BL21(DE3)/pET28a-act and purification of recombinant MsAcT was carried out as described by Shu et al. (2016) and Jia et al. (2020).36,37 In brief, Escherichia coli BL21(DE3)/pET28a-act was firstly inoculated to Luria–Bertani broth (containing 50 μg mL−1 kanamycin) and grown at 30 °C. IPTG was then added into the culture media to a final concentration of 1 mmol L−1 as soon as the cell density (OD600) of Escherichia coli reached 0.6. The induction cultivation continued for 16 h at 30 °C, and then the cells were harvested by centrifugation. The cell pellet from 250 mL culture broth was resuspended in the following buffer: 20 mmol L−1 NaH2PO4–Na2HPO4 (pH 8.0), 20 mmol L−1 imidazole, and 500 mmol L−1 NaCl, and then disrupted by ultrasonication. The cell lysate was centrifuged, and the supernatant was then purified using the immobilized metal-affinity chromatography (HisTrap HP, 1 mL, GE healthcare). The recombinant MsAcT was eluted with a linear gradient of 20 mL of 20–500 mmol L−1 imidazole in the same buffer with a flow rate of 0.8 mL min−1.
The homogeneity of the purified MsAcT was determined using SDS-PAGE. Protein concentration was determined using the Bradford method, in which bovine serum albumin was used as the standard.38
2.3 Immobilization of MsAcT using hydrophobic sol–gel material
Immobilization of MsAcT was carried out as described by Reetz et al., with slight modification.39,40 In brief, 1 mg recombinant MsAcT was added into a mixture of sodium fluoride aqueous (1 mol L−1, 50 μL added) and the right amount of water. The solution was shaken and TMOS (487 μL, 3 mmol) was then added. The reaction mixture was vigorously shaken for 10 s on a vortex mixer and then gently shaken by hand. Over 1 min, the mixture warmed up and then gelation occurred. The reaction vessel was left to stand closed at 4 °C for 12 h, then the white bulk gel was washed with 10 mL water and acetone, respectively. The immobilized MsAcT was dried in the air at room temperature and then grinded into a powder using a mortar and pestle.
To determine the effect of the molar ratio of total water to silane on the transesterification activity of the immobilized MsAcT, different amounts of water were added into the reaction mixtures for MsAct immobilization, and the molar ratio of total water to silane varied in the range of 5
:
1 to 17
:
1. The immobilization procedure of MsAcT was carried out as mentioned above. Protein immobilization efficiency, activity recovery, relative specific activity, and specific activity of the immobilized MsAcT were determined, respectively. The activity recovery is defined as the total transesterification activity of the immobilized MsAcT to that of the free MsAcT. The relative specific activity is defined as the specific activity for the transesterification reaction of the immobilized MsAcT to that of the free MsAcT.
2.4 Hydrolysis activity assay and kinetic constant determination of MsAcT
The hydrolysis activity of MsAcT was determined using the spectrophotometer assay method as described by Kordel et al. with slight modification, in which p-nitrophenyl acetate (pNPA) was used as substrate.41
The reaction mixture was composed of 1 mmol L−1 p-nitrophenyl acetate (pNPA) in 20 mmol L−1 Na2HPO4–NaH2PO4 buffer (pH 6.0) and 30 μL of the appropriately diluted MsAcT solution. The kinetics was detected for 5 min at 405 nm. Under the above condition used, the molar extinction coefficient (ε405) of p-nitrophenol was 2.6 × 10−3 L μmol−1 cm−1.
Kinetic constants for pNPA hydrolysis were determined as above by the spectrophotometric assay method, in which the pNPA concentration was increased from 10 μmol L−1 to 400 μmol L−1. The amount of MsAcT added was adjusted to give a linear dependence of the reaction rate to MsAcT concentration. The kinetic constants were found by fitting the initial rates to the Michaelis–Menten equation using nonlinear regression.
To determine the hydrolysis activity and kinetic constant of the immobilized MsAcT, MsAcT immobilizate was firstly suspended in 20 mmol L−1 Na2HPO4–NaH2PO4 buffer (pH 6.0) containing 4% (w/v) polyethylene glycol 1000, and the suspension of the MsAcT immobilizate was then added into the reaction system.
All reactions were carried out at 40 °C and 20 mmol L−1 Na2HPO4–NaH2PO4 (pH 6.0) buffer. One unit of the hydrolysis activity of MsAcT was defined as the amount of MsAcT that liberated 1 μmol of p-nitrophenol from p-nitrophenyl acetate per min. All measurements were carried out three times and the average value was taken.
2.5 Transesterification activity assay and kinetic constant determination of MsAcT
The transesterification activity of MsAcT was determined using the gas chromatography assay method, in which 2-phenylethyl alcohol was used as substrate.
Standard transesterification reactions were carried out in 10 mL screw cap bottles containing 1 mL of reaction volume. The reaction mixture consisted of 1 mol L−1 acetic anhydride and 100 mmol L−1 2-phenylethyl alcohol in 20 mmol L−1 Na2HPO4–NaH2PO4 buffer (pH 8.0) and 33 μL of the appropriately diluted MsAcT solution. Reaction mixtures were shaken (200 rpm) at 40 °C for 10 min, and then extracted with 894 μL n-hexane. Then, 550 μL organic phases containing 5 mmol L−1 benzyl acetate as internal standard were collected and determined by gas chromatography (Shimadzu GC 2010 Pro, People's Republic of China) equipped with the MXT-65 TG capillary column (30 m length × 0.25 mm inside diameter) and a hydrogen flame ionization detector. Furthermore, 1 μL of sample (split ratio 100
:
1) was injected into gas chromatography and nitrogen gas was used as the carrier gas. The initial oven temperature was 80 °C for 1 min and was increased at 10 °C min−1 to 190 °C. Oven temperature was maintained at 190 °C for 2 min. Afterward, the oven was heated at 20 °C min−1 to 270 °C, and then maintained for 2 min. The temperature of the injector and detector was 330 °C. Under the above gas chromatography analysis condition, the retention times for 2-phenylethyl alcohol, benzyl acetate, and 2-phenylethanol acetate were 4.022 min, 4.476 min, and 5.516 min, respectively.
Kinetic constants for the transesterification activity of MsAcT were determined as above, in which the 2-phenylethyl alcohol concentration was increased from 10 mmol L−1 to 160 mmol L−1. The kinetic constants were found by fitting the initial rates to the Michaelis–Menten equation using nonlinear regression.
To determine the transesterification activity and kinetic constant of the immobilized MsAcT, the dried MsAcT immobilizate was directly added into the reaction system.
One unit of the transesterification activity of MsAcT was defined as the amount of MsAcT that synthesized 1 μmol of phenethyl acetate per min. All measurements were assayed in triplicate and the average value was taken. Unless otherwise stated, all determined activities in this research referred to the transesterification activity.
2.6 Optimization synthesis process of 2-phenethyl acetate using the immobilized MsAcT
To determine the effect of acyl donor type and concentration of 2-phenylethyl alcohol on conversion rate, three types of acyl donor, including ethyl acetate, vinyl acetate, and acetic anhydride, and three different concentrations of 2-phenylethyl alcohol, 100 mmol L−1, 250 mmol L−1 and 500 mmol L−1, were used for the transesterification reaction, respectively. The total volume of the reaction mixture was 1 mL. The reaction system consisted of 1 mol L−1 acyl donor, the gel powder containing 0.1 mg MsAcT protein and a certain concentration of 2-phenylethyl alcohol in 20 mmol L−1 Na2HPO4–NaH2PO4 (pH 8.0). The reaction mixture in a 10 mL screw cap bottle was agitated using the orbital shaker (200 rpm) at 40 °C for 5 h. Sample processing and component analysis was carried out as above mentioned.
Effect of water content in the reaction system on the conversion rate of the transesterification reaction was investigated after the optimal molar ratios of acyl donor to 2-phenylethyl alcohol were obtained. In the transesterification reaction system, the amount of acyl donor was 1 mole and gel powder containing 0.1 mg MsAcT was used. The molar ratios of ethyl acetate, vinyl acetate, and acetic anhydride to 2-phenylethyl alcohol were 10
:
1, 2
:
1, and 10
:
1, respectively. According to the weight of the mixture of acyl donor and 2-phenylethyl alcohol, the suitable amount of Na2HPO4–NaH2PO4 buffer (20 mmol L−1 pH 8.0, acting as water, weight to weight) was added into the reaction system. The reaction mixture in a 10 mL screw cap bottle was agitated using the orbital shaker (200 rpm) at 40 °C for 5 h. Sample processing and component analysis was carried out as above mentioned.
2.7 Time course of 2-phenylethanol acetate enzymatic synthesis using different acyl donor
The time course of the conversion rate of 2-phenylethyl alcohol was determined under the optimal synthesis condition for every acyl donor, respectively. The standard reaction system consisted of 1 mole acyl donor and tetramethoxysilane gel powder containing 0.1 mg MsAcT. The molar ratio (mole acyl donor/mole 2-phenylethyl alcohol) of the ethyl acetate reaction system, vinyl acetate reaction system, and acetic anhydride reaction system was 10
:
1, 2
:
1, and 10
:
1, respectively. The water content of the ethyl acetate reaction system, vinyl acetate reaction system, and acetic anhydride reaction system was 60% (w/w), 80% (w/w), and 80% (w/w), respectively. The reaction mixture in a 10 mL screw cap bottle was agitated using the orbital shaker (200 rpm) at 40 °C for 5 h. Samples were taken periodically from the reaction system at 0.5 h, 1 h, 2 h, 3 h, 4 h, and 5 h, and then 2-phenylethyl acetate yield was determined using gas chromatography, respectively.
2.8 Operational stability of the immobilized MsAcT
The vinyl acetate-containing transesterification reaction system was selected to evaluate the operational stability of the immobilized MsAcT. The reaction mixture consisted of 1 mole vinyl acetate, 0.5 mole 2-phenylethyl alcohol, 80% (w/w) water content, and TMOS gel powder containing 0.1 mg MsAcT. The reaction mixture in a 10 mL screw cap bottle was agitated using the orbital shaker (200 rpm) at 40 °C for 0.5 h. The reaction mixture was centrifuged to collect the immobilized MsAcT. The immobilized MsAcT was firstly washed three times using 20 mmol L−1 Na2HPO4–NaH2PO4 buffer (pH 8.0) and then used for the next batch transesterification reaction. The supernatant was used to determine the conversion rate of 2-phenylethyl alcohol.
2.9 Statistical analysis
All experiments were carried out three times independently. Data were presented as the average ± standard deviation. The data were statistically analyzed using SPSS software and groups were compared using Student's t-test with a significant difference defined as p < 0.05.
3. Results
3.1 Purification of the recombinant MsAcT
As shown in Fig. 1, the homologous recombinant MsAcT could be directly obtained using Ni-NTA affinity chromatography column, and the activity recovery reached 72.6%. The soluble expression level of act gene in E. coli BL21 (DE3) was 270 mg L−1. The relative molecular weight was about 26 kDa, which was the same as that reported previously.22 The specific activity of the purified MsAcT for hydrolysis reaction towards p-nitrophenyl acetate was 379.6 U mg−1.
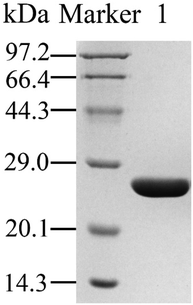 |
| Fig. 1 SDS-PAGE analysis for the purified MsAcT on a 12% separating gel. | |
3.2 MsAcT immobilization using silane sol–gel
MsAcT was entrapped into silica matrices during silane sol–gel solidification. The molar ratio (R) of total water to tetramethoxysilane (TMOS) in the gel-forming reaction mixture was an important factor influencing the transesterification activity of silane immobilized MsAcT. As shown in Fig. 2A, there were significant differences in the activity recovery, relative specific activity, and specific activity of the immobilized MsAcT among different R values. When R was increased up to 14, activity recovery, relative specific activity, and specific activity of the immobilized MsAcT reached its maximum, at 63.12 ± 2.13%, 65.85 ± 2.22%, and 300.38 ± 10.12 U g−1, respectively. However, regardless of the R value, there was no obvious negative effect on the protein immobilization efficiency, which ranged between 95% and 97%. The immobilized MsAcT powder displayed lamellar morphology using scanning electron microscope observation (Fig. 2B), which was totally different from that of spherical particles in a previous report. The formation of microsphere particles occurred when a mixture of two silane gel molecules, methyltrimethoxysilane (MTMS) and polydimethylsiloxane (PDMS) at a molar ratio of 6
:
1, was used as the precursor, and the R value was set to 6.39
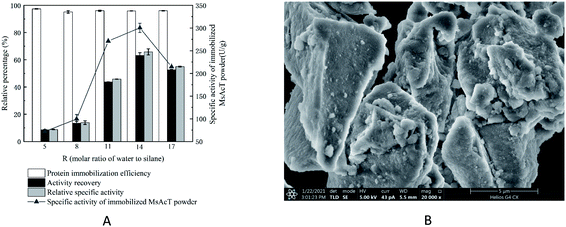 |
| Fig. 2 Immobilization process of MsAcT. (A) Effect of molar ratio of total water to tetramethoxysilane on transesterification activity of the immobilized MsAcT. (B) Scanning electron microscope micrograph of MsAcT-containing TMOS gel powder under the immobilization condition where the molar ratio of total water to tetramethoxysilane was 14 : 1. | |
3.3 Relative selectivity for transesterification reaction over hydrolysis reaction of free MsAcT and the immobilized MsAcT
Relative selectivity for the transesterification reaction over the hydrolysis reaction of free MsAcT and immobilized MsAcT were measured, respectively. Hydrolysis activity was determined using p-nitrophenyl acetate (pNPA) as substrate, and transesterification activity was determined using 2-phenethyl alcohol as acyl acceptor and acetic anhydride as acyl donor for 2-phenethyl acetate synthesis. The initial rates of hydrolysis reaction and transesterification reaction catalyzed by free MsAcT and immobilized MsAcT are shown in Fig. 3, respectively. Kinetic parameters were obtained using Prism software (Graph Pad prism 8) analysis with Michaelis–Menten non-linear regression and are shown in Table 1.
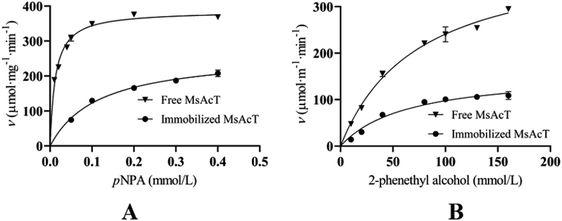 |
| Fig. 3 Initial rates of the hydrolysis reaction (A) and the transesterification reaction (B) catalyzed by free MsAcT and immobilized MsAcT, respectively. The concentration of p-nitrophenyl acetate (pNPA) was increased from 10 μmol L−1 to 400 μmol L−1 in the hydrolysis reaction mixture; while the concentration of 2-phenethyl alcohol was increased from 10 mmol L−1 to 160 mmol L−1 in the transesterification reaction mixture. | |
Table 1 Kinetic constant for the hydrolysis reaction and the transesterification reaction catalyzed by free MsAcT and immobilized MsAcT, respectively
|
Reaction |
Vmax (μmol mg−1 min−1) |
Km (mmol L−1) |
kcat/Km (L mmol−1 min−1) |
Relative selectivitya |
Relative selectivity is defined as the catalytic efficiency (kcat/Km) of the transesterification reaction to that of the hydrolysis reaction. |
Free MsAcT |
Hydrolysis |
386.9 |
12.6 |
770.12 |
1.82 × 10−4 |
Transesterification |
0.42 |
74.3 |
0.14 |
Immobilized MsAcT |
Hydrolysis |
284.3 |
136.7 |
52.16 |
1.15 × 10−3 |
Transesterification |
0.16 |
64.4 |
0.06 |
As shown in Table 1, kcat/Km for the hydrolysis reaction of free MsAcT and immobilized MsAcT were 770.12 L mmol−1 min−1 and 52.16 L mmol−1 min−1, respectively. kcat/Km for the transesterification reaction of free MsAcT and immobilized MsAcT were 0.14 L mmol−1 min−1 and 0.06 L mmol−1 min−1, respectively. The relative selectivity for the transesterification reaction over the hydrolysis reaction of free MsAcT was 1.82 × 10−4, while the relative selectivity for the transesterification reaction over the hydrolysis reaction of the immobilized MsAcT was 1.15 × 10−3. Relative selectivity for the transesterification reaction over the hydrolysis reaction of the immobilized MsAcT increased by 6.33-fold. The enhanced selectivity of the immobilized MsAcT might be attributed to the fact that the immobilization process changed the microenvironment surrounding MsAcT. Hoffmann et al. also reported that selectivity of the immobilized β-glucosidase for synthesis activity over the hydrolysis reaction was directly improved through the tailored microenvironment.31
3.4 Optimization synthesis process of 2-phenethyl acetate using the immobilized MsAcT
As shown in Fig. 4, the maximum conversion rate of 2-phenethyl alcohol using three acyl donors, vinyl acetate, ethyl acetate, and acetic anhydride, was 91.24 ± 1.22%, 83.87 ± 0.94%, and 79.54 ± 0.81%, respectively. The conversion rates were in line with the reactivity of acyl donor, respectively.42,43 To obtain the maximum conversion rate, the molar ratio of acyl donor to 2-phenethyl alcohol varied with the types of acyl donors. When ethyl acetate and acetic anhydride were used as acyl donors, a molar ratio of 10
:
1 (acyl donor to 2-phenethyl alcohol) was required. As for vinyl acetate, a molar ratio of 2
:
1 was required (Fig. 4). As the transesterification reaction catalyzed by MsAcT was carried out in water, the reversible hydrolysis reaction also proceeded at the same time.44 In the transesterification reaction system with vinyl acetate as the acyl donor, the side product was spontaneously rearranged into acetaldehyde, which would decrease the undesired reversible hydrolysis reaction.42,45
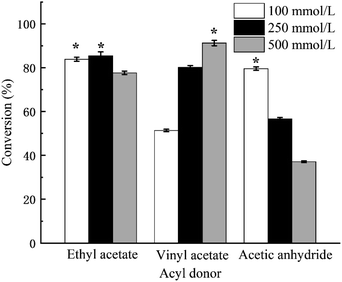 |
| Fig. 4 Effect of types of acyl donor and concentration of 2-phenylethyl alcohol on conversion rate. The asterisk (*) meant there was a significant difference and the p-value was less than 0.05. | |
As shown in Fig. 5, the conversion rate of 2-phenylethyl alcohol increased with the increase in water content in the range from 20% (w/w) to 80% (w/w) in all three transesterification reaction systems, regardless of the type of acyl donors. However, the maximum conversion rate displayed significant differences. The maximum conversion rate reached as high as 99.17 ± 0.6% in the reaction system using vinyl acetate as acyl donor with 80% (w/w) water content, while only a 50.63 ± 0.58% conversion rate was obtained in the reaction system using acetic anhydride as acyl donor with 80% (w/w) water content. Acetic anhydride was unstable and easy to decompose in water, which resulted in the low conversion rate of the acetic anhydride-reaction system.
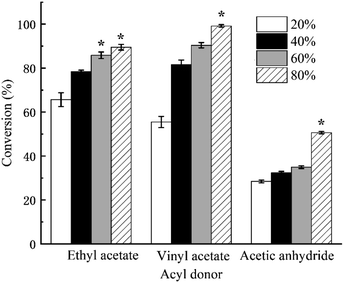 |
| Fig. 5 Effect of water content in the reaction system on the conversion rate. The asterisk (*) meant that there was a significant difference and the p-value was less than 0.05. | |
3.5 Time course of enzymatic synthesis of 2-phenylethanol acetate and operation stability of the immobilized MsAcT
Only thirty minutes was taken to reach the maximum conversion rate for the transesterification reaction systems using vinyl acetate and acetic anhydride as acyl donor. However, 4 h was taken for the reaction system using ethyl acetate as acyl donor (Fig. 6). Mestrom et al. also reported that the catalytic activity and catalytic efficiency of MsAcT using vinyl acetate as acyl donor was far higher than that of MsAcT using ethyl acetate as acyl donor.42 Various acyl donors had different chemical structures, which displayed different effects on enzyme acylation activity. The appropriate acyl donor closely resembled the “pocket” structure of enzyme, which resulted in a fully open active site cleft and an obviously positive effect on the activity.46,47
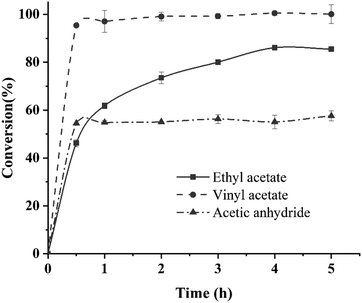 |
| Fig. 6 Time course of the conversion rate of 2-phenylethyl alcohol during the transesterification reaction catalyzed by the immobilized MsAcT using ethyl acetate, vinyl acetate, and acetic anhydride, respectively. | |
As shown in Fig. 7, the conversion rate of 2-phenylethyl alcohol catalyzed by the immobilized MsAcT did not decrease significantly in the first five batches and was kept stable around 95%. However, the conversion rate showed a slight decrease in the next five reaction batches, and only a 73.27 ± 1.68% conversion rate was obtained in the tenth batch. There were two reasons for the decrease in the conversion rate. Firstly, the by-product acetaldehyde in the reaction system using vinyl acetate as an acyl donor led to inactivation and denaturation of MsAcT. Secondly, part of the immobilized MsAcT powder was so small that it was difficult to be fully recovered from the reaction system in the process of recycling the immobilized MsAcT.
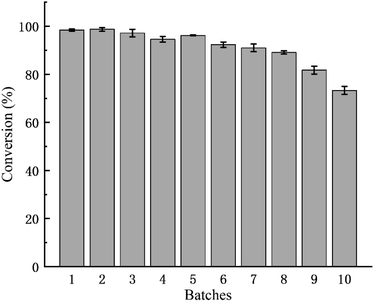 |
| Fig. 7 Operational stability of the immobilized MsAcT for the transesterification reaction using vinyl acetate as acyl donor. | |
4. Discussion
The toxicity of the solvent in a reaction system is one of the key criteria for whether the catalytic process can be identified as green catalysis.5 In previous work, various organic solvents and ionic liquids with low toxicity, such as n-hexane and 1,3-di(2-methylbutyl)imidazolium hexafluorophosphate, were selected for the enzymatic synthesis of 2-phenylethyl acetate.48,49 Compared with that of organic solvents and ionic liquids, MsAcT-catalyzed synthesis technology using water as the solvent simplifies the separation process of 2-phenylethyl acetate. However, water is strongly polar, while substrates in most organic synthesis reactions are weakly polar or hydrophobic. The enzyme-catalyzed synthesis process using water as the solvent will restrict the solubility of the substrate in the reaction system. In this research, the maximum concentration of 2-phenylethyl alcohol was 500 mmol L−1. Although the solubility of 2-phenylethyl alcohol in water is only 163.72 mmol L−1 at 20 °C, the solubility of 2-phenylethyl alcohol in the reaction system is significantly improved owing to the acyl donor (ethyl acetate, vinyl acetate, and acetic anhydride) with the high concentration used in this research. Under the current experimental condition, the reaction mixture is homogeneous for the whole reaction time and no interface appears. A clear two-phase with an interface will be formed from the MsAcT-catalyzed synthesis system of 2-phenylethyl acetate using water as a solvent when the concentration of 2-phenylethyl alcohol is over 500 mmol L−1. In fact, aqueous-organic two-phase systems have been widely used for the enzymatic-synthesis system.31,50,51 Some strategies, including screening organic solvents and adding co-solvents such as DMSO and acetone to the enzymatic-catalysis synthesis system with two aqueous-organic phases, can increase the concentration of insoluble substrates, and thereby increase the reaction rate.52–55
The second key parameter for MsAcT-catalyzed transesterification reaction using water as a solvent is the selectivity of the transesterification reaction over the hydrolysis reaction. The 3D structural characteristic of MsAcT itself directly affects and determines the strict preference for the synthesis reaction. MsAcT has a strongly hydrophobic tunnel leading to the catalytic site, which forms the mechanistic basis for favoring the transesterification reaction over the hydrolysis reaction in water.22 Similar structural characteristic and catalytic behavior is also reported from Candida parapsilosis lipase/acyltransferase (CpLIP2) and several of its homologs.56 The microenvironment in which MsAcT is surrounded is another important factor that regulates the selectivity of the transesterification reaction over the hydrolysis reaction. In this research, immobilization of MsAcT using tetramethoxysilane gel increased the selectivity of the transesterification reaction over the hydrolysis reaction by 6.33-fold. The hydrophobic tetramethoxysilane gel surrounded the MsAcT can exclude water molecules, while concentrate 2-phenylethyl alcohol.33 As shown in Table 1, the Michaelis–Menten constant (Km) of the immobilized MsAcT was significantly increased for hydrolysis reaction, and slightly decreased for transesterification reaction. Hoffmann et al. also reported that the tailored microenvironment of the immobilized β-glucosidase resulted in a 9.2-fold increase in the selectivity of synthesis activity over hydrolysis activity.31 However, the molecular mechanism by which the microenvironment improves the selectivity of synthesis activity over hydrolysis activity is still to be further investigated.
5. Conclusion
In summary, 2-phenylethyl acetate synthesis using MsAcT as a biocatalyst in water is a prospective green process technology. MsAcT immobilization using tetramethoxysilane gel is a simple and effective method to modify the microenvironment around MsAcT or its active site, which improves the catalytic selectivity of synthesis activity over hydrolysis activity.
Conflicts of interest
There are no conflicts to declare.
Acknowledgements
This research was supported by the National Natural Science Funds of China (No. 31870787 and No. 31370802) and by the Natural Science Funds of Fujian Province, China (No. 2021J01169 and No. 2017J01441).
References
- L. Poppe and B. G. Vértessy, ChemBioChem, 2018, 19, 1–5 CrossRef PubMed.
- U. T. Bornscheuer, Angew. Chem., Int. Ed., 2016, 55, 4372–4373 CrossRef CAS PubMed.
- S. Wu, R. Snajdrova, J. C. Moore, K. Baldenius and U. T. Bornscheuer, Angew. Chem., Int. Ed., 2021, 60, 88–119 CrossRef CAS PubMed.
- U. T. Bornscheuer, G. W. Huisman, R. J. Kazlauskas, S. Lutz, J. C. Moore and K. Robins, Nature, 2012, 485, 185–194 CrossRef CAS PubMed.
- J. B. Zimmerman, P. T. Anastas, H. C. Erythropel and W. Leitner, Science, 2020, 367, 397–400 CrossRef CAS PubMed.
- L. Maiuolo, V. Algieri, B. Russo, M. A. Tallarida, M. Nardi, M. L. Di Gioia, Z. Merchant, P. Merino, I. Delso and A. De Nino, Molecules, 2019, 24, 2909 CrossRef CAS PubMed.
- R. A. Sheldon and J. M. Woodley, Chem. Rev., 2018, 118, 801–838 CrossRef CAS PubMed.
- A. M. Klibanov, Nature, 2001, 409, 241–246 CrossRef CAS PubMed.
- W. Tsuzuki, A. Ue and A. Nagao, Biosci., Biotechnol., Biochem., 2003, 67, 1660–1666 CrossRef CAS PubMed.
- M. Mangiagalli, H. Carvalho, A. Natalello, V. Ferrario, M. L. Pennati, A. Barbiroli, M. Lotti, J. Pleiss and S. Brocca, Int. J. Biol. Macromol., 2020, 150, 930–940 CrossRef CAS PubMed.
- M. Z. Kamal, P. Yedavalli, M. V. Deshmukh and N. M. Rao, Protein Sci., 2013, 22, 904–915 CrossRef CAS PubMed.
- W. Tsuzuki, A. Ue and Y. Kitamura, Biosci., Biotechnol., Biochem., 2001, 65, 2078–2082 CrossRef CAS PubMed.
- N. Rueda, J. C. Dos Santos, C. Ortiz, R. Torres, O. Barbosa, R. C. Rodrigues, Á. Berenguer Murcia and R. Fernandez Lafuente, Chem. Rec., 2016, 16, 1436–1455 CrossRef CAS PubMed.
- B. G. Davis, Curr. Opin. Biotechnol., 2003, 14, 379–386 CrossRef CAS.
- J. N. Pedersen, Y. Zhou, Z. Guo and B. Pérez, Biotechnol. Bioeng., 2019, 116, 1795–1812 CrossRef CAS PubMed.
- S. Pramanik, G. V. Dhoke, K. E. Jaeger, U. Schwaneberg and M. D. Davari, ACS Sustainable Chem. Eng., 2019, 7, 11293–11302 CrossRef CAS.
- M. Parmentier, C. M. Gabriel, P. Guo, N. A. Isley, J. Zhou and F. Gallou, Curr. Opin. Green Sustain. Chem., 2017, 7, 13–17 CrossRef.
- M. J. Fink and P. O. Syrén, Curr. Opin. Chem. Biol., 2017, 37, 107–114 CrossRef CAS PubMed.
- P. Hendil Forssell, M. Martinelle and P. O. Syrén, Chem. Commun., 2015, 51, 17221–17224 RSC.
- D. B. Sindrila, N. Mathias, W. John and P. Günther, Catalysts, 2017, 7, 227 CrossRef.
- L. Lv, L. Dai, W. Du and D. Liu, Process Biochem., 2017, 58, 239–244 CrossRef CAS.
- I. Mathews, M. Soltis, M. Saldajeno, G. Ganshaw, R. Sala, W. Weyler, M. A. Cervin, G. White and R. Bott, Biochemistry, 2007, 46, 8969–8979 CrossRef CAS PubMed.
- A. A. Elamin, M. Stehr, R. Spallek, M. Rohd and M. Singh, Mol. Microbiol., 2011, 81, 1577–1592 CrossRef CAS PubMed.
- P. Kumar, M. W. Schelle, M. Jain, F. L. Lin, C. J. Petzold, M. D. Leavell, J. A. Leary, J. S. Cox and C. R. Bertozzi, Proc. Natl. Acad. Sci. U. S. A., 2007, 104, 11221–11226 CrossRef CAS PubMed.
- N. S. Amin, M. G. Boston, R. R. Bott, M. A. Cervin, E. M. Concar, M. E. Gustwiller, B. E. Jones, K. Liebeton, G. Miracle and H. Oh, Perhydrolyase, WO2005056782, 23.06, 2005.
- E. Jost, M. Kazemi, V. Mrkonji, F. Himo, C. K. Winkler and W. Kroutil, ACS Catal., 2020, 10, 10500–10507 CrossRef CAS.
- I. C. Perdomo, S. Gianolio, A. Pinto, D. Romano, M. L. Contente, F. Paradisi and F. Molinari, J. Agric. Food Chem., 2019, 67, 6517–6522 CrossRef CAS PubMed.
- M. L. Contente, A. Pinto, F. Molinari and F. Paradisi, Adv. Synth. Catal., 2018, 360, 4814–4819 CrossRef CAS.
- T. Hansson and P. Adlercreutz, Biotechnol. Bioeng., 2001, 75, 656–665 CrossRef CAS PubMed.
- M. Subileau, A. H. Jan, J. Drone, C. Rutyna, V. Perrier and E. Dubreucq, Catal. Sci. Technol., 2017, 7, 2566–2578 RSC.
- C. Hoffmann, C. Grey, M. Pinelo, J. M. Woodley, A. E. Daugaard and P. Adlercreutz, ChemPlusChem, 2020, 85, 137–141 CrossRef CAS.
- M. Bilal, J. Cui and H. M. N. Iqbal, Int. J. Biol. Macromol., 2019, 130, 186–196 CrossRef CAS PubMed.
- Y. F. Zhang and H. Hess, Chin. J. Chem. Eng., 2020, 28, 2028–2036 CrossRef CAS.
- H. Müller, A. K. Becker, G. J. Palm, L. Berndt, C. P. S. Badenhorst, S. P. Godehard, L. Reisky, M. Lammers and U. T. Bornscheuer, Angew. Chem., Int. Ed., 2020, 59, 11607–11612 CrossRef PubMed.
- J. Müller, M. A. Sowa, B. Fredrich, H. Brundiek and U. T. Bornscheuer, ChemBioChem, 2015, 16, 1791–1796 CrossRef PubMed.
- Z. Y. Shu, H. L. Wu, H. Lin, T. Li, Y. R. Liu, F. Ye, X. D. Mu, X. Li, X. Z. Jiang and J. Z. Huang, Biochem. Eng. J., 2016, 115, 56–63 CrossRef CAS.
- W. J. Jia, H. Li, Q. Wang, H. Lin, X. Li, J. Z. Huang, L. T. Xu, W. Q. Dong and Z. Y. Shu, J. Biotechnol., 2021, 328, 106–114 CrossRef CAS PubMed.
- M. M. Braford, Anal. Biochem., 1976, 72, 248–254 CrossRef.
- M. T. Reetz, A. Zonta and J. Simpelkamp, Biotechnol. Bioeng., 1996, 49, 527–534 CrossRef CAS.
- M. T. Reetz, P. Tielmann, W. Wiesenhoefer, W. Koenen and A. Zonta, Adv. Synth. Catal., 2003, 345, 717–728 CrossRef CAS.
- M. Kordel, B. Hofmann, D. Schomburg and R. D. Schmid, J. Bacteriol., 1991, 173, 4836–4841 CrossRef CAS PubMed.
- L. Mestrom, J. G. R. Claessen and U. Hanefeld, ChemCatChem, 2019, 11, 20042010 CrossRef.
- U. Hanefeld, Org. Biomol. Chem., 2003, 1, 2405–2415 RSC.
- M. Lundh, O. Nordin, E. Hedenström and H. E. Högberg, Tetrahedron: Asymmetry, 1995, 6, 2237–2244 CrossRef CAS.
- N. de Leeuw, G. Torrelo, C. Bisterfeld, V. Resch, L. Mestrom, E. Straulino, L. van derWeel and U. Hanefeld, Adv. Synth. Catal., 2018, 360, 242–249 CrossRef CAS.
- H. Ismail, R. M. Lau, F. V. Rantwijk and R. Sheldon, Adv. Synth. Catal., 2008, 350, 1511–1516 CrossRef CAS.
- J. H. Sun, R. J. Dai, W. W. Meng and Y. L. Deng, Catal. Commun., 2010, 11, 987–991 CrossRef CAS.
- H. Kim and C. Park, Enzyme Microb. Technol., 2017, 100, 37–44 CrossRef CAS PubMed.
- H. Shan, Y. Lu, Z. Li, M. Li, Y. Cai, X. Sun and Y. Zhang, Chin. J. Catal., 2010, 31, 289–294 CrossRef CAS.
- X. L. Tang, G. Y. Ye, X. Y. Wan, H. W. Li and Y. G. Zheng, Biochem. Eng. J., 2020, 161, 107708 CrossRef CAS.
- A. Negoi, V. I. Parvulescu and M. Tudorache, Catal. Today, 2018, 306, 199–206 CrossRef CAS.
- C. J. C. Rodrigues, M. Ferrer and C. C. C. R. de Carvalho, Catalysts, 2021, 11, 307 CrossRef CAS.
- E. Castillo, L. Casas-Godoy and G. Sandoval, Biocatalysis, 2015, 1, 178–188 Search PubMed.
- D. Ahumada, F. Arenas, F. Martínez-Gó, C. Guerrero, A. Illanes and C. Vera, Front. Bioeng. Biotechnol., 2020, 8, 859 CrossRef PubMed.
- I. P. Rosinha Grundtvig, S. Heintz, U. Krühne, K. V. Gernaey, P. Adlercreutz, J. D. Hayler, A. S. Wells and J. M. Woodley, Biotechnol. Adv., 2018, 36, 1801–1814 CrossRef CAS PubMed.
- A. H. Jan, M. Subileau, C. Deyrieux, V. Perrier and É. Dubreucq, Biochim. Biophys. Acta, 2016, 1864, 187–194 CrossRef CAS PubMed.
Footnote |
† Equal contributors. |
|
This journal is © The Royal Society of Chemistry 2022 |
Click here to see how this site uses Cookies. View our privacy policy here.