DOI:
10.1039/D1RA08232A
(Paper)
RSC Adv., 2022,
12, 2972-2979
A new highly selective “off–on” typical chemosensor of Al3+, 1-((Z)-((E)-(3,5-dichloro-2-hydroxybenzylidene)hydrazono)methyl) naphthalene-2-ol, an experimental and in silico study†
Received
10th November 2021
, Accepted 14th January 2022
First published on 21st January 2022
Introduction
Aluminum is the most abundant metal element in the earth's crust1,2 and is widely utilized to support human daily needs such as for kitchen ware, electrical apparatus, in the pharmaceutical industry, and also food for packaging materials.3,4 As a consequence, humans can be exposed easily to Al3+ ions for long periods, resulting in slow accumulation in various human organs through food, drugs, and water.3,5–13 Excessive intake of Al3+ ions in the human body may lead to severe diseases such as Alzheimer's,14,15 Parkinson,3,16 osteoporosis, osteomalacia,17 breast cancer,16,17 amyotrophic lateral sclerosis,14 neurofibrillary, enzymatic, and neurotransmitter changes in the central nervous system.18 Thus, early detection of the ions with a proper analysis method becomes urgently necessary, especially for trace analysis of the ions. Such expensive and time-consuming methods for aluminum detection are well established, like graphite furnace atomic absorption spectrometry and inductively coupled plasma atomic emission spectrometry. Comparatively, optical detection such as the fluorescence method shows unique potential for high sensitivity.19 At present, fluorescent chemosensors have been widely used to detect different metal ions because of their high sensitivity, selectivity, and fast response time,20,21 including to detect a trace of Al3+ ions. Therefore, the development of an analytical method of Al3+ ions based on fluorescence detection is still interesting, in particular, to solve the issues of weak coordination ability of Al3+ ions and its lack of spectroscopic characteristics.16,22–25
One of the most important and widely explored organic species for its potential applications as a chemosensor is Schiff bases with nitrogen moiety as the chelating centre of metal ions.13,24,26 Many fluorescent chemosensors of metal ions based on Schiff-base have been designed and examined owing to the simplicity, sensitivity, low-cost, synthetic ease, and strong chelating ability.23,27,28
Since the last decade, sensor molecule based on excited-state intramolecular proton transfer (ESIPT) mechanism draws considerable attention because exclusive photophysical properties like extraordinarily large Stokes shifted fluorescence emission without self-reabsorption, and environmental sensitivity.29 A molecule will experience favourably an ESIPT mechanism if it contains both an acidic (hydrogen donor group) and a basic site (hydrogen acceptor group) in proximity leading to keto–enol tautomerization at excited-state.30 Since the ESIPT process usually occurs on an ultrafast time scale (<100 fs), thus steady-state and femtosecond time-resolved spectroscopy are appropriately utilized to explore the mechanism.31 However, a DFT/TD-DFT calculation is commonly recommended to study the mechanism instead. In addition, a pre-condition of the ESIPT process such as intramolecular hydrogen bonding and emission changes upon ion chelation could support the theoretical prediction of the mechanism firmly.31–33 Perhaps, upon combination between an extended Schiff base imine behaving as a hydrogen acceptor and more hydrogen donors, it will not only direct a sensor to an ESIPT process but also improve the spectral properties of the chelated Al3+.
In this article, a new chemosensor with typical extended Schiff base moiety was successfully synthesized. The compound exhibited high selectivity and stability of coordination against Al3+ ion. A theoretical study was also performed to investigate the chelating mechanism of the sensor with the ion.
Experimental
Materials and method
The pro analysis grade reagents and solvents, purchased from Sigma Aldrich and Merck, were used. All glassware apparatus was oven-dried before use. 1H- and 13C-NMR spectra were obtained from Agilent 500 MHz and 126 MHz spectrometers, respectively in CDCl3. The NMR signals were referenced to the residual peak of the (major) solvent. The deuterated solvents were stored over activated 3 Å molecular sieves (8–12 mesh) under dry N2. UV/Visible absorption spectra and their molar absorptivity were measured on a Shimadzu 8400 UV/Vis spectrometer with matched 1.0 cm quartz cells. The spectra were recorded over 0.1 nm interval data. Emission spectra were recorded on an automated Fluorescence Agilent G9800A Cary Eclipse spectrometer in a 1.0 cm quartz fluorescence cuvettes at 25 °C. The slit of excitation and emission was set to 2.5 nm and 20 nm, respectively while the scanning rate was 600 nm min−1. Each sample was measured at least three times and the spectra were averaged. Infrared spectra were obtained on PerkinElmer FTIR with a potassium bromide (KBr) pellet. Relative masses were recorded on a Mass Spectrometer (Waters High Resolution-Time of Flight-MS Lockspray/HR-TOF-MS) in positive ion mode. The melting points were determined on an uncorrected MP55 electrothermal melting point apparatus in open-end capillary tubes. DFT and TD-DFT calculations32 were performed on a PC with Processor Intel®Xeon (R) CPU E5-2650 v2@2.60 GHz × 32, RAM 16 GB, Hard disk SSD PCIe, and VGA Zotac GeForce GTX 1080TI AMP EXT CORE. The calculation was performed using the Gaussian 09 program34 with B3LYP hybrid method35 and a basis set of 6-311+G(d,p). To achieve the actual condition of experiments, the solvent effect of DMSO was included in the calculations employing Integral Equation Formalism Variant Polarizable Continuum Mode (IEFPCM).36 Calculation covered such parameters as, structural geometry and spectrum of infra-red, for the mechanism of interaction study,36 and gap energy of HOMO–LUMO to study the stability of the chelate compound.35
Synthesis of 1-(hydrazonomethyl)naphthalene-2-ol (3)
The precursor of 3 was synthesized based on the method reported by Sousa et al. (2003).37 Hydrazine monohydrate 98% (0.29 g, 5.69 mmol) and 2-hydroxy-1-napthaldehyde (0.20 g, 1.16 mmol) were dissolved in methanol. The mixture was then stirred for 1.5 hours at ambient temperature. The reaction course was monitored by TLC with an eluent mixture of n-hexane: ethyl acetate (8:2, v/v). A yellowish-red solid was obtained upon solvent evaporation of the reaction mixture with a chemical yield of 92%. The product was directly used for the synthesis of 1 without any further purification. IR spectra (KBr): ν/cm−1; 3390 (weak, O–H), 3250 (weak, NH2), 3055 (weak, C–H aromatic), 1622 (strong, C
N), and 1580 (strong, C
C aryl) (Fig. SI1†). ToF-HRMS (ES+) m/z: [M−H]+ calculated for C11H11N2O 187.0866; found 187.0877 (Fig. SI2†).
Synthesis of 1-((Z)-((E)-(3,5-dichloro-2-hydroxybenzilydine)hydrazono) methyl)naphthalene-2-ol (1)
The target compound 1 was designed according to Scheme 1 by referring to analogue procedure reported by Gholizadeh Dogaheh et al. (2017).38 1-(hydrazonomethyl)naphthalene-2-ol 3 (0.11 g, 0.62 mmol) was dissolved in a two necks round bottom flask with 30 mL of methanol. 3,5-dichlorobenzaldehyde (0.13 g, 0.68 mmol) was then added stepwise to the reaction flask and stirred. The reaction mixture was further refluxed for 2 hours while employing TLC of silica gel GF-254 and eluent mixture of n-hexane: ethyl acetate (8:2, v/v) to monitor the product formation intermittently. A precipitated product was filtered out and washed with methanol to produce a pure yellow solid with chemical yield of 68%. M.P./°C: decomposed. UV/Vis spectra in DMSO (5 × 10−5 mol dm−3): λmax/nm (ε/mol−1 dm−3 cm−1): 330 (9420), 394 (13
760) and 494 (4840) (Fig. 1). Emission spectra in DMSO (10−5 mol dm−3): λex/nm; 360, λem/nm; 593 (Fig. 1). IR spectra (KBr): ν/cm−1; 3074 (medium, C–H sp2), 1612 (strong, C
N) and 1467 (medium, C
C aryl) (Fig. SI3†). 1H-NMR (500 MHz, CDCl3): δH/ppm; 12.73 (s, 1H); 12.24 (s, 1H); 9.62 (s, 1H); 8.66 (s, 1H) 8.09 (d, J = 8.4 Hz, 1H), 7.90 (d, J = 8.6 Hz, 1H), 7.81 (d, J = 8.0 Hz, 1H), 7.63–7.58 (t, 1H), 7.47 (s, 1H), 7.44–7.40 (t, 1H), 7.27 (s, 1H), 7.23 (d, J = 9.0 Hz, 1H) (Fig. SI4†). 13C-NMR (125 MHz, CDCl3): δC/ppm; 107.87; 119.12; 119.24; 119.94; 122.96; 124.27; 124.43; 128.36; 128.55; 129.50; 129.93; 132.75; 132.87; 136.05; 154.22; 161.63; 161.77 and 162.56 (Fig. SI5†). ToF-HRMS (ES+) m/z: [M−H]+ calculated for C18H13Cl2N2O2 359.0349; found 359.0368 (Fig. SI6†).
 |
| Scheme 1 Outline synthesis route of 1, 1-((Z)-((E)-(3,5-dichloro-2-hydroxybenzilydine)hydrazono) methyl)naphthalene-2-ol. (a) NH2NH2·H2O, MeOH, stirred at r.t. for 2 h, (b) 3,5-dichloro-2-hydroxybenzaldehyde, MeOH, refluxed for 2 h. | |
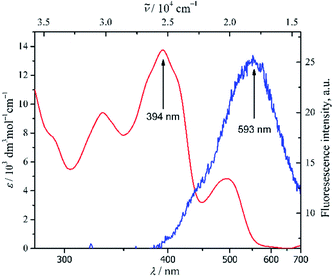 |
| Fig. 1 Spectra of UV/Vis of 1 in DMSO; λmax 394 nm ( ) (ctot 5 × 10−5 mol dm−3) and emission; λex 360 nm, λem 593 nm ( ) (ctot 10−5 mol dm−3). | |
General procedure of metal ion sensing of 1-((Z)-((E)-(3,5-dichloro-2-hydroxybenzilydine)hydrazono) methyl)naphthalene-2-ol (1)
The sensing method was adopted from the reported procedure.23,39 A stock solution of 1.0 × 10−4 mol dm−3 of 1 (C1) and metal cationic was prepared in DMSO independently. The representative metal cations were obtained from sulphate salt (K+, Li+, Mg2+, Fe3+, Ni2+, Cu2+, Al3+, Zn2+) and nitrate salt (Na+, Co2+, Cd2+, Hg2+, Pb2+, Ag+). The stock solution of C1 was mixed with various metal ions in a 5 mL volumetric flask at a mol ratio of 1:9 to 9:1 (C1: metal ion) by keeping constant total concentration at 10−5 mol dm−3. Binding stoichiometry was calculated based on Job's plot experiment, whilst the selectivity experiment was accomplished by mixing C1 and Al3+ at a mol ratio of 1
:
1 in the presence of each 1 mol of an interference ion. An association constant of the complex calculated with the Benesi–Hildebrand equation40,41 was obtained from the data of UV-Vis titration experiment of 1 (C1) (10 mol dm−3) in the presence of an increasing amount of Al3+ (2.4–26 mol dm−3). Whereas the LoD (3s/k) and LoQ (10s/k)42 of the 1 toward Al3+ were obtained from the data of fluorescence titration experiment of 1 (C1) (10−5 mol dm−3) in the presence of a gradual amount of Al3+ (1.3–16 mol dm−3). The pH effect was studied in DMSO
:
H2O (99
:
1, v/v) at 10−5 mol dm−3 using an aqueous solution of NaOH and HCl for pH adjustment. All fluorescence spectra were recorded at an excitation wavelength (λex) of 360 nm. The reversibility of the 1 against Al3+ was obtained by a fluorescence titration of the 1+Al3+ with EDTA.43,44 The emission quantum yield (ϕem) of 1 before and after the addition of Al3+ was calculated based on a relative value43 against a standard of quinine sulphate in 0.5 M of H2SO4 (ϕem = 0.546).45
Result and discussion
Synthesis of 1-((Z)-((E)-(3,5-dichloro-2-hydroxybenzilydine)hydrazono) methyl)naphthalene-2-ol (1)
Upon the formation of a double imine bond via addition–elimination reaction of hydrazine to the related aldehyde precursors, compound 1 was produced straightforwardly with an overall chemical yield of 63% (Scheme 1). Effortlessness synthesis of Schiff base compound46 was likewise applied to compound 1. Thus, no further purification was necessary to obtain a pure yellow solid of the product.
The infrared spectra of 1 (Fig. SI3†) showed clearly the appearance of imine moiety by a typical C
N vibration at 1612 cm−1 (strong, sharp). The appearance of the aromatic group of naphthalene and substituted benzaldehyde was assigned by the vibration of sp2 C–H at 3074 cm−1 (medium, sharp) and C
C aryl at 1467 cm−1 (medium, sharp). However, the sign of –OH moiety was barely apparent, which might be due to intramolecular hydrogen bonding with the nitrogen of imine. To clarify the structure, further analysis of 1H-NMR and 13C-NMR were recorded in CDCl3 (Fig. SI4 and SI5†). Six aromatic protons of naphthalene and two of substituted benzaldehyde had resonance at 7.23–8.10 ppm, whilst two protons of azomethine linkage of Schiff base were revealed at 8.66 ppm and 9.62 ppm. In fact, two more protons signal of –OH group appeared at 12.24 ppm and 12.73 ppm, shifted more downfield than common phenolic proton.47,48 The shifting of phenolic –OH chemical shift emphasized the probable intramolecular hydrogen bonding occurred, thus it was not distinctive on IR spectra. Furthermore, the intramolecular interaction confirmed the plausible geometry of compound 1. Supporting the 1H-NMR data, the 13C-NMR analysis revealed 18 distinctive carbon signals. Ten carbons had resonance at 107.87, 119.24, 119.94, 122.96, 128.36, 128.55, 129.50, 132.87, 136.05 and 162.56 ppm, were attributed to naphthalene carbon, whereas the six carbon signals of substituted benzaldehyde were revealed at 119.12, 124.27, 124.43, 129.93, 132.75 and 161.77 ppm. The other two carbons of azomethine were confirmed at 154.22 and 161.63 ppm. Finally, ToF-HRMS confirmed the molecular formula of compound 1 as C18H13Cl2N2O2 (ES+), which deviated merely 5 ppm from the theoretical mass (Fig. SI6†).
Photophysical properties of compound 1
The absorption spectra of 1 were obtained at 5 × 10−5 mol dm−3 in the most polar aprotic solvents of DMSO (Fig. 1). The spectra revealed n → π* characteristic transition bands at λmax 394 nm (13
760 ε/dm3 mol−1 cm−1) and 494 nm (4840 ε/dm3 mol−1 cm−1), which were plausibly attributed to azomethine and phenol moieties respectively. In addition, a π → π* transition band of conjugated aromatic ring and azomethine chromophore was also observed at 330 nm (9420 ε/dm3 mol−1 cm−1). Such a stronger absorption band of n → π* transition than commonly found was might be due to better solvation of polar aprotic DMSO through intermolecular hydrogen bonding, thus allowing to have better stabilization of the compound at the ground state than at the excited state.49 In fact, upon a less polar aprotic solvent like 1,4-dioxane, just diminished the corresponding n → π* transition band at 494 nm (Fig. SI7†). Further analysis of fluorescence spectra of 1 in DMSO at 10−5 mol dm3 (Fig. 1) asserted the strong interaction between compound 1 with the solvent which quenched significantly emission of the fluorophore of 1.
Sensing properties of compound 1 against various metal ions
The sensing properties of compound 1 against representative various metal ions including Na+, K+, Li+, Ca2+, Mg2+, Fe3+, Co2+, Ni2+, Cu2+, Cd2+, Hg2 +, Pb2+, Ag+, Al3+ and Zn2+ were evaluated in DMSO at ambient temperature. Typical metal-induced fluorescence enhancement was demonstrated by 1 in the presence of various metal ions under UV light at 365 nm (Fig. SI8†). In particular, considerable changes of fluorescence intensity of 1 were induced by Al3+ ion upon the molar ratio of 1:1 (Fig. 2). The Al3+ ion was able to induce the fluorescence of 1 up to one order of magnitude compared to the blank (ϕem of 1 = 0.020 ± 0.001; ϕem of 1 + Al3+ = 0.203 ± 0.009). In addition, hypsochromic shift emission maxima of 1 + Al3+ were also observed up to 152 nm compared to the maxima of 1. Such an enhanced electronic interaction of Al3+ with the core of 1, containing three coordination moieties, might increase planarity and stability between two aromatic parts of the chelated compound,50 thus a blue shift was perceived significantly.
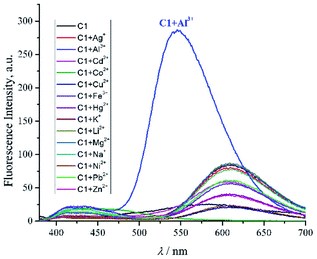 |
| Fig. 2 Emission spectra of compound 1 (C1) with the addition of various metal ion (1 : 1, molar ratio) in DMSO (ctotal 10−5 mol dm−3, λex 360 nm, λem 546 nm). | |
Such a fluorophore with Schiff base moieties nearby to any acidic proton like C1 potentially experienced excited-state intramolecular proton transfer (ESIPT) via intramolecular hydrogen bonding13,51 thus, the fluorescence was quenched. Conversely, upon coordination with Al3+ ion, the fluorescence enhancement might be attributed to the ESIPT inhibition process of C1, which favoured intramolecular charge transfer (ICT).52 The aforementioned plausible mechanism was in agreement with other similar Schiff base compounds reported elsewhere.53,54 Since the value of hydrogen bond-acceptor of DMSO is the strongest one (0.752) among other polar aprotic solvents,55,56 thereby could assist Al3+ ion to disrupt the ESIPT of C1 compared to other polar aprotic solvents (Fig. SI9†). Further competitive experiment of 1 + Al3+ chelated compound against other metal ions revealed that the presence of Al3+ ion among other metal ions exhibited immediate response of fluorescence enhancement peculiarly (Fig. 3). Thus, it emphasized the selectivity of 1 towards Al3+ and explicitly defined 1 as a typical off–on chemosensor.
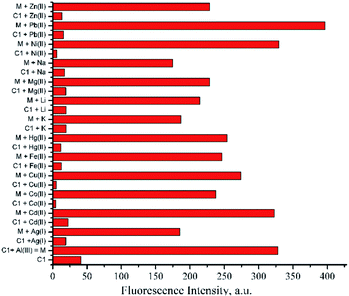 |
| Fig. 3 Selectivity of compound 1 (C1) toward Al3+ ion in the presence of other metal ions; Ag+, Cd2+, Co3+, Cu2+, Fe3+, Hg2+, K+, Li2+, Mg2+, Na+, Ni2+, Pb2+, Zn2+ in DMSO (1 : 1, molar ratio, ctotal 10−5 mol dm−3, λex 360 nm, λem 546 nm). | |
To determine binding stoichiometry between 1 and Al3+, Job's plot analyses were employed. Upon keeping the total concentration of the mixture at 10−5 mol dm−3 and varying the molar ratio of Al3+ from 0.1 to 0.9, the complex was found to be firmly established at a molar ratio of 1
:
1 (Fig. 4). In addition, analysis of ToF-HRMS (ES+) showed the exact complex composition of 1 + Al3+ as a 1:1 complex (calculated for C18H11AlCl2N2O2 385.0086; found 385.0436) (Fig. SI10†). The aforementioned data was further proved by the high association constant of the complex (8.73 × 105 M−1), obtained from the UV-Vis titration experiment and Benesi–Hildebrand equation (Fig. 5).40,41 The high association constant also confirmed a strong electronic interaction of Al3+ with the core of 1, hence forming a highly stable complex.
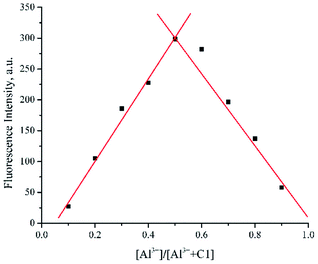 |
| Fig. 4 Job's plot of compound 1 (C1) with Al3+ in DMSO (ctotal 10−5 mol dm−3, λex 360 nm, λem 546 nm). | |
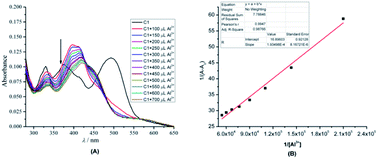 |
| Fig. 5 (A) UV/Vis titration profile of 1 (C1) (10 mol dm−3) in the presence of an increasing amount of Al3+ (2.4–26 mol dm−3) in DMSO (B) Benesi–Hildebrand plot of 1. | |
A fluorescence titration experiment of 1 in the presence of a gradual amount of Al3+ in DMSO was also conducted (Fig. 6). Based on the plotted data assisted by equations of 3s/k and 10s/k,42 the LoD and LoQ of 1 were found to be 0.04 and 0.14 μM respectively.
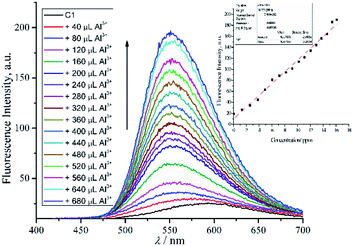 |
| Fig. 6 Fluorescence titration of 1 (C1) (10−5 mol dm−3; λex = 360 nm) against Al3+ ions at room temperature. Inset: plotted fluorescence intensity changes at 546 nm as a function of Al3+ concentration. | |
As compound 1 contained donor and acceptor of an acidic proton within proximity, further study of the interaction between compound 1 and Al3+ ion (1
:
1 molar ratio) at various pH was managed with aqueous NaOH or HCl in a mixture of DMSO-H2O (99
:
1, v/v) (Fig. 7). The graph revealed that Al3+ + 1 chelated compound exhibited changes of fluorescence intensity at various pH. In particular, the intensity weakened significantly below pH 7 and above pH 9. The faded fluorescence intensity at acidic conditions was presumably due to protonated imine Schiff base and phenolic moieties which inhibited 1 to coordinate Al3+ ion.57 On the other hand, upon extremely basic condition, Al3+ was plausibly converted to aluminum hydroxide and aluminate anion,58 allowed the sensor to be free as anionic species in the solution, and finally turned off the emission. Such a pH-dependent typical Schiff base chemosensor, involving an aqueous system, is commonly observed,43,59,60 especially for the biological application. However, to explore the extensive application of probe 1 under aqueous system domination, further investigation must be conducted. Especially, in terms of the changes of the sensing mechanism in the presence of water which eventually will influence the sensitivity and selectivity. The solvent and molecule symmetry was reported to affect significantly the ESIPT mechanism. In such a case, protic solvent (which undergoes hydrogen bonding) like water could suppress ESIPT by inhibiting keto (K*) emission, hence enol-emission remained at a shorter wavelength.61 On the other hand, a similar solvent could activate ESIPT and allow to switch on keto (K*) emission62 by inhibiting photoinduced electron transfer. In spite of that, sensor 1 was very potentially remained applicable at physiological conditions (pH 7–8). The pH study also confirmed the use of probe 1 as a practical sensor in a mixed aqueous medium DMSO-H2O (99
:
1, v/v).
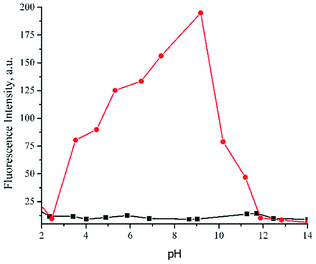 |
| Fig. 7 Effect of pH towards fluorescence intensity of 1 (( ) without and ( ) with Al3+ ion) in DMSO-H2O (99 : 1, v/v). | |
To observe the reversibility of sensor 1 toward Al3+, such a complexing agent like EDTA was used.43,44 Upon addition of 1 eq of EDTA to a mixture of 1 and Al3+, the fluorescence intensity of 1 + Al3+ was reduced substantially and approached the fluorescence profile of 1. Further addition of excess Al3+ (5 eq.) to the previous mixture, recovered the quenched fluorescence of 1 + Al3+ in proximity (Fig. 8). The results implied that the 1 + Al3+ chelated compound was reversibly dissociated by other complexing agents. Thus, it was potentially reused for certain times.
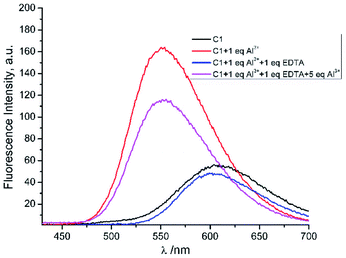 |
| Fig. 8 The fluorescence reversibility of 1 (C1) toward Al3+ (λex = 360 nm). | |
Computational study of chemosensor mechanism of 1 against Al3+ ion
To verify the interaction mechanism of 1 against Al3+ ion, DFT and TD-DFT calculations were performed. An in silico study (Table 1) revealed changes of bond order of hydrogen of donor toward acceptor upon excitation of 1-enol to S1. The bond order of O11–H36 (1-enol of S0) was found to stretch up to 0.96201 Å at S1 (1-enol of S1) approaching N14. Instead, decreasing a distance of N14–H36 down to 0.84294 Å, tailed by a bond formation sequentially was also observed. Based on optimized geometry of 1-enol (Fig. 9), the stretching vibration of O11–H36 at 3575.90 cm−1 was perceptible at S0 yet undetectable at S1. In addition, a new stretching vibration of N14–H36 at 3415.25 cm−1 appeared at S1. Accordingly, it validated the existence of proton transfer at an excited state. Overall, the optimized geometry didn't show any negative values of frequency vibration (Table SI1†), which indicated good accordance with calculation. The bond formation of N14–H36 at S1 should be favourable to occur because of intramolecular hydrogen bonding of O11–H36 and N14 was initially formed at S0 which eventually triggered an ESIPT at S1. Such intramolecular interaction between a hydrogen donor (–OH or –NH2) with a hydrogen acceptor (C
N– or C
O) in proximity less than 2 Å are generally arisen and allowed to undergo ESIPT at an excited state. Consequently, the fluorescence properties are quenched. However, upon strong coordination with an ion-assisted by strong acceptor hydrogen of aprotic solvent like DMSO, the ESIPT is plausibly inhibited, thus turning on back the fluorescence.51,53,54,63,64 Further study of HOMO–LUMO energy revealed that the gap energy of HOMO–LUMO of 1 decreased significantly up to 0.50 eV upon chelation with Al3+ (3.43 eV → 2.93 eV). Such low band gap energy of 1 + Al3+ presented good stability of the complex which was displayed in Fig. 10 as a model chelated compound. The calculation was in agreement with the high association constant of the complex obtained from the titration experiment. Overall, the computational study uncovered the interaction mechanism and reinforced the experimental results accordingly.
Table 1 The primary bond lengths (Å) of 1-enol at S0 and S1 states in DMSO, calculated by DFT and TD-DFT method at levels of B3LYP/6-31+G(d,p) and TD-B3LYP/6-31+G(d,p)
Bond |
Compound 1-enol/Å |
S0 |
S1 |
O22–H35 |
0.98585 |
0.97384 |
O11–H36 |
0.97451 |
1.93652 |
N14–H35 |
1.81533 |
1.97098 |
N14–H36 |
1.86873 |
1.02579 |
N14–O22 |
2.68221 |
2.77635 |
N14–O11 |
2.77314 |
2.81878 |
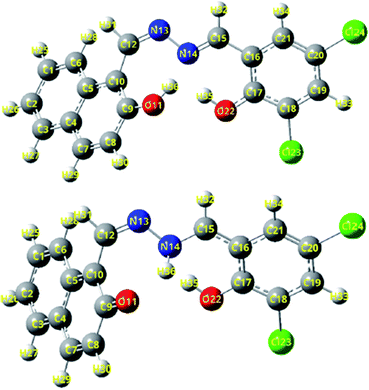 |
| Fig. 9 The optimized structures of 1-enol form at S0 and S1 state, employing a function of B3LYP/TD-B3LYP/6-311+G(d,p) level in DMSO. Top: 1-enol (S0) and below: 1-enol (S1). Red: oxygen, blue: nitrogen, white: hydrogen, grey: carbon, green: chlor. | |
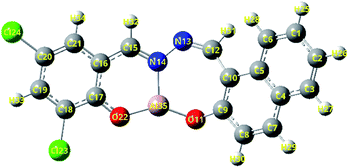 |
| Fig. 10 Optimized structure Chelated compound of 1-enol + Al3+. | |
Conclusions
A new simple Schiff base molecule based on 2-hydroxynaphthaldehyde derivative was successfully synthesized with an overall chemical yield of 63%. The compound demonstrated excellent interaction stability and sensing properties against Al3+ selectively. The probe showed good reversibility of sensing and was potentially applicable at physiological conditions (pH 7–8) in a mixture of DMSO
:
H2O (99
:
1, v/v). The LoD and LoQ of 1 against Al3+ in DMSO were 0.04 and 0.14 μM respectively. DFT/TD-DFT calculation confirmed the interaction mechanism of 1 via ESIPT inhibition. Thus, the compound was suggested as a potential chemosensor of Al3+.
Author contributions
JA was the main project leader and conceived the overall research idea. JA, DI, and AFA performed the experiments and data collection. AZ, IWH, MY, RM, and ATH were substantially involved in data analysis. DI and AFA drafted the manuscript and JA finalized it. All authors read and approved the final manuscript prior to submission.
Conflicts of interest
There are no conflicts to declare.
Acknowledgements
This work was supported by KEMENRISTEK DIKTI research grant (No. 1207/UN6.3.1/PT.00/2021) and ALG grant of Prof. Ace T. Hidayat. (No. 1959/UN6.3.1//PT.00/2021).
References
- D. Maity, S. Dey and P. Roy, New J. Chem., 2017, 41, 10677–10685 RSC.
- L. Bai, Y. Xu, G. Li, S. Tian, L. Li, F. Tao, A. Deng, S. Wang and L. Wang, Polymer, 2019, 11, 573 Search PubMed.
- B. Naskar, R. Modak, Y. Sikdar, D. K. Maiti, A. Bauzá, A. Frontera, A. Katarkar, K. Chaudhuri and S. Goswami, Sens. Actuators, B, 2017, 239, 1194–1204 CrossRef CAS.
- F. Liu, C. Fan, Y. Tu and S. Pu, RSC Adv., 2018, 8, 31113–31120 RSC.
- S. M. Hossain, K. Singh, A. Lakma, R. N. Pradhan and A. K. Singh, Sens. Actuators, B, 2017, 239, 1109–1117 CrossRef CAS.
- S. Santhi, S. Amala and S. M. Basheer, J. Chem. Sci., 2018, 130, 1–13 CrossRef CAS.
- S. Sambamoorthy and A. Subbiah, Athens J. Sci., 2018, 5, 141–166 CrossRef.
- R. Azadbakht, T. Almasi, H. Keypour and M. Rezaeivala, Inorg. Chem. Commun., 2013, 33, 63–67 CrossRef CAS.
- Y. Li, C. Liao, S. Huang, H. Xu, B. Zheng, J. Du and D. Xiao, RSC Adv., 2016, 6, 25420–25426 RSC.
- C. Liu, Z. Yang, L. Fan, X. Jin, J. An, X. Cheng and B. Wang, J. Lumin., 2015, 158, 172–175 CrossRef CAS.
- Y.-Y. Huang, F.-X. Wang, S.-Y. Mu, X. Sun, Q.-Z. Li, C.-Z. Xie and H.-B. Liu, Spectrochim. Acta, Part A, 2020, 15, 118754 CrossRef PubMed.
- J. Sun, M. Zhang and C. Sun, Can. J. Chem., 2019, 97, 387–391 CrossRef CAS.
- M. Tajbakhsh, G. B. Chalmardi, A. Bekhradnia, R. Hosseinzadeh, N. Hasani and M. A. Amiri, Spectrochim. Acta, Part A, 2018, 189, 22–31 CrossRef CAS PubMed.
- C. Chen, D. Liao, C. Wan and A. Wu, Analyst, 2013, 2, 2527–2530 RSC.
- V. K. Gupta, A. K. Singh and L. K. Kumawat, Sens. Actuators, B, 2014, 195, 98–108 CrossRef CAS.
- Y. W. Choi, G. J. Park, Y. J. Na, H. Y. Jo, S. A. Lee, G. R. You and C. Kim, Sens. Actuators, B, 2014, 194, 343–352 CrossRef CAS.
- R. Azadbakht and S. Rashidi, Spectrochim. Acta, Part A, 2014, 127, 329–334 CrossRef CAS PubMed.
- T. Jia, W. Cao, X. Zheng and L. Jin, Tetrahedron Lett., 2013, 54, 3471–3474 CrossRef CAS.
- R. Azadbakht, T. Almasi, H. Keypour and M. Rezaeivala, Inorg. Chem. Commun., 2013, 33, 63–67 CrossRef CAS.
- J. Xu, H. Li, L. Li, J. Wang, F. Wang and L. He, J. Braz. Chem. Soc., 2020, 31, 1778–1786 CAS.
- J. Al-Anshori, A. Rahim, A. Abror, I. Hidayat, T. Mayanti, M. Yusuf, J. Juliandri and A. Hidayat, J. Serb. Chem. Soc., 2021, 86, 971–982 CrossRef CAS.
- S. Banerjee, RSC Adv., 2016, 6, 101924–101936 RSC.
- C. Sun, X. Miao, L. Zhang, W. Li and Z. Chang, Inorg. Chim. Acta, 2018, 478, 112–117 CrossRef CAS.
- N. Roy, H. A. R. Pramanik, P. C. Paul and S. T. Singh, J. Fluoresc., 2014, 24, 1099–1106 CrossRef PubMed.
- J. C. Qin, Z. Y. Yang and P. Yang, Inorg. Chim. Acta, 2015, 432, 136–141 CrossRef CAS.
- J. C. Qin, Z. Y. Yang and P. Yang, Inorg. Chim. Acta, 2015, 432, 136–141 CrossRef CAS.
- H. Shekaari, A. Kazempour and M. Khoshalhanc, Phys. Chem. Chem. Phys., 2015, 17, 2179–2191 RSC.
- K. Zhang, Z. Y. Yang, B. D. Wang, S. B. Sun, Y. D. Li, T. R. Li, Z. C. Liu and J. M. An, Spectrochim. Acta, Part A, 2014, 124, 59–63 CrossRef CAS PubMed.
- A. Gupta and N. Kumar, RSC Adv., 2016, 6, 106413–106434 RSC.
- H. Tian, X. Qiao, Z.-L. Zhang, C.-Z. Xie, Q.-Z. Li and J.-Y. Xu, Spectrochim. Acta, Part A, 2019, 207, 31–38 CrossRef CAS PubMed.
- P. Zhou and K. Han, Acc. Chem. Res., 2018, 51, 1681–1690 CrossRef CAS PubMed.
- J.-S. Chen, P.-W. Zhou, L. Zhao and T.-S. Chu, RSC Adv., 2014, 4, 254–259 RSC.
- N. Zhao, Y. Li, Y. Jia and P. Li, J. Phys. Chem. C, 2018, 122, 26576–26583 CrossRef CAS.
- M. J. Frisch, G. Trucks, H. B. Schlegel, G. E. Scuseria, M. A. Robb, J. R. Cheeseman, G. Scalmani, V. Barone, G. A. Petersson, H. Nakatsuji, X. Li, M. Caricato, A. V Marenich, J. Bloino, B. G. Janesko, R. Gomperts, B. Mennucci, H. P. Hratchian, J. V Ortiz, A. F. Izmaylov, J. L. Sonnenberg, Williams, F. Ding, F. Lipparini, F. Egidi, J. Goings, B. Peng, A. Petrone, T. Henderson, D. Ranasinghe, V. G. Zakrzewski, J. Gao, N. Rega, G. Zheng, W. Liang, M. Hada, M. Ehara, K. Toyota, R. Fukuda, J. Hasegawa, M. Ishida, T. Nakajima, Y. Honda, O. Kitao, H. Nakai, T. Vreven, K. Throssell, J. A. Montgomery Jr, J. E. Peralta, F. Ogliaro, M. J. Bearpark, J. J. Heyd, E. . Brothers, K. N. Kudin, V. N. Staroverov, T. A. Keith, R. Kobayashi, J. Normand, K. Raghavachari, A. P. Rendell, J. C. Burant, S. S. Iyengar, J. Tomasi, M. Cossi, J. M. Millam, M. Klene, C. Adamo, R. Cammi, J. W. Ochterski, R. L. Martin, K. Morokuma, O. Farkas, J. B. Foresman and D. J. Fox, https://gaussian.com, 2016.
- I. Abdulazeez, C. Basheer and A. A. Al-Saadi, RSC Adv., 2018, 8, 39983–39991 RSC.
- S. Su and H. Fang, Spectrochim. Acta, Part A, 2020, 233, 118214 CrossRef CAS PubMed.
- C. Sousa, C. Freire and B. De Castro, Molecules, 2003, 12, 894–900 CrossRef.
- S. Gholizadeh Dogaheh, H. Khanmohammadi and E. C. Sañudo, Polyhedron, 2017, 133, 48–53 CrossRef CAS.
- M. Amirnasr, R. Sadeghi Erami and S. Meghdadi, Sens. Actuators, B, 2016, 233, 355–360 CrossRef CAS.
- K. C. Tayade, A. S. Kuwar, U. A. Fegade, H. Sharma, N. Singh, U. D. Patil and S. B. Attarde, J. Fluoresc., 2014, 24, 19–26 CrossRef CAS.
- Y. He, J. Yin and G. Wang, Chem. Heterocycl. Compd., 2018, 54, 146–152 CrossRef CAS.
- Q. Zhang, Q. Jiao, Q. Zhang and Z. Liu, Spectrochim. Acta, Part A, 2019, 211, 1–8 CrossRef CAS PubMed.
- J. Tian, X. Yan, H. Yang and F. Tian, RSC Adv., 2015, 5, 107012–107019 RSC.
- S. M. Hossain, K. Singh, A. Lakma, R. N. Pradhan and A. K. Singh, Sens. Actuators, B, 2017, 239, 1109–1117 CrossRef CAS.
- A. Brouwer, Pure Appl. Chem., 2011, 83, 2213–2228 CAS.
- A. Kundu, P. S. Hariharan, K. Prabakaran and S. P. Anthony, Spectrochim. Acta, Part A, 2015, 151, 426–431 CrossRef CAS PubMed.
- P. Charisiadis, V. G. Kontogianni, C. G. Tsiafoulis, A. G. Tzakos, M. Siskos and I. P. Gerothanassis, Molecules, 2014, 19, 13643–13682 CrossRef PubMed.
- P. E. Hansen and J. Spanget-Larsen, Molecules, 2017, 22, 552 CrossRef PubMed.
- A. Mohammadi and J. Jabbari, Can. J. Chem., 2016, 94, 631–636 CrossRef CAS.
- Z. Ning, S. Xuan, F. R. Fronczek, K. M. Smith and M. G. H. Vicente, J. Org. Chem., 2017, 82, 3880–3885 CrossRef PubMed.
- A. Grupta, RSC Adv., 2016, 6, 106413–106434 RSC.
- G. Dhaka, N. Kaur and J. Singh, J. Photochem. Photobiol., A, 2017, 335, 174–181 CrossRef CAS.
- H.-Y. Lin, P.-Y. Cheng, C.-F. Wan and A.-T. Wu, Analyst, 2012, 137, 4415–4417 RSC.
- L. Yang, W. Zhu, M. Fang, Q. Zhang and C. Li, Spectrochim. Acta, Part A, 2013, 109, 186–192 CrossRef CAS PubMed.
- M. J. Kamlet and R. W. Taft, J. Am. Chem. Soc., 1976, 98, 377–383 CrossRef CAS.
- M. J. Kamlet, J. L. M. Abboud, M. H. Abraham and R. W. Taft, J. Org. Chem., 1983, 48, 2877–2887 CrossRef CAS.
- T. S. Singh, P. C. Paul and H. A. R. Pramanik, Spectrochim. Acta, Part A, 2014, 121, 520–526 CrossRef CAS PubMed.
- Z. Salarvand, M. Amirnasr and S. Meghdadi, J. Lumin., 2019, 207, 78–84 CrossRef CAS.
- D. Maity, S. Dey and P. Roy, New J. Chem., 2017, 41, 10677–10685 RSC.
- C. Hazneci, K. Ertekin, B. Yenigul and E. Cetinkaya, Dyes Pigm., 2004, 62, 35–41 CrossRef CAS.
- S. Sharma and K. S. Ghosh, Spectrochim. Acta, Part A, 2021, 254, 119610 CrossRef CAS PubMed.
- L. McDonald, J. Wang, N. Alexander, H. Li, T. Liu and Y. Pang, J. Phys. Chem. B, 2016, 120, 766–772 CrossRef CAS PubMed.
- M. Tajbakhsh, Spectrochim. Acta, Part A, 2018, 189, 22–31 CrossRef CAS PubMed.
- A. C. Sedgwick, L. Wu, H. H. Han, S. D. Bull, X. P. He, T. D. James, J. L. Sessler, B. Z. Tang, H. Tian and J. Yoon, Chem. Soc. Rev., 2018, 47, 8842–8880 RSC.
Footnote |
† Electronic supplementary information (ESI) available. See DOI: 10.1039/d1ra08232a |
|
This journal is © The Royal Society of Chemistry 2022 |
Click here to see how this site uses Cookies. View our privacy policy here.