DOI:
10.1039/D1RA08875K
(Paper)
RSC Adv., 2022,
12, 2595-2602
Production of biologically active hydroxytyrosol rich extract via catalytic conversion of tyrosol
Received
6th December 2021
, Accepted 11th January 2022
First published on 19th January 2022
Abstract
An effective and economical process was established to produce hydroxytyrosol, a naturally occurring orthodiphenolic antioxidant molecule found in olive oil from its monophenolic precursor tyrosol. The approach proposed in the present work presents an environment-friendly method based on wet hydrogen peroxide catalytic oxidation with montmorillonite KSF as an inexpensive and environmentally benign solid acid at room temperature. The influence of the principal operating parameters including concentration of tyrosol, H2O2, and catalyst used were studied. The antioxidant activity was realized by the 2,2-diphenyl-1-picrylhydrazyl (DPPH) method. High antioxidant activity was detected according to the high hydroxytyrosol production (IC50 = 0.7 μg mL−1). The bactericidal and fungicidal properties of hydroxytyrosol rich extract were investigated using the NCCLS broth dilution and EN 1276 standard methods. Positive bactericidal and fungicidal effects of concentrations ranging between 1–0.5 g L−1 and 4–2 g L−1 were obtained.
1. Introduction
Olive oil is generally the main source of fat in the Mediterranean diet.1 Today, olive oil is synonymous with health, widely associated with many favorable effects, such as reduced incidence of different chronic diseases and prolonged longevity.2 Although present in minor amounts in the composition of the oil, phenolic compounds seem to be of great importance when it comes to health benefits, and interest in their biological and potential therapeutic effects is huge. These compounds are naturally recognized by the human body, and they do not present the adaptation problems encountered by other types of foreign polyphenol. There are also no problems due to the accumulation of polyphenols in the body, as they are soluble in water and are excreted by the renal and digestive systems. Tyrosol (Tyr) and hydroxytyrosol (Hyd), are the most abundant and representative phenolic alcohols in olive oil (90–95%).3,4 These compounds are also the most important for their contribution to flavor, stability, and nutritional value of the oil, and also demonstrate bioavailability in humans.5 In particular, hydoxytyrosol has been implicated for its health-stimulating effect mainly due to its antioxidative capacity. In various in vitro tests, it has been found that hydroxytyrosol offers better protection than oleuropein and vitamin E against various radicals such as linoleic acid micelles or in the removal of superoxide anion.6 Hydroxytyrosol has been shown to be a good antimicrobial agent against pathogenic bacteria in humans such as Haemophilus influenza, salmonellosis, and Vibrio parahaemolyticus, in clinically isolated strains, with greater efficacy than erythromycin and ampicillin.7 Hydroxytyrosol enforces the immunity system, it is the first group of molecules capable of multiple actions against the AIDS virus.8 It has been shown that Hyr is able to inhibit both initiation and promotion/progression phases of carcinogenesis by preventing the DNA damage induced by different genotoxic molecules and by inhibiting proliferation and inducing apoptosis in different tumor cell lines, respectively.8 Small dose of hydroxytyrosol can prevent the vasculopathy associated with diabetes mellitus and protect the cardiovascular system by suppressing LDL synthesis and against neurodegenerative processes.9 It is beneficial for eye health, especially for the regeneration of retinal pigment epithelium, macular degeneration, and glaucoma due to oxidative stress.10 Hyd has dermoprotective effects protection against UVB rays by reducing the pigmentation of the skin and stimulating the production of proteins that promote cell survival.11
Diverse synthesis procedures to produce HT have been developed,12 including by-electrochemical oxidation of p-tyrosol by means of electro-Fenton process using a carbon fiber cloth as cathode and Pt anode,13 biotransformation of tyrosol,14 enzymatic synthesis using tyrosinase as biocatalyst,15 conversion of oleuropein,16 mild photochemical synthesis via conversion of tyrosol,17 benchscale purification from olive mill wastewaters18 and bacterial synthesis.19 The production methods proposed are highly expensive and/or produce low yields or use severe conditions.
Given the reported values of hydroxytyrosol's biological properties, it is interesting that Hyd can be easily produced at competitive prices. In this investigation, we have developed, a mild and simple technique to produce high amounts of hydroxytyrosol from the chemoselective hydroxylation of tyrosol via the hydrogen peroxide catalytic oxidation process. This system is based on the generation of very reactive oxidizing free radicals with the assistance of montmorillonite KSF clay as a catalyst. The structure of the converted products was confirmed by using HPLC and LC-MS. The antiradical ability of tyr and its oxidation products was assessed by the 2,2-diphenyl-1-picrylhydrazyl (DPPH) assay. The bactericidal and fungicidal properties were also investigated using the NCCLS broth dilution and EN 1276 standard methods.
2. Materials and methods
2.1. Reagents
4-Hydroxyphenylethanol (Tyrosol 98%) was purchased from Alfa Aesar Company. Pure 3,4-dihydroxyphenylethanol (Hydroxytyrosol) used as standards for chromatographic calibration was provided by Seprox Biotech, S.L., (Madrid, Spain). The montmorillonite KSF choose as catalysts in this study was purchased from Fluka and used without further treatment. Hydrogen peroxide (30% solution in water) was obtained from Ce Pharma (Tunisia). Deionized water was used throughout the experiments and in the HPLC mobile phase. HPLC-grade solvents were used in all cases.
2.2. Tyrosol conversion
Tyr oxidation was carried out in a 100 mL Pyrex reactor equipped with a magnetic stirrer. The KSF catalyst (0.2–1 g L−1) was introduced into 50 mL of Tyr (10−3 to 10−2M) aqueous solution, under continuous stirring. The desired quantity of H2O2 (10−3 to 10−1M) was added indicating the beginning of the reaction. During the experiments, aliquots were taken at regular intervals for monitoring purposes. Following conversion of Tyr, the preparation was immediately filtered to completely remove catalyst particles.
2.3. Product characterization
2.3.1. HPLC analysis. Tyr and all other aromatics were identified and quantified by high performance liquid chromatography (Agilent 1260 Infinity quaternary LC, Germany). Phenolic compounds were separated on a C18 column (4.6 mm × 250 mm) and then analyzed using a Shimadzu SPD6AUV detector measuring wavelength at 278 nm. The mobile phase was a mixture of A and B solutions: (A) 70% acetonitrile in water and (B) 0.1% phosphoric acid in water with the percentage by volume of (B) solution varying linearly along the time as follows: from 10 to 25% for the first 25 min then from 25 to 80% up to 35 min and finally from 80 to 100% up to 50 min. The column temperature was maintained at 40 °C and the mobile flow rate was fixed at 0.6 mL min−1. Tyr, Hyd and hydroquinone (HQ) were identified and quantified using external standards.
2.3.2. LC-MS/MS analysis. LC-UV-MS/MS analyses were carried out as depicted by Kite and et al.20 on a Thermo Scientific System consisting of an Accela U-HPLC unit with a photodiode array detector and an LTQ Orbitrap XL mass spectrometer fitted with an electrospray source. Chromatography was performed with 5 μl sample injections on to a 150 × 3 mm i.d, 3 μm, Luna C18 (2) column (Phenomenex) using a 400 μL min−1 linear mobile phase with a methanol/water/acetonitrile + 1% formic acid changing 0
:
90
:
10 to 90
:
0
:
10 over 20 min followed by an isocratic phase for 5 min a column equilibrium in start condition was performed for 3 min before injection. Under the recommended manufacturers' conditions for the mobile phase parameters, the ESI source of the mass spectrometer was carried out in both positive and negative modes. The orbitrap mass analyzer was set to scan in a range of m/z 100–2000 at 30
000 resolutions, in either positive or negative polarity, while the linear ion-trap analysis was performed in both polarities. Analyses of MSn were required on the most abundant ions in both polarities using an ion isolated window of ±2 m/z and relative collision energy of 35%. Product ions, generated by MS2 in the ion trap, were scanned by the orbitrap at 7000 resolutions in order to attain accurate mass analyses. Negative mode ESI generally yielded more satisfactory ionization of the phenolic compounds. Acidification of the LC mobile phase allows the best separation, since the hydroxyl groups in the compounds are kept in their acidic form, thereby increasing their retention on the column and decreasing peak broadening.
2.4. Antioxidant activity assay
The DPPH (2,2-diphenyl-1-picrylhydrazyl) radical scavenging effect was evaluated according to the method described by Bouaziz et al.21,22 4 mL of methanolic solution of varying hydroxytyrosol sample concentration (25, 50, 100, and 150 μg mL−1) was added to 10 mL of DPPH methanol solution (1.5 × 10−4 M). After the two solutions had been gently mixed and left for 30 min at room temperature, the optical density was measured at 520 nm using a Shimadzu UV-160 A spectrophotometer. The test samples and positive control BHT were tested over a range of concentrations. The antioxidant activities of each test sample and BHT were expressed in terms of concentration required to inhibit 50% DPPH radical formation (IC50, μg mL−1) and calculated from the log-dose inhibition curve.
2.5. Antimicrobial activity
2.5.1. Microbial cultures. Antimicrobial activity was tested against a large panel of microorganisms including Staphylococcus aureus ATCC 9144, Pseudomonas aeruginosa ATCC 15442, Escherichia coli ATCC 10536, Bacillus subtilis ATCC 6633, Salmonella enterica CIP 80.39, Enterococcus hirae ATCC 10541, Candida albicans ATCC 10231 and Aspergillus niger ATCC 16404. The bacteria were cultivated in LB broth or LB Agar (Difco Laboratories, USA) at the appropriated temperature (30–37 °C) of the strains. Fungi were cultured on Malt Extract Broth (MEB) or Agar (MEA) (Difco Laboratories, USA) at 30 °C. Inocula were prepared by adjusting the turbidity of each culture of bacterium and yeast to reach an optical comparison to that of a 0.5 McFarland standard, corresponding to approximately 1–5 × 108 cfu per mL. The concentration of spore suspensions was determined using a hematocytometer (Thoma cell) and adjusted to 1–5 × 107 spore per mL.
2.5.2. Minimal inhibitory concentration (MIC), minimal bactericidal concentration (MBC) and minimal fungicidal concentration (MFC). The MICs, MBCs and MFCs of the synthesized compound against test organisms were determined as was recommended by the National Committee for Clinical Laboratory Standard.23 This test was performed in sterile 96-well microplates. The sample solutions were properly prepared and transferred to each microplate well in order to obtain a twofold serial dilution of the original sample. The inocula (10 μL) containing 104 cfu per mL of bacteria, yeasts or fungal spores were added to each well. Negative control wells contained bacteria or fungi only in their adequate medium. Positive control wells contained 10 μL−1 of chloramphenicol or gentamycin antibiotics for bacteria and fungi respectively. Thereafter, 30 μL of 0.02% resazurin and 12.5 μL of 20% Tween 80 were added. Plates were aerobically incubated at 30 °C for 16–20 h. After incubation, the wells were observed for a colour change from blue to pink. MIC was defined as the lowest concentration at which no growth was observed (blue coloured) after incubation. To determine MBC/MFC values, from each well with no visible growth, 100 μL of the mixture were removed and inoculated in LB or ME plates. After aerobic incubation at the appropriated temperature during 24 h, the CFU number of surviving organisms was determined.
3. Results and discussion
3.1. Chemical tyrosol conversion: identification of the intermediate derivatives
The catalytic oxidation of Tyr was performed in the presence of hydrogen peroxide with the assistance of montmorillonite KSF. From the first hour, the tyrosol begins to be converted into new phenolic intermediates until complete disappearance. During the reaction course, it was interestingly noted that the initial transparent color of the solution changed from colorless to yellow, then to brown and the intensity of the latter increased with time (Fig. 1). This reaction relies on the production of powerful antioxidant OH by the combination of the oxidant hydrogen peroxide with the presence of iron ions through the redox process known as the Fenton reaction.24 Fig. 2 shows the chromatogram of the evolution of the peaks during the reaction time, confirming the presence of three different peaks. Tyr peak (retention time 19.84 min) decreased with the accompanying increase of Hyd peak (retention time 12.5 min). To elucidate the structures of Tyr aromatic products obtained after the catalytic oxidation, a sample was analyzed by LC-MS.
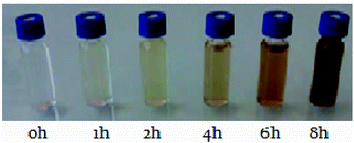 |
| Fig. 1 Intensity evolution of color aqueous solution during Tyr conversion over time: [Tyr] = 10−2 M; [H2O2] = 5.05 × 10−3 M, KSF = 0.2 g L−1. | |
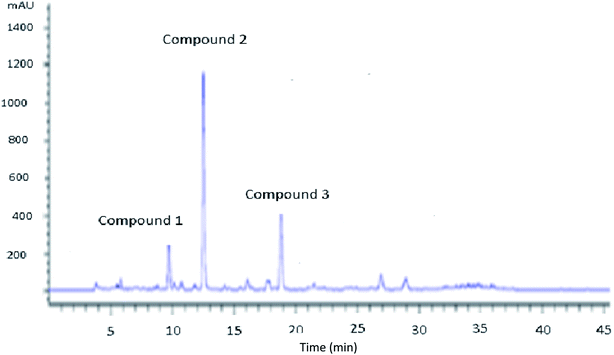 |
| Fig. 2 HPLC chromatogram of Tyr oxidation after 4 hours. Peaks: compound 1: HQ; compound 2: Hyd; compound 3: Tyr. Experiment conditions: [Tyr] = 10−2 M; [H2O2] = 5.05 × 10−3 M, KSF = 0.2 g L−1. | |
Compound 1 eluted at 9.67 min and exhibited [M–H]− at m/z 109.0597, predicting a molecular formula of C6H6O2 and in accordance with benzene-1,4-diol. The MS2 spectrum obtained by the fragmentation of the ion m/z 109.0597 yielded a major fragment at m/z 81 by the loss of carbonyl group (Fig. 3a).
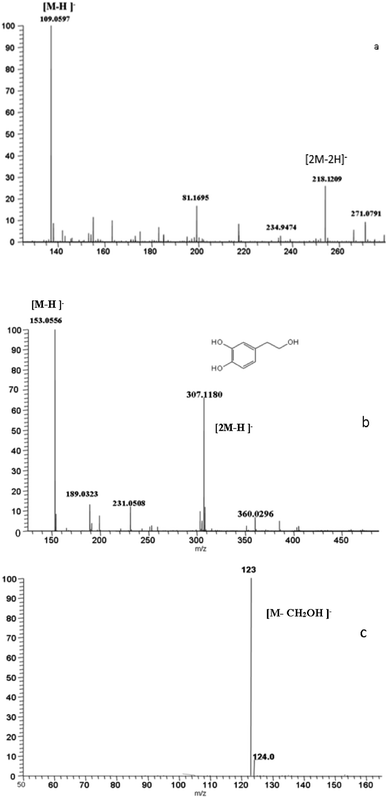 |
| Fig. 3 LC-MS/MS spectrum (MS1, MS2) highlighting the main fragments of Tyr and Tyr products. | |
Compound 2 was identified as 3,4-dihydroxyphenylethanol (hydroxytyrosol) by comparison of analytical data with an authentic standard. It eluted at 12.50 min with (λmax = 278 nm) and gave [M–H]− at m/z 153.0556, in accordance with a molecular formula of C8H10O3 (Fig. 3b). The MS2 of [M–H]− gave a fragment at m/z 123 by the loss of formaldehyde (Fig. 3c). Based on preliminary experiments and to a literature review, the most influential variables on tyrosol oxidation are specifically initial tyrosol concentration, H2O2 concentration, and catalyst concentration.
3.2. Oxidation mechanism of the tyrosol
The preceding results allow to schematize the oxidation mechanism of Tyr (Fig. 4). The Tyr molecules undergo a first electron transfer leading to the formation of phenoxy radicals for which the electronic charge reparation can be represented by three mesomeric forms [A], [B] and [C]. Moreover, the radical [B] undergoes a second electron transfer leading to the carbocation [B1], which gives the Hdr by hydrolysis. In the same way, the radical [C] was found to undergo a second electron transfer, leading to the carbocation [C1], yielding BQ [C2], which is reduced to HQ.
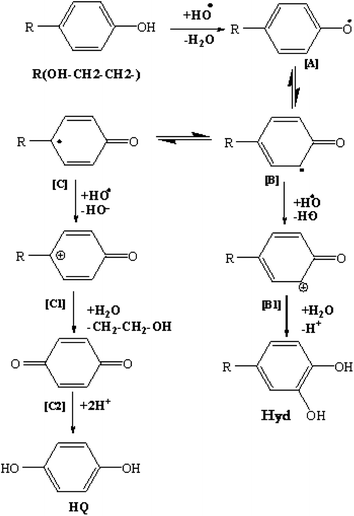 |
| Fig. 4 Reaction pathway of Tyr oxidation by KSF catalyst. | |
3.3. Influence of operating parameters on hydroxytyrosol production
In order to improve hydroxytyrosol production, several main operating factors can be adjusted, mainly the concentrations of tyrosol, hydrogen peroxide, and catalyst.
3.3.1. Effect of initial tyrosol concentration. Fig. 5 shows the Hyd production percentage versus time for three different Tyr concentrations with 5.05 × 10−3 M and 0.6 g L−1 the H2O2 and catalyst concentration, respectively. It can be observed that the rate of Hyd production is strongly dependent on the initial concentration of Tyr. Indeed, an increase in the concentration of 10−2 M to 10−1 M leads to an enhancement of the conversion rate of Hyd from 22% to 58%.
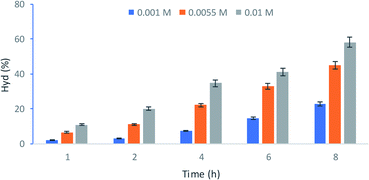 |
| Fig. 5 Effect of initial tyrosol concentration to Hyd production. Experiment conditions: [H2O2] = 5.05 × 10−3 M, KSF = 0.6 g L−1. | |
3.3.2. Effect of H2O2 concentration. The effect of H2O2 concentration on the catalytic oxidation was also studied. Fig. 6 shows the evolution of Hyd production rate versus time for a constant Tyr concentration (5.5 × 10−3 M) over KSF catalyst concentration (0.6 g L−1). It is clear that the concentration of H2O2 has an impact on the kinetics of the conversion. During the first 4 hours, an increase in the studied concentration from 10−3 M to 10−1 M allows having a significant improvement in the yield of 5% to 20% of Hyd production. This could be explained by the fact that more hydroxy radicals are formed as hydrogen peroxide increases. |
Fe 2+ + H2O2 → Fe3+ + HO− + ˙OH
| (3) |
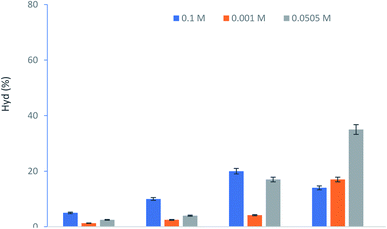 |
| Fig. 6 Effect of H2O2 concentration to Hyd production. Experiment conditions: [Tyr] = 5.5 × 10−3 M, KSF = 0.6 g L−1. | |
However, after this time, the excess of H2O2 has a negative impact and the percentage of Hyd formed was decreased. In fact, using a higher concentration of H2O2 acts as a quencher (eqn (4), (5), therefore, low content of hydroxy radical concentration.25
|
H2O2 + ˙OH → HO2˙ + H2O
| (4) |
|
HO2˙ + ˙OH → H2O + O2
| (5) |
Moreover, HO2 is less reactive than OH and this has a diminishing return on the reaction rate. It was reported26 that the higher production of OH radicals accelerates the oxidation kinetic of Tyr and other derivatives such as the hydroxytyrosol–iron complex. The performance of this conversion depends on the optimal dose of hydrogen peroxide. A low concentration of H2O2 cannot donate the maximum of hydroxy radical and the catalytic oxidation of Tyr is limited.27,28
3.3.3. Effect of KSF catalyst concentration. Given the important role played by the catalyst in all chemical reactions. This section established the impact of the catalyst amount on the oxidation reaction progress. For this, Tyr conversion tests were carried out over three different KSF catalyst concentrations (0.2, 0.6 and 1 g L−1) with a constant concentration 5.5 × 10−3 M of Tyr and 5.05 × 10−2 M of H2O2. The evolution of the production of Hyd is shown in Fig. 7. It seems that in the presence of a small amount of catalyst, although the conversion rate is slow at the beginning of the reaction, it continues to increase gradually until converting 45% of the initial Tyr after 8 hours. It is worth mentioning that the conversion of Tyr efficiently increases with a limited amount of catalyst up to 0.2 g L−1. Indeed, the concentration of Hyd decreases when the concentration of KSF increases (0.6 and 1 g L−1). This finding is in line with the literature which indicates that higher Fe2+ concentration (delivered from KSF) leads to the parasitic reaction and a loss of OH (eqn (6)) increases.29 |
Fe2+ + ˙OH → Fe3+ + OH−
| (6) |
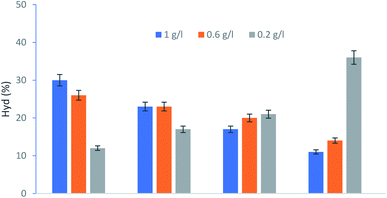 |
| Fig. 7 Effect of KSF concentration to Hyd production. Experiment conditions: [Tyr] = 5.5 × 10−3 M; [H2O2] = 5.05 × 10−3 M. | |
This result could be ascribed to the fact that the high concentration of the active Fe2+ species and the lowest rate of H2O2 parasitic decomposition increase the precipitation of the insoluble ferric hydroxides.30
The Hydroxytyrol concentrations obtained under different experimental conditions are summarized in Table 1. The optimum conditions, giving a higher concentration of Hyd, were as follows: Tyr concentration 10−1 M, the hydrogen peroxide concentration 7 × 10−2 M and 0.2 g L−1 of KSF.
Table 1 Concentration of Hydroxytysol production over different conditions
Experiment |
[Tyr] 10−3 M |
[H2O2] 10−2 M |
KSF (g) |
[Hyd] 10−4 M |
1 |
1 |
0.1 |
0.6 |
4 |
2 |
1 |
10 |
0.6 |
1 |
3 |
1 |
5.05 |
0.6 |
2.2 |
4 |
5.5 |
5.05 |
0.6 |
24.2 |
5 |
5.5 |
10 |
0.6 |
4.4 |
6 |
5.5 |
5.05 |
1 |
5.5 |
7 |
5.5 |
5.05 |
0.2 |
24.75 |
8 |
10 |
0.1 |
0.6 |
19.8 |
9 |
10 |
5.05 |
0.6 |
58 |
10 |
10 |
5.05 |
1 |
10 |
11 |
10 |
5.05 |
0.2 |
68 |
12 |
10 |
10 |
0.6 |
25 |
13 |
10 |
7 |
0.2 |
74 |
3.4. Antioxydant activity
The DPPH radical scavenging assay is a simple, rapid and easily reproducible method used for evaluating the efficiency of antioxidants as radical scavengers against stable DPPH free radicals. Scavenging activities of the evaluated antioxidants are shown in Fig. 8. Results are expressed as IC50, which is the effective concentration required to decrease the initial DPPH radical concentration by 50%.31
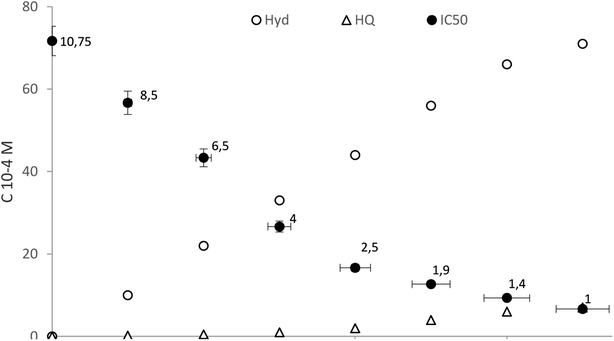 |
| Fig. 8 Free radical scavenging activity during catalytic oxidation of Tyr over the following conditions: 10−2 M [Tyr], 7 × 10−2 M [H2O2] and 0.2 g L−1 [KSF]. | |
It was clear (Fig. 8) that the antioxidant activity was related to the concentration of ortho hydroxylated phenolic compounds in the solution. However, in the beginning the aqueous methanolic extract exhibited lower radical-scavenging activity due to the low concentrations of Hyd. The IC50 value decreasing as the reaction progresses from 10.75 μg mL−1 at the start time to 0.7 μg mL−1 after 8 h. In fact, over time the solution is increasingly rich in hydroxytyrosol and therefore its oxidizing power increases. This finding coincides with earlier reports by Sun et al.,32 where Hyd had highest scavenging activity as the most polar antioxidant. As shown by Bouaziz et al., its activity is higher than that butylated hydroxytoluene BHT (IC50 = 0.91 μg mL−1), used as chemical food-preserving agents, taken as a ref. 33 This phenomenon is explained by the polar paradox and interface theories in which the polarity of antioxidants is proportional to their DPPH radical scavenging activity. It is known that the presence of two hydroxyl groups on the phenyl ring can increase radical-scavenging activity by allowing more hydrogen atoms of the phenolic hydroxyl groups which can be donated to stabilize the free radicals.34
3.5. Antimicrobial activities
The antimicrobial activities, as given by the minimum inhibitory concentration (MIC) and minimum bactericidal or fungicidal concentrations (MBC or MFC) values, of the tyrosol and its oxidative products are shown in Table 2. These results show that these products exhibited much higher antimicrobial activities compared to its precursor. This large increase in antimicrobial activity is strongly related to the high percentage of hydroxytyrosol. In fact, several research works35,36 have presented the high biological activity of this molecule, particularly its antioxidant and antimicrobial properties. In this context, when assayed against Salmonella species, and Staphylococcus aureus, hydroxytyrosol seemed much more active than oleuropein. MIC values were between 0.97 and 31.25 mg mL−1 for hydroxytyrosol and between 31.25 and 250 mg mL−1 for oleuropein which also considered more active than tyrosol.7 As reported by Papadopoulou et al., tyrosol is least likely to exhibit any antimicrobial activity, at least towards S. aureus, E. coli and Candida albicans.37 The low antimicrobial activity of tyrosol compared to hydroxytyrosol and oleuropein was probably attributed to its lack of a catecholic structure.38
Table 2 Minimal inhibitory concentrations (MICs) and minimal cidal concentrations (MCCs) of tyrosol and Hydroxytyrosol reach extracta
|
Organism |
MIC (mg mL−1) |
MBC/MFC (mg mL−1) |
MIC/MBC: 10 μg L−1 of chloramphenicol; MIC/MFC: 10 μg L−1 of gentamycin. |
Tyrosol |
Escherichia coli ATCC 10536 |
65–35 |
130–65 |
Bacillus subtilis ATCC 6633 |
65–35 |
130–65 |
Enterococcus hirae ATCC 10541 |
65–35 |
130–65 |
Salmonella enterica CIP 8039 |
65–35 |
130–65 |
Pseudomonas aeruginosa ATCC 15442 |
65–35 |
130–65 |
Staphylococcus aureus ATCC 9144 |
65–35 |
130–65 |
Aspergillus niger ATCC 16404 |
35–17 |
65–35 |
Candida albicans ATCC 10231 |
35–17 |
65–35 |
Hydroxytyrosol reach extract |
Escherichia coli ATCC 10536 |
2–1 |
2–1 |
Bacillus subtilis ATCC 6633 |
1–0.5 |
1–0.5 |
Enterococcus hirae ATCC 10541 |
4–2 |
4–2 |
Salmonella enterica CIP 8039 |
2–1 |
2–1 |
Pseudomonas aeruginosa ATCC 15442 |
2–1 |
2–1 |
Staphylococcus aureus ATCC 9144 |
4–2 |
4–2 |
Aspergillus niger ATCC 16404 |
2–1 |
4–2 |
Candida albicans ATCC 10231 |
2–1 |
2–1 |
Otherwise, the hydroxytyrosol riched extract revealed significant inhibition of growth of fungi as well as of Gram-positive and Gram-negative bacteria at low concentration (1–2 mg mL−1).
These statement are consistent with the work and opposes others that confirm that although several naturally occurring compounds have antimicrobial properties, few are active against Gram-negative bacteria.39 Hydroxytyrosol riched extract has also exhibit a high activity against Staphylococcus aureus, a microorganism extensively studied because of its capacity to produce enterotoxins and its exceptional resistance to natural phenolic compounds.
4. Conclusion
In vitro experimental study clearly reveals the efficient bactericidal and fungicidal action of Hydroxytyrosol providing effectiveness against a large panel of human pathogens and supports its use as an antimicrobial agent which could play a vital role in alleviating the spread of pathogenic microorganisms in food-, cosmetic-, agricultural and pharmaceutical-fields. These results reveal the biological importance of this eco-friendly synthesised product. Catalytic wet hydrogen peroxide oxidation of Tyr solutions in the presence of KSF heterogeneous catalysts could be considered as an alternative cheaper and nonpolluting route for Hyd production, a commercially unavailable compound with well-known biological properties that justify a potential commercial application.
Conflicts of interest
The authors declare that they have no conflict of interest.
Acknowledgements
This research was supported by the Tunisian Ministry of Higher Education and Scientific Research. We acknowledge the significant contribution of all the experts who participated in this study.
References
- A. Mazzocchi, L. Leone, C. Agostoni and I. Pali-Schöll, Nutrients, 2019, 3, 2941 CrossRef PubMed.
- A. Cañuelo, B. Gilbert-López, P. Pacheco-Liñán, E. Martínez-Lara, E. Siles and A. Miranda-Vizuete, Mech. Ageing Dev., 2012, 133, 563–574 CrossRef PubMed.
- R. Mateos, S. Martínez López, G. Baeza Arévalo, M. Amigo-Benavent, B. Sarriá and L. Bravo Clemente, Food Chem., 2016, 205, 248–256 CrossRef CAS PubMed.
- N. Kitsati, M. D. Mantzaris and D. Galaris, Redox Biol., 2016, 10, 233–242 CrossRef CAS PubMed.
- J. Anter, I. Tasset, S. Demyda-Peyrás, I. Ranchal, M. Moreno-Millán, M. Romero-Jimenez, J. Muntané, M. Dolores Luque de Castro, A. Muñoz-Serrano and A. Alonso-Moraga, Mutat. Res., Genet. Toxicol. Environ. Mutagen., 2014, 772, 25–33 CrossRef CAS PubMed.
- J. E. Hayes, P. Allen, N. Brunton, M. N. O'Grady and J. P. Kerry, Food Chem., 2011, 126, 948–955 CrossRef CAS.
- G. Bisignano, A. Tomaino, R. Lo Cascio, G. Crisafi, N. Uccella and A. Saija, J. Pharm. Pharmacol., 1999, 51, 971–974 CrossRef CAS PubMed.
- C. Vilaplana-Pérez, D. Auñón, L. A. García-Flores and A. Gil-Izquierdo, Front. Nutr., 2014, 1, 18 Search PubMed.
- C. D'Angelo, S. Franceschelli, J. L. Quiles and L. Speranza, Cells, 2020, 9, 1932 CrossRef PubMed.
- L. Zhu, Z. Liu, Z. Feng, J. Hao, W. Shen, X. Li, L. Sun, E. Sharman, Y. Wang, K. Wertz, P. Weber, X. Shi and J. Liu, J. Nutr. Biochem., 2010, 21, 1089–1098 CrossRef CAS PubMed.
- L. Martínez, G. Ros and G. Nieto, Medicines, 2018, 5, 13 CrossRef PubMed.
- R. Bernini, N. Merendinob, A. Romanic and F. Velotti, Curr. Med. Chem., 2013, 20, 655–670 CrossRef CAS PubMed.
- F. B. Abdallah, E. Hmani, M. Bouaziz, M. Jaziri and R. Abdelhedi, Sep. Purif. Technol., 2017, 188, 260–265 CrossRef.
- S. J. Brooks, E. M. Doyle and K. E. O'Connor, Enzyme Microb. Technol., 2006, 39, 191–196 CrossRef CAS.
- J. C. Espín, C. Soler-Rivas, E. Cantos, F. A. Tomás-Barberán and H. J. Wichers, J. Agric. Food Chem., 2001, 49, 1187–1193 CrossRef PubMed.
- R. Briante, F. La Cara, M. P. Tonziello, F. Febbraio and R. Nucci, J. Agric. Food Chem., 2001, 49, 3198–3203 CrossRef CAS PubMed.
- S. Azabou, W. Najjar, A. Ghorbel and S. Sayadi, J. Agric. Food Chem., 2007, 55, 4877–4882 CrossRef CAS PubMed.
- N. Allouche, S. Sayadi and M. Damak, J. Soc. Chim. Tunis., 2004, 6, 33–44 CAS.
- Z. Bouallagui and S. Sayadi, J. Agric. Food Chem., 2006, 54, 9906–9911 CrossRef CAS PubMed.
- G. C. Kite, C. A. Stoneham and N. C. Veitch, Phytochemistry, 2007, 68, 1407–1416 CrossRef CAS PubMed.
- M. Bouaziz, I. Feki, M. Ayadi, H. Jemai and S. Sayadi, Eur. J. Lipid Sci. Technol., 2010, 112, 894–905 CrossRef CAS.
- S. K. Trabelsi, O. Gargouri Dridi, B. Gargouri, R. Abdelhèdi and M. Bouaziz, Electrochim. Acta, 2015, 173, 370–376 CrossRef CAS.
- National Committee for Clinical Laboratory Standard, Method for Dilution Antimicrobial Susceptibility Tests for Bacteria That Grow Aerobically Approved Standard, M7-A6, Wayne, Pa, USA, 2003 Search PubMed.
- P. Salgado, V. Melin, D. Contreras, Y. Moreno and H. D. Mansilla, J. Chil. Chem. Soc., 2013, 58, 1842–1847 CrossRef.
- R. Ben Hmida, N. Frikha, S. Bouguerra, G. Kit and F. Medina, Int. J. Chem. Kinet., 2020, 52, 124–133 CrossRef CAS.
- I. Sirés, E. Brillas, M. A. Oturan, M. A. Rodrigo and M. Panizza, Environ. Sci. Pollut. Res., 2014, 21, 8336–8367 CrossRef PubMed.
- X. Wei, H. Wu and F. Sun, J. Colloid Interface Sci., 2017, 504, 611–619 CrossRef CAS PubMed.
- D. Gümüş and F. Akbal, Process Saf. Environ. Prot., 2016, 103, 252–258 CrossRef.
- L. Ma, M. Zhou, G. Ren, W. Yang and L. Liang, Electrochim. Acta, 2016, 200, 222–230 CrossRef CAS.
- N. Frikha, S. Bouguerra, S. Ammar, F. Medina, R. Abdelhedi and M. Bouaziz, React. Kinet., Mech. Catal., 2019, 128, 903–916 CrossRef CAS.
- Z. Wenjing, C. Haixia, W. Zhaoshuai, L. Gaoshuang and Z. Likang, J. Food Sci. Technol., 2013, 50, 1122–1129 CrossRef PubMed.
- Y. Sun, D. Zhou and F. Shahidi, Food Chem., 2018, 245, 1262–1268 CrossRef CAS PubMed.
- M. Bouaziz, A. Dhouib, S. Loukil, M. Boukhris and S. Sayadi, Afr. J. Biotechnol., 2010, 8(24), 7017–7027 Search PubMed.
- E. Bendary, R. R. Francis, H. M. G. Ali, M. I. Sarwat and S. El Hady, Ann. Agric. Sci., 2013, 58, 173–181 CrossRef.
- N. D. Utami, A. Nordin, H. Katas, R. B. H. Idrus and Mh Busra Fauzi, Biomolecules, 2020, 10, 1397 CrossRef CAS PubMed.
- M. S. Medina-Martínez, P. Truchado, I. Castro-Ibáñez and A. Allende, Biosci., Biotechnol., Biochem., 2016, 80, 801–810 CrossRef PubMed.
- C. Papadopoulou, K. Soulti and I. G. Roussis, Food Technol. Biotechnol., 2005, 43, 41–46 CAS.
- E. Tripoli, M. Giammanco, G. Tabacchi, D. Di Majo, S. Giammanco and M. La Guardia, Nutr. Res. Rev., 2005, 18, 98–112 CrossRef CAS PubMed.
- H. S. Tranter, C. C. Tassou and G. J. Nychas, J. Appl. Bacteriol., 1993, 74, 253–259 CrossRef CAS PubMed.
|
This journal is © The Royal Society of Chemistry 2022 |
Click here to see how this site uses Cookies. View our privacy policy here.