DOI:
10.1039/D1RA09062C
(Paper)
RSC Adv., 2022,
12, 10460-10466
Synthesis of benzoin under supramolecular catalysis involving cyclodextrins in water: application for the preparation of the antiepileptic drug phenytoin†
Received
15th December 2021
, Accepted 23rd March 2022
First published on 4th April 2022
Abstract
Among the cyclodextrins screened for the synthesis of 2-hydroxy-1,2-diphenylethanone (benzoin) in water, 2-hydroxypropyl-β-cyclodextrin (HP-β-CD) exhibited the highest yield in the benzoin condensation reactions, and HP-β-CD can be recycled several times with little loss of activity through the addition of fresh VB1. As an example of supramolecular catalysis, the methodology was applied to the “green” synthesis of the antiepileptic drug phenytoin through benzoin condensation, oxidation, and cyclization reactions in the presence of HP-β-CD, without the use of any harmful organic solvent. Moreover, the complexation behaviors of HP-β-CD with benzaldehyde and intermediates were studied by UV-vis and 2D-ROESY NMR spectroscopies to reveal the plausible mechanisms of the reactions, and HP-β-CD did not act as a simple phase transfer agent.
Introduction
Cyclodextrins (CDs) are cyclic oligosaccharides composed of six, seven, or eight glucopyranose units linked by α-1,4-glycosidic bonds (α, β, and γ-CD, respectively) (Scheme 1).1–3 The distinguishing feature of CDs is to form inclusion complexes with small guest molecules by noncovalent bonds in aqueous solution or in solid state.4 Due to their remarkable inclusion capabilities with small organic molecules, more recent interests focus on organic reactions catalyzed by CDs and their modified derivatives.5–8
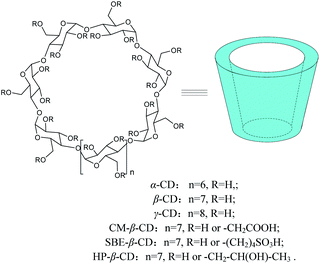 |
| Scheme 1 The chemical structures of CDs. | |
Benzoin, 2-hydroxy-1,2-diphenylethanone, is an important chemical intermediate, and has been widely used as a photo-sensitizer for photosensitive resins, gravure inks, and photocurable coatings as well as a catalyst for the production of polyesters.9–11 The old-fashioned method for synthesizing benzoin is catalyzed by cyanide, which consisted of toxic substances and caused environmental pollution. In recent years, vitamin B1 (VB1) was reported as a new catalyst for benzoin condensation reaction.12–19 However, the reaction system needs organic solvents (ethanol) and is carried out under reflux conditions. An interesting strategy is that the conjugates of VB1 with CDs, where VB1 is covalently linked to CDs, catalyzed the benzoin condensation reaction, which required DMSO as a solvent.20,21
Additionally, one of the most important applications of benzoin is the synthesis of the antiepileptic drug phenytoin, which suppresses systemic epilepsy and has a good effect on partial epilepsy.22,23 Most of the routes for the synthesis of phenytoin include the oxidation of benzoin to 1,2-diphenylethanedione, and the cyclization of 1,2-diphenylethanedione with urea, and the processes generally use ethanol and glacial acetic acid as solvents. Recently, novel strategies of the synthesis of phenytoin are developed, such as liquid phase heterogeneous synthesis and mechanochemistry.24–29
In general, the preparation procedures of benzoin and phenytoin require organic solvents, which exhibit a certain level of human and environmental toxicity. Therefore, green synthesis is an innovative method to approach the synthesis of benzoin and phenytoin by supramolecular catalysis involving CDs in water. In the present study, we reported the efficient and mild VB1-catalyzed benzoin condensation reaction, using CDs as cocatalysts in water. The effects of some key variables on the yield of benzoin were studied for optimizing the most suitable reaction conditions. In addition, the methodology was applied to the “green” synthesis of the antiepileptic drug phenytoin with no use of any harmful organic solvent, and the mechanism underlying the reactions was proposed through 2D-ROESY NMR investigations.
Experiment approaches
Materials
α-Cyclodextrin (α-CD), β-cyclodextrin (β-CD), γ-cyclodextrin (γ-CD), carboxymethyl-β-cyclodextrin (CM-β-CD, DS = 6.8, MW = 1528), sulfobutylether-β-cyclodextrin (SBE-β-CD, DS = 6.5, MW = 2018), and 2-hydroxypropyl-β-cyclodextrin (HP-β-CD, DS = 4.76, MW = 1410) were purchased from Shanghai Macklin Biochemical Co., Ltd, Shanghai, China. Benzaldehyde (≥98.5%) was obtained from Tianjin Kexin Chemical Industry Co., Ltd, China. Vitamin B1 was provided by Shanghai Aladdin Biochemical Technology Co., Ltd, Shanghai, China. All other reagents and solvents were of analytical grade and used without further purification unless indicated. Distilled water was used in all the experiments.
Synthesis of benzoin
Vitamin B1 (0.72 g, 2.39 mmol) and HP-β-CD (1.5 g, 1.06 mmol) were dissolved in distilled water (8 mL). Then cold 2N NaOH solution (2 mL) and benzaldehyde (2 mL, 19.6 mmol) were added to the above mixed solution and shook. The pH of the solution was adjusted to 9.0 with cold 2N NaOH solution. The reaction solution was placed at room temperature for 1 week, and a yellow white crystal was formed. The precipitate was filtrated under vacuum, washed several times with distilled water, and dried to give 1.85 g crystal benzoin in 89% yields. M.p. 135–137 °C.30 1H NMR (400 MHz, MeOD) δ 7.93–7.91, 7.51–7.47, 7.39–7.35, 7.30–7.20, 6.08, 4.87 (H2O), and 3.36 (MeOD).
Synthesis of 1,2-diphenylethanedione
FeCl3·6H2O (16.8 g, 62.2 mmol) were dissolved in distilled water (25 mL), and heated to reflux for 5 minutes. After the reaction solution was cooled to room temperature, HP-β-CD (2 g, 1.42 mmol), benzoin (3 g, 14.15 mmol) were added, and heated to reflux for 90 minutes. After slightly cooled, the reaction solution was poured into 60 mL H2O and stirred to cool until the crystals were completely precipitated. The precipitate was filtrated under vacuum, washed several times with distilled water, and dried to give 2.8 g 1,2-diphenylethanedione in 94% yields. M.p. 95–98 °C.27 1H NMR (400 MHz, MeOD) δ 7.97, 7.95, 7.76, 7.74, 7.72, 7.61, 7.59, 7.57, 7.35, 4.90 (H2O), and 3.33 (MeOD).
Synthesis of phenytoin
1,2-Diphenylethanedione (2.6 g, 12.38 mmol), urea (0.98 g, 16.33 mmol), 15% NaOH (8.2 mL), and HP-β-CD (1.84 g, 1.31 mmol) were dissolved in distilled water (14 mL) and heated to reflux for 60 minutes. Then, the solution was poured into 82 mL H2O with CH3COONa (0.33 g, 4.03 mmol). After filtration, the filtrate was adjusted to about pH 4–5 with 10% hydrochloric acid. The precipitate was filtrated under vacuum, washed several times with distilled water, and dried to give 2.5 g phenytoin in 80% yields. M.p. 296–299 °C.25,28 1H NMR (400 MHz, MeOD) δ 7.40, 7.39, 7.38, 7.36, 4.91 (H2O), 3.36 (MeOD), 1.96, 1.16.
Results and discussion
Green synthesis of benzoin catalyzed by CDs
In the synthesis of benzoin catalyzed by CDs (Scheme 2), the effects of some key variables on the yield of benzoin were investigated by single-factor experiments for optimizing the most suitable reaction conditions. These variables include the reaction time, reaction pH, amount of VB1, type and amounts of CDs, and the recycling efficiency of catalysts.
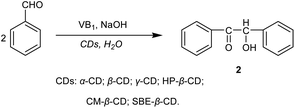 |
| Scheme 2 Synthesis of benzoin catalyzed by CDs. | |
Effect of the type of CDs on the yield of benzoin
In our work, we found that simply mixing benzaldehyde with VB1 in water did not result in an obvious reaction, due to the poor solubility of benzaldehyde as a raw material. For the successful VB1-catalyzed benzoin condensation reaction using CDs as cocatalysts in water, the selection of the suitable CDs on the reaction should be one of the key factors to accelerate the condensation of benzaldehyde, and to increase reaction efficiency. Therefore, the effect of the type of CDs on the yield of benzoin was studied for the benzoin condensation reaction, and the reaction results were shown in Fig. 1.
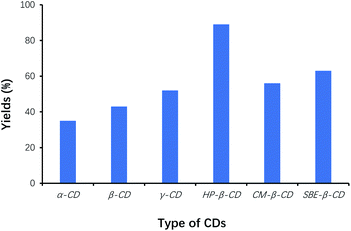 |
| Fig. 1 Effects of type of CDs on the yields of benzoin. The same concentration of different CDs was used with respect to the initial amount of benzaldehyde (catalyst loading 5%). | |
Among the six types of CDs, the yield of benzoin was the highest using HP-β-CD as cocatalysts, whereas that was the lowest using α-CD as cocatalysts. Efficacy of HP-β-CD was better than that of phase transfer agents such as TBAB, TMAB, ETPB and TBAHS.31 Since CDs played the role of phase-transfer catalysts, the effects of CDs on solubilization of benzaldehyde were also evaluated by phase-solubility methods. Fig. 2 showed that HP-β-CD displayed the most solubilization of benzaldehyde by 120-folds, which interpreted that the solubilization of benzaldehyde by CDs was one of the important factors to accelerate the condensation of benzaldehyde, and to increase reaction efficiency. Therefore, HP-β-CD as the cocatalyst was selected for optimizing the synthesis of benzoin.
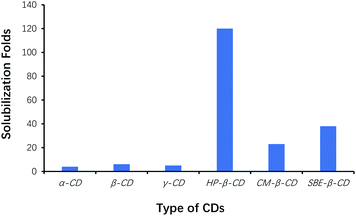 |
| Fig. 2 Effects of type of CDs on solubilization of benzaldehyde. The concentration of the saturated solutions of benzaldehyde in aqueous solution of CDs were detected by their UV-absorbances at 249 nm. | |
Effect of catalyst loading on the yield of benzoin
Organic molecules of benzaldehyde failed to completely enter the cavity of HP-β-CD when HP-β-CD was added in insufficient amounts, and most of benzaldehyde molecules remained in the organic phase. As shown in Fig. 3, the yield of benzoin gradually increased with the increase of the amount of HP-β-CD, and its maximum yield of benzoin appeared at around HP-β-CD loading 5% of benzaldehyde. When HP-β-CD loading continued to increase, the yield of benzoin almost remained unchanged, probably on account of the dynamic balance between reaction and complexation of benzaldehyde with HP-β-CD.
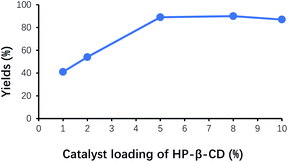 |
| Fig. 3 Effect of the amount of HP-β-CD on the yields of benzoin. | |
Effect of pH on the yield of benzoin
Under the alkaline condition, VB1 may be converted into the ylide by deprotonation, and catalyzes the benzoin condensation reaction. However, the structure of VB1 can be damaged under the strong alkaline, which makes reaction reproducibility poor. On the other hand, Cannizzaro reaction easily occurs under the strong alkaline condition, which makes reaction yield low. As shown in Fig. 4, the yield of benzoin gradually increased with the increase of pH, and its maximum yield of benzoin appeared at around pH 9.0–9.5. It is worth to note that the yield of benzoin decreased with pH values above 9.5.
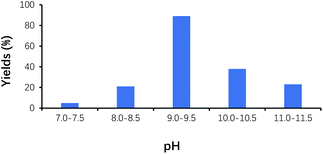 |
| Fig. 4 Effect of reaction pH on the yields of benzoin. | |
Effect of VB1 dosage on the yield of benzoin
The amount of VB1 on the yields of benzoin was shown in Fig. 5. With the increase of VB1 dosage, the yields of benzoin continuously increased. While the amount of VB1 exceeded 2.4 mmol (5% of benzaldehyde), the yield of benzoin did not display further improvement. Therefore, the suitable amount of VB1 under this experimental condition was 2.4 mmol (5% of benzaldehyde).
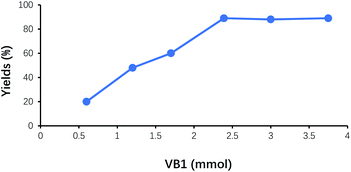 |
| Fig. 5 Effect of the amount of VB1 on the yields of benzoin. | |
Effect of reaction time on the yield of benzoin
Fig. 6 demonstrated the effect of the reaction time on the yields of benzoin. No product was observed at the beginning of 2 h. After that, the reaction solution became turbid, and the precipitate gradually formed. The yield of benzoin rapidly increased to 28% at 8 h, and its maximum yield appeared at around 7 days. While the reaction time exceeded 7 days, the yield of benzoin did not display further improvement. Therefore, 7 days was considered as the optimum reaction time.
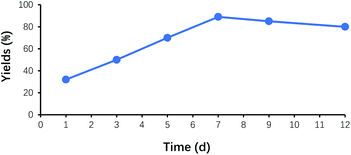 |
| Fig. 6 Effect of the reaction time on the yields of benzoin. | |
Effect of catalyst recycling times on the yield of benzoin
In the benzoin condensation reaction of benzaldehyde, VBl acted as the main catalyst, while HP-β-CD worked as the phase transfer catalyst. In principle, they can be recycled for catalyzing the reaction. As shown in Fig. 7(a), for the first catalyst recycling, the filtrate, obtained at the end of the initial experiment, was mixed with fresh benzaldehyde, and was adjusted to pH 8.5–9.5 with 2N of NaOH. As a result, the yield of benzoin was about 38% for the first round of catalyst recycling, and 7% for the second round of catalyst recycling, respectively. This observation could account for the fact that VB1 was unstable and destroyed under the alkaline conditions, and the amount of VB1 in each cycle greatly reduced. After two rounds of catalyst recycling, the remaining amount of VB1 could not effectively catalyze the reaction. Therefore, another strategy was applied for the experiment of catalyst recycling. As shown in Fig. 7(b), for each catalyst recycling, 30% additional amount of VB1 was added to the filtrate obtained from the last experiment, and next operations was same as the above procedures. After the experiment was repeated five times, the yield of benzoin remained around 80%, which indicated that HP-β-CD was stable under such experimental conditions. The decrease in yields might result from the loss of HP-β-CD, due to the multiple physical operations of the experiments, such as filtration and transfer.
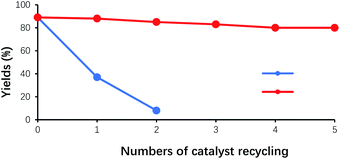 |
| Fig. 7 Effect of the numbers of HP-β-CD recycling on the yields of benzoin. (a) Not adding the fresh VB1 ( ); (b) adding the fresh VB1 each cycle ( ). | |
Application to the synthesis of the antiepileptic drug phenytoin
According to the above results, HP-β-CD, as the phase transfer catalyst, demonstrated the best catalytic activity for the synthesis of benzoin. Based on the optimized reaction conditions for the synthesis of benzoin, the antiepileptic drug phenytoin was synthesized through benzoin condensation, oxidation, and cyclization reactions by three kinds of protocols, i.e. in water, in organic solvents and in the aqueous solution of HP-β-CD (Scheme 3). As shown in Table 1, the total yield of phenytoin in water was about 22%. However, the total yield of phenytoin in the aqueous solution of HP-β-CD, up to 67%, was better than that in organic solvents. The results clearly showed that HP-β-CD did not act exclusively as the solubilizing agent.
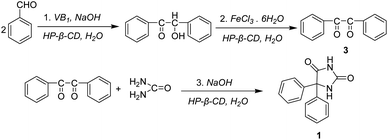 |
| Scheme 3 Synthesis of phenytoin catalyzed by HP-β-CD in water. | |
Table 1 Synthesis of phenytoin through three kinds of protocolsa
Compounds |
Isolated yields (%) |
a |
b |
c |
a, In water; b, in the organic solvents, benzoin in the ethanol, 1,2-diphenylethanedione in the glacial acetic acid and phenytoin in the ethanol; c, in the aqueous solution of HP-β-CD. |
Benzoin |
51 |
76 |
89 |
1,2-Diphenylethanedione |
90 |
98 |
94 |
Phenytoin |
48 |
75 |
80 |
Total yields of phenytoin |
22 |
56 |
67 |
Since HP-β-CD played one of the roles of phase-transfer catalysts, effects of HP-β-CD on solubilization of the reaction intermediates (benzoin and 1,2-diphenylethanedione) were also evaluated by phase-solubility methods (Fig. S2 and S3†). The experiments of phase solubility showed that benzoin was solubilized 37.2-folds by HP-β-CD, and 1,2-diphenylethanedione was solubilized 180-folds by HP-β-CD.
2D-ROESY NMR investigations for reaction mechanisms of phenytoin
HP-β-CD exhibited the highest yield in the benzoin condensation reactions, and was applied to the synthesis of the antiepileptic drug phenytoin through benzoin condensation, oxidation, and cyclization reactions in water. The solubilization of reactants by HP-β-CD was one of the important factors to accelerate the reactions, and to increase reaction efficiency, by forming the inclusion complexes of HP-β-CD with reactants. The formation of the inclusion complexes of HP-β-CD with reactants was characterized by the 1H NMR titration (Fig. S7–S9†). To reveal the plausible mechanisms of the reactions catalyzed by HP-β-CD in water, the geometries of inclusion complexes were investigated.
NMR is a powerful tool for studying the conformation of inclusion complexes,32 especially the 2D rotating frame 1H–1H nuclear Overhauser effect (2D-ROESY) because of its reliable and detailed information on the molecular geometry of those inclusion complexes.
In regard to the inclusion complexes between HP-β-CD and benzaldehyde (Fig. 8), the 2D-ROESY spectra contained crossing peaks between aromatic signals of the benzaldehyde and the signals of the HP-β-CD protons (H3 and H5) located inside the host cavity, indicating the formation of inclusion complexes. The possible inclusion geometry of the complexes can be deduced from the clear signals for the H5/Ha and H3/Hb crossing points. i.e. the Ha protons of benzaldehyde were near to H5 protons of HP-β-CD, and the Hb protons of benzaldehyde were close to H3 protons of HP-β-CD.
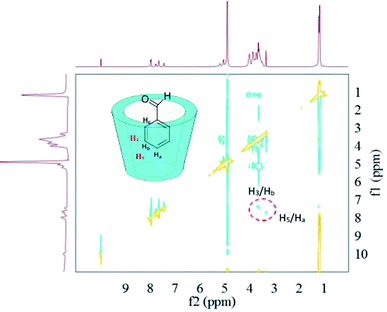 |
| Fig. 8 2D-ROESY map of the inclusion complexes of HP-β-CD with benzaldehyde. The 2D-ROESY spectra were acquired with 32 scans using a relaxation period of 2 s. | |
In the aspect of the inclusion complexes between HP-β-CD and benzoin (Fig. 9), the observation of the 2D-ROESY spectrum only showed a crossing peak between the aromatic proton (Ha) of the benzoin and the HP-β-CD protons (H3). The lack of crossing peaks for the H5 protons of HP-β-CD with any proton of benzoin suggested a complexation geometry where the guest molecule is partly included in the hydrophobic host cavity.
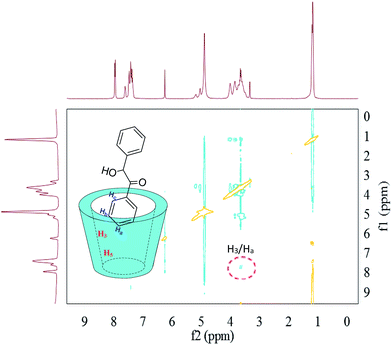 |
| Fig. 9 2D-ROESY map of the inclusion complexes of HP-β-CD with benzoin. The 2D-ROESY spectra were acquired with 32 scans using a relaxation period of 2 s. | |
In terms of the inclusion complexes between HP-β-CD and 1,2-diphenylethanedione (Fig. 10), it is worth to note that the 2D-ROESY spectrum gave two sets of crossing peaks between the aromatic signals of 1,2-diphenylethanedione and the signals of the HP-β-CD protons (H5/Hb and H5/Hc, H3/Hb and H3/Hc), which indicated that the aromatic ring of 1,2-diphenylethanedione was deeply included in the hydrophobic host cavity. Consistent with this hypothesis, the lack of crossing peaks for the Ha of 1,2-diphenylethanedione with any proton of HP-β-CD (H3 and H5) indicated that Ha was far enough from H3 and H5.
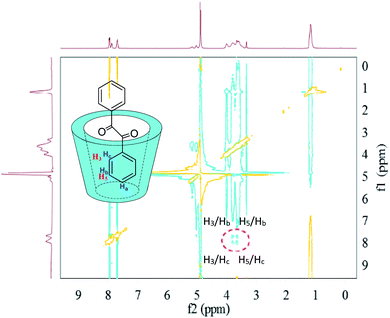 |
| Fig. 10 2D-ROESY map of the inclusion complexes of HP-β-CD with 1,2-diphenylethanedione. The 2D-ROESY spectra were acquired with 32 scans using a relaxation period of 2 s. | |
Based on the above results of 2D-ROESY spectra, there were differences in the depths of the introduction of aromatic rings into the host cavity, in order of depths (1,2-diphenylethanedione > benzaldehyde > benzoin), which was consistent with the order of solubilization by HP-β-CD, i.e., HP-β-CD could solubilize 1,2-diphenylethanedione by 180-folds, benzaldehyde by 120-folds, and benzoin by 37.2-folds, respectively.
According to the results of synthesis experiments and 2D-ROESY NMR investigations, a plausible reaction mechanism was proposed for the synthesis of phenytoin through benzoin condensation,33,34 oxidation, and cyclization reactions in water, using HP-β-CD as the cocatalysts (Fig. 11).
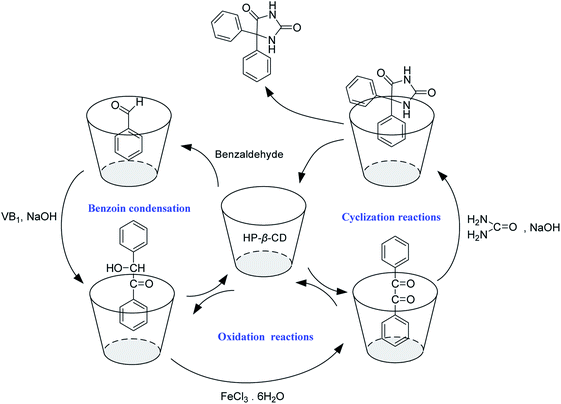 |
| Fig. 11 Proposed mechanisms of the reactions for the synthesis of phenytoin catalyzed by HP-β-CD in water. | |
Conclusions
CDs were used to mediate the benzoin condensation reaction in water. HP-β-CD displayed the best catalytic activity for the synthesis of benzoin, and could be recycled several times with little loss of activity through the addition of fresh VB1 solution. As an example of supramolecular catalysis, the methodology was applied to the “green” synthesis of the antiepileptic drug phenytoin through benzoin condensation, oxidation, and cyclization reactions in water under the presence of HP-β-CD, without the use of any harmful organic solvent. The solubilization of reactants by HP-β-CD was one of the important factors to accelerate the reactions, and to increase reaction efficiency, by forming the inclusion complexes of HP-β-CD with reactants. In addition, the 2D-ROESY NMR spectroscopies suggested the geometries of inclusion complexes between HP-β-CD and reactants, revealing the plausible mechanisms of the reactions. As the cocatalyst, HP-β-CD did not act exclusively as the solubilizing agent.
Author contributions
Conceptualization, Z. W.; methodology, Q. H.; software, H. C.; validation, Z. W. and Q. H.; formal analysis, H. C.; investigation, Z. J. and C. Y.; resources, Q. H.; data curation, H. C.; writing—original draft preparation, Z. J. and C. Y.; writing—review and editing, Q. H. and Z. J.; visualization, H. C.; supervision, Z. W.; project administration, Z. W.; funding acquisition, Q. H. and Z. W. All authors have read and agreed to the published version of the manuscript.
Conflicts of interest
There are no conflicts to declare.
Acknowledgements
This work was supported by the Key Research & Development Program of Ningxia (No. 2019BEG03064) and the Natural Science Foundation of China (No. 21666031 and 21506104), which are gratefully acknowledged.
Notes and references
- G. Gonzalez-Gaitano, J. R. Isasi, I. Velaz and A. Zornoza, Curr. Pharm. Des., 2017, 23, 411–432 CrossRef CAS PubMed.
- N. Sharma and A. Baldi, Drug Delivery, 2016, 23, 739–757 Search PubMed.
- P. Jansook, N. Ogawa and T. Loftsson, Int. J. Pharm., 2018, 535, 272–284 CrossRef CAS PubMed.
- G. Crini, Chem. Rev., 2014, 114, 10940–10975 CrossRef CAS PubMed.
- C. C. Bai, B. R. Tian, T. Zhao, Q. Huang and Z. Z. Wang, Molecules, 2017, 22, 1475 CrossRef PubMed.
- A. Patil, S. Gajare, G. Rashinkar and R. Salunkhe, Catal. Lett., 2020, 150, 127–137 CrossRef CAS.
- T. Ge, C. Zou and C. Zuo, Ind. Eng. Chem. Res., 2015, 54, 1723–1730 CrossRef CAS.
- S. V. Bhosale and S. V. Bhosale, Mini-Rev. Org. Chem., 2007, 4, 231–242 CrossRef CAS.
- W. Wang, Y. Wang, Z. Liu, Y. Han and C. Wang, Prog. Org. Coat., 2019, 136, 105294 CrossRef CAS.
- T. Fan, Z. Li, B. Cheng and J. Li, J. Membr. Sci., 2018, 556, 107–117 CrossRef CAS.
- L. Donnelly, J. G. Hardy, S. P. Gorman, D. S. Jones, N. J. Irwin and C. P. McCoy, Pharm. Res., 2017, 34, 1469–1476 CrossRef CAS PubMed.
- D. A. Albanese and N. Gaggero, Catalysts, 2018, 8, 181 CrossRef.
- F. Tamaddon and S. E. Tadayonfar, J. Mol. Liq., 2019, 280, 71–78 CrossRef CAS.
- T. Soeta, S. Mizuno, Y. Hatanaka and Y. Ukaji, Tetrahedron, 2017, 73, 3430–3437 CrossRef CAS.
- M. J. White and F. J. Leeper, J. Org. Chem., 2001, 66, 5124–5131 CrossRef CAS PubMed.
- J. H. Lee, J. H. Jang, N. Velusamy, H. S. Jung, S. Bhuniya and J. S. Kim, Chem. Commun., 2015, 51, 7709–7712 RSC.
- E. Rafiee, M. Joshaghani and P. Ghaderi-Shekhi Abadi, RSC Adv., 2015, 5, 74091–74101 RSC.
- K. Iwamoto, H. Kimura, M. Oike and M. Sato, Org. Biomol. Chem., 2008, 6, 912–915 RSC.
- K. Iwamoto, M. Hamaya, N. Hashimoto, H. Kimura, Y. Suzuki and M. Sato, Tetrahedron Lett., 2006, 47, 7175–7177 CrossRef CAS.
- R. Breslow and E. Kool, Tetrahedron Lett., 1988, 29, 1635–1638 CrossRef CAS.
- H. Ikeda, Y. Horimoto, M. Nakata and A. Ueno, Tetrahedron Lett., 2000, 41, 6483–6487 CrossRef CAS.
- S. Dharani, S. F. Barakh Ali, H. Afrooz, R. Bhattacharya, M. A. Khan and Z. Rahman, J. Pharm. Sci., 2019, 108, 1808–1817 CrossRef CAS PubMed.
- Z. Rahman, S. Dharani, S. F. Barakh Ali, M. T. H. Nutan and M. A. Khan, AAPS PharmSciTech, 2020, 21, 104 CrossRef CAS PubMed.
- T. T. Du, J. F. Li and L. J. Min, Adv. Mater. Res., 2012, 518–523, 3917–3920 CAS.
- A. Kadam, S. Jangam and R. Oswal, E-J. Chem., 2011, 8, S47–S52 CrossRef CAS.
- J. Safari and L. Javadian, RSC Adv., 2014, 4, 48973–48979 RSC.
- Y. Tang, Q. Cheng, S. Wang and J. Zhang, Monatsh. Chem., 2014, 145, 1501–1506 CrossRef CAS.
- L. Konnert, B. Reneaud, R. D. Figueiredo, J. M. Campagne, F. Lamaty, J. Martinez and E. Colacino, J. Org. Chem., 2014, 79, 10132–10142 CrossRef CAS PubMed.
- D. Sachdev and A. Dubey, Catal. Commun., 2010, 11, 1063–1067 CrossRef CAS.
- J. C. Sheehan and T. Hara, J. Org. Chem., 1974, 39, 1196–1199 CrossRef CAS.
- G. D. Yadav and A. A. Kadam, Org. Process Res. Dev., 2012, 16, 755–763 CrossRef CAS.
- A. Kasprzak, M. Koszytkowska-Stawińska, A. M. Nowicka, W. Buchowicz and M. Poplawska, J. Org. Chem., 2019, 84, 15900–15914 CrossRef CAS PubMed.
- R. S. Massey, J. Murray, C. J. Collett, J. Zhu, A. D. Smith and A. C. O'Donoghue, Org. Biomol. Chem., 2021, 19, 387–393 RSC.
- S. Yamabe and S. Yamazaki, Org. Biomol. Chem., 2009, 7, 951–961 RSC.
Footnote |
† Electronic supplementary information (ESI) available: Fig. S1–S3: UV-vis absorption spectra of compounds at various concentrations of HP-β-CD by the phase-solubility method. Fig. S4–S6: the raw 2D-ROESY map of the inclusion complexes of HP-β-CD with compounds. Fig. S7–S9: the 1H NMR titration of the inclusion complexes of HP-β-CD with compounds. See DOI: 10.1039/d1ra09062c |
|
This journal is © The Royal Society of Chemistry 2022 |
Click here to see how this site uses Cookies. View our privacy policy here.