DOI:
10.1039/D2RA00212D
(Paper)
RSC Adv., 2022,
12, 10219-10236
Metal-bio functionalized bismuthmagnetite [Fe3−xBixO4/SiO2@L-ArgEt3+I−/Zn(II)]: a novel bionanocomposite for the synthesis of 1,2,4,5-tetrahydro-2,4-dioxobenzo[b][1,4]diazepine malononitriles and malonamides at room temperature and under sonication†
Received
11th January 2022
, Accepted 13th March 2022
First published on 1st April 2022
Abstract
In this work, a new magnetized composite of bismuth (Fe3−xBixO4) was prepared and functionalized stepwise with silica, triethylargininium iodide ionic liquid, and Zn(II) to prepare a multi-layered core–shell bio-nanostructure, [Fe3−xBixO4/SiO2@L-ArgEt3+I−/Zn(II)]. The modified bismuth magnetic amino acid-containing nanocomposite was characterized using several techniques including Fourier-transform infrared (FT-IR), X-ray fluorescence (XRF), vibrating sample magnetometer (VSM), field-emission scanning electron microscopy (FESEM), energy dispersive X-ray analysis (EDAX), thermogravimetric/differential scanning calorimetric (TGA/DSC) analysis, X-ray photoelectron spectroscopy (XPS), Brunauer–Emmett–Teller (BET), and inductively coupled plasma-optical emission spectrometry (ICP-OES). The magnetized bionanocomposite exhibited high catalytic activity for the synthesis of 1,2,4,5-tetrahydro-2,4-dioxobenzo[b][1,4]diazepine malononitriles via five-component reactions between 1,2-phenylenediamines, Meldrum's acid, malononitrile, aldehydes, and isocyanides at room temperature in ethanol. The efficacy of this protocol was also examined to obtain malonamide derivatives via pseudo six-component reactions of 1,4-phenylenediamine, Meldrum's acid, malononitrile, aldehydes, and isocyanides. When the above-mentioned MCRs were repeated under the same conditions with the application of sonication, a notable decrease in the reaction time was observed. The recovery and reusability of the metal-bio functionalized bismuthmagnetite were examined successfully in 3 runs. Furthermore, the characteristics of the recovered Fe3−xBixO4/SiO2@L-ArgEt3+I−/Zn(II) were investigated though FESEM and EDAX analysis.
Introduction
Multi-component reactions (MCRs) are versatile strategies in organic synthesis, which utilize more than two different starting substances to prepare a single product. The first MCR was accomplished by Strecker in 1850, resulting in the formation of α-amino nitriles. Subsequently, the role of MCRs in the preparation of various classes of organic compounds increased rapidly. In fact, MCRs, as smart types of transformations resulted in the evolution of organic synthesis and sustainable chemistry.1–3 MCRs are also versatile tools in drug discovery and medicinal chemistry,4 asymmetric synthesis,5 preparation of bioactive molecules,6 ligation and bioconjugation chemistry,7 synthesis of polyheterocycles and complex molecules,8 peptide macrocyclization and stapling,9 and steroid diversification.10 Another remarkable feature of MCRs is that the combination of more than two MCRs, which leads to a union of MCRs, multiplies their efficacy.11 In fact, the occurrence of union MCRs increases the possibility of the one-pot synthesis of more substrates to obtain a single complex product in an economical and environment-friendly procedure.
The preeminent efficacy of MCRs in comparison to stepwise reactions is due to some notable points such as their simple operation via a one-pot reaction, atom-economy, high chemoselectivity and/or stereoselectivity, reduced energy consumption, waste reduction by avoiding the purification and separation of intermediates, short procedures, slight protection and deprotection of functional groups, and high yields of the main product without the formation of by-products.12
Benzodiazpines (BDZs) are important bicyclic aromatic heterocyclic scaffolds made up of a benzene ring fused to a seven-membered ring containing two nitrogen atoms. Based on the positions of the nitrogen atoms, many types of benzodiazpines exist such as 1,2-BDZs, 1,3-BDZs, 1,4-BDZs, 1,5-BDZs, and 2,3-BDZs.13 Various classes of organics and functionalized heterocycles, which include diverse types of BDZ motifs, play critical role in medicinal chemistry, pharmacology, and treatment.14 BDZ is a key synthon in different therapeutic chemicals and drugs, which possess a wide range of properties such as antiarrhythmic,15 antidepressant,16 anticonvulsant,17 analgesic,18 and antituberculosis.19 Alprazolam (a short-acting tranquilizer), bromazepam (for anxiety treatment), brotizolam (used for short-term treatment of severe insomnia), and lorazepam (used to treat anxiety disorders and sleeping troubles) are some drugs that possess a benzodiazepine skeleton.
Malonamide derivatives (MDs) have gained significant attention due to their interesting properties in various fields of science and technology.20 MDs are important organics in the fields of medicinal chemistry, drugs, and biology as antiinflammatories,21 potent agonists of TGR5 (which are promising molecular targets for metabolic diseases),22 selective κ opioid receptor agonists,23 potent α-glucosidase inhibitors,24 γ-secretase inhibitors for the potential treatment of Alzheimer's disease,25 and antibiotics against methicillin-resistant Staphylococcus aureus.26 Various classes of compounds bearing malonamide building blocks act as gelators,27 extractors in separation technology,28 materials for the recovery of rare-earth metals from end-of-life products (lamp phosphors),29 and stabilizers for nitrocellulose-based propellants.30
Magnetized nanostructures can be prepared via the metal doping of magnetite with the general formula of Fe3−xMxO4 (M = Ni, Zn, Mg, Co, Mg, Ta, etc.) employing various methods. In 1998, a crystalline film of spinel Fe3−xMxO4 (M = Ni and Zn) was prepared through a spine-spray ferrite plating method and its crystallographic and magnetic properties were studied.31a The Opel group reported thin films of the ferrimagnetic spinel oxide ZnxFe3−xO4 as spintronic materials with tunable electrical and magnetic properties.31b The Alrozi group, in 2019, utilized an Fe3−xMnxO4–MKSF composite catalyst for the degradation of acid orange II dye thorough a heterogeneous Fenton-like reaction.31c The He group, in 2013, studied the valence and site occupancy of substituted metals in the magnetite spinel structure Fe3−xMxO4 (M = Cr, Mn, Co, and Ni) and their influence on its thermal stability.31d The Gusevskaya research group, in 2004, reported the oxidation of β-pinene in the presence of Fe3−xMxO4 (M = Co and Mn) as heterogenous catalysts.31e He's group, in 2009, examined the decolorization of methylene blue via a heterogeneous Fenton reaction using Fe3−xTixO4 at neutral pH.32 Some catalytic systems also have been applied based on a titanomagnetite (Fe3−xTixO4) core to promote organic transformations.33 The Walz group, in 2002, studied some characteristics of Fe3−xMxO4 (M = Ni, Mg, Co, Al, Ti, and Ga) via magnetic after-effect (MAE) spectroscopy.34 However, although the investigations on Fe3−xMxO4 with different metals are comprehensive, in the literature, there are no reports on Fe3−xBixO4. Sun's group, in 2019, prepared and characterized bismuth-doped Ni–Cu–Co nano ferrites (Ni0.2Cu0.2Co0.6Fe2−xBixO4) via sol–gel auto-combustion technology.35 Praveena et al., in 2014, synthesized NiFe2−xBixO4 nanopowder via a chemical co-precipitation method.36
It should be mentioned that some composites have been prepared by loading bismuth on magnetite. For example, Cai's research group, in 2017, prepared a Bi/Fe3O4 composite through a one-pot process from ferrous sulfate and bismuth chloride using hydrazine hydrate as a reducing agent. They examined its catalytic performances for 4-nitrophenol reduction.37a Hasanpour's group, in 2013, synthesized a Bi–Fe3O4 nanocomposite mechanochemically and investigated its dielectric behavior.37b Gao and coworkers, in 2015, loaded bismuth and Fe3O4 nanoparticles on reduced graphene oxide to fabricate Bi–Fe3O4@RGO hybrids, which catalyzed the reduction of 4-nitrophenol.37c
Silica-coated magnetized nanostructures have attracted increasing attention in multiple fields of science and technology.38 Pratapa's group, in 2019, prepared amorphous-silica-coated magnetite-nanoparticle (Fe3O4/α-SiO2) composites via co-precipitation and modified Stöber methods, respectively.39a These nanocomposites have been utilized in various aspects of applied technologies such as dye removal as sorbents,39b carriers of hydrophilic polymer as high-density polymer brush shell,39c protein isolation,39d antimicrobial agents,39e rewarming organs through nanowarming technology,39f and bifunctional agents for magnetic resonance imaging and Zn(II) fluorescent sensing.39g
Recently, promoting different MCRs in the presence of various catalytic systems containing silica-coated magnetized cores has gained considerable interest. In 2017 Mobinikhaledi's group reported the use of sodium polyaspartate-functionalized silica-coated magnetite nanoparticles (MNPs-SPAsp) as heterogeneous and reusable catalysts for the solvent-free synthesis of 2-amino-4H-chromenes.40a Shirini et al., in 2020, utilized silica-coated magnetic nanoparticles containing bis dicationic bridge (γ-Fe2O3@SiO2@[Bis-APTES]Cl2) for the synthesis of 1,2,4-triazolo pyrimidine/quinazolinone derivatives.40b In 2021, Baharfar prepared indol-3-yl-4H-chromenes in the presence of Fe3O4@SiO2@D-NHCS-Tr under solvent-free conditions.40c Mao's group immobilized phospholipase D on silica-coated magnetic nanoparticles to obtain functional phosphatidylserines.40d In 2021, Gholizadeh and coworkers applied copper-functionalized silica-coated magnetic nanoparticles for an efficient Suzuki cross-coupling reaction.40e
Currently, bio-based nanostructures and nanocomposites are important in various scientific and technological fields. They possess broad-range of applications in medicine, packaging, consumer goods, electronics, transportation, construction, and green processes.41a The antimicrobial, industrial, and biomedical applications of gum bio-based nanocomposites were reported in 2019.41b Edible bio-based nanostructures (as nanocarriers of bioactive compounds to specific body sites) and their delivery, absorption and potential toxicity were also previously discussed.41c Some nanostructured bio-based carbon electrodes were exploited for energy storage applications.41d They were also utilized in cancer therapy and oncotherapy due to their potent properties such as autologous pharmaceutical and synergetic effects, biocompatibility, biodegradability and biosafety.41e
Amino acids are a great and attractive class of bio-based compounds, which play a critical role in organic and green chemistry. Many types of catalytic systems such as amino acidic-based ionic liquids (AAILs) and amino acid-containing bionanocomposites have been reported to promote various types of MCRs and other applications in science and technology.42
Arginine (Arg), a charged aliphatic amino acid at physiological pH, is a semi-essential α-amino acid utilized in the biosynthesis of proteins. Thus, arginine and arginine-containing structures are significant in variant application fields. A vast range of ligands and composites possessing Arg motifs are used as surfactants,43 sticky protein equivalents for viable cell accommodation,44 and membranes for carbon capture45 and ion removal from water.46
Green chemistry is a valuable environmentally friendly concept in many branches of science and technology. Based on the 12 principles introduced and explained by Anastas and Warner in 1998 for the first time, the enlarged “green chemisTREE” was introduced in 2018 and the concept of “periodic table of green and sustainable chemistry” was recommended in 2019.47 Nowadays, due to the critical role of green chemistry, different research, data, and discoveries form various scientific and operational perspectives such as green techniques to perform reactions48 utilizing green catalysts and reagents in chemical transformations, drug delivery, and medicine,49 and solvent-free or green-media reactions.50
In continuation of our interest in preparing novel magnetized nanostructures to promote MCRs under green conditions,51 herein, we report the preparation of a novel magnetized multi-layered core–shell bionanostructure, [Fe3−xBixO4/SiO2@L-ArgEt3+I−/Zn(II)]. The organic–inorganic bio-hybrid obtained forms the Fe3−xBixO4 core, which was functionalized stepwise through the immobilization of silica, triethylargininium iodide ionic liquid, and Zn(II) (Scheme 1). The synthesized nanostructure was examined as a heterogenous catalyst for the synthesis of 2,4,5-tetrahydro-2,4-dioxobenzo[b][1,4]diazepine malononitriles and malonamides in ethanol at room temperature (method A) and also in the presence of ultrasound irradiation (method B) (Scheme 2).
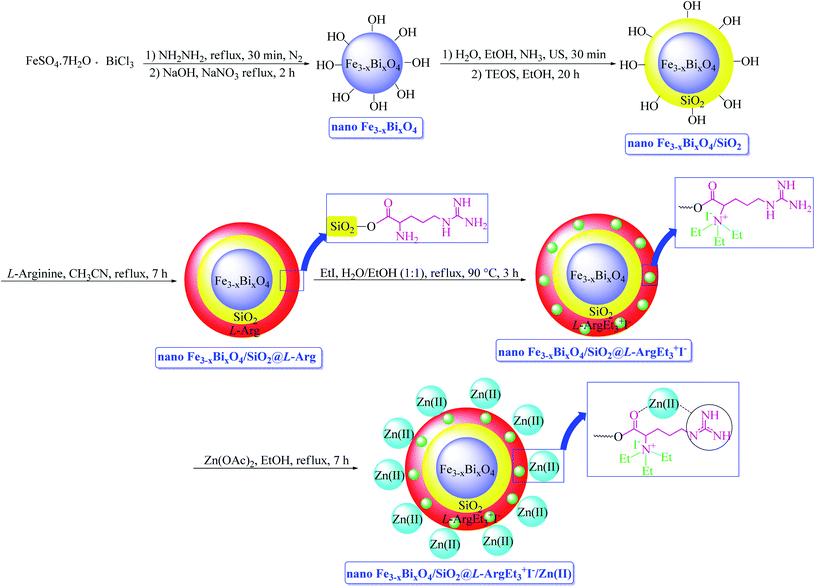 |
| Scheme 1 Synthesis of Fe3−xBixO4/SiO2@L-ArgEt3+I−/Zn(II) bionanocomposite. | |
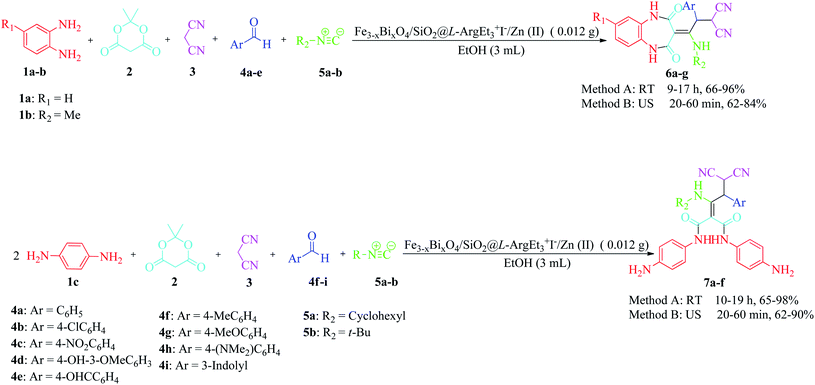 |
| Scheme 2 Synthesis of 4,5-tetrahydro-2,4-dioxobenzo[b][1,4]diazepine malononitriles 6a–g and malonamides 7a–f in the presence of nano Fe3−xBixO4/SiO2@L-ArgEt3+I−/Zn(II). | |
Results and discussion
Characterization of the bionanocomposite
The FT-IR spectra of Fe3−xBixO4 (a), Fe3−xBixO4/SiO2 (b), Fe3−xBixO4/SiO2@L-Arg (c), Fe3−xBixO4/SiO2@L-ArgEt3+I− (d), and Fe3−xBixO4/SiO2@L-ArgEt3+I−/Zn(II) (e) are presented in Fig. 1. According to Fig. 1a, the peak at 563 cm−1 and 844 cm−1 are attributed to the stretching vibration of the Fe–O bond and symmetrical stretching vibration of the Bi–O bonds, respectively. The very weak peak at 1047 cm−1 can be ascribed to some other vibration of Bi–O caused through the interaction between the Bi–O bonds and their surroundings. The broad band at 3440 cm−1 and the peak at 1633 cm−1 are related to the stretching and bending vibrations of the hydroxyl group, respectively.52 As shown in Fig. 1b, the appearance of bands at 1085 cm−1 (Si–O–Si stretching vibrations), 790 cm−1 (Si–O–Si bending vibrations), and 466 cm−1 (Si–O–Si rocking vibrations) confirmed the embedding of silica on the bismuthmagnetite core.53 The peaks at 3253 cm−1 and 3122 cm−1 (stretching vibration of NH and NH2 bonds), 1647 cm−1 (bending vibration of N–H), 1679 cm−1 (C
O stretching vibration), and 1112 cm−1 (C–N stretching vibration), as shown in Fig. 1c, confirmed the presence of L-Arginine on the surface of Fe3−xBixO4/SiO2@L-Arg. As shown in Fig. 1d, the weakening of the bands in the region of the N–H stretching vibrations represented the settling of EtI on the structure. According to Fig. 1e, the shifting the peaks of the C
O stretching vibrations form 1679 cm−1 to 1656 cm−1 confirmed the coordination of Zn(II) to L-ArgEt3+I−, as the outer layer of the bionanocomposite.
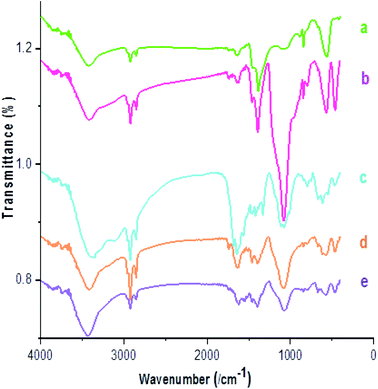 |
| Fig. 1 FT-IR spectra of (a) Fe3−xBixO4, (b) Fe3−xBixO4/SiO2, (c) Fe3−xBixO4/SiO2@L-Arg, (d) Fe3−xBixO4/SiO2@L-ArgEt3+I−, and (e) Fe3−xBixO4/SiO2@L-ArgEt3+I−/Zn(II). | |
The EDAX analysis of the Fe3−xBixO4 core in Fig. 2 (top) revealed the presence of iron (29.96 wt%), bismuth (41.66 wt%), and oxygen (28.38 wt%). No other impurities based on the compounds utilized during the preparation procedure were observed. The bottom diagram in Fig. 2 confirms that Fe3−xBixO4/SiO2@L-ArgEt3+I−/Zn(II) is made up of iron (17.02 wt%), bismuth (39.84 wt%), oxygen (26.16 wt%), silicon (6.05 wt%), carbon (7.08 wt%), nitrogen (0.31 wt%), iodine (1.34 wt%), and zinc (2.20 wt%). These results confirm the successful preparation of the bio multi-layered Fe3−xBixO4/SiO2@L-ArgEt3+I−/Zn(II) nanostructure. In addition, the ICP-OES analysis demonstrated that the catalyst contains 30
963.3 ppm of Zn (3.096% Zn or 0.473 mmol Zn per 1 g of catalyst).
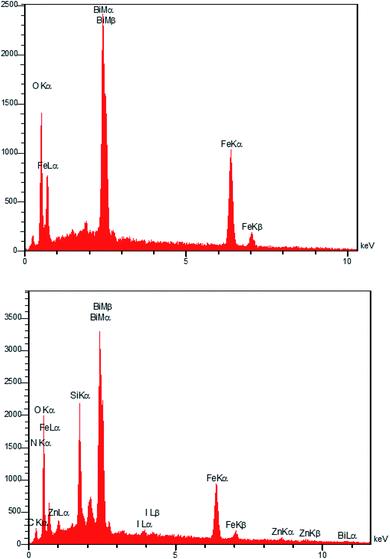 |
| Fig. 2 EDAX analysis of Fe3−xBixO4 (top) and Fe3−xBixO4/SiO2@L-ArgEt3+I−/Zn(II) (bottom). | |
The FESEM images of the Fe3−xBixO4 core (top) and the final magnetized bionanocomposite Fe3−xBixO4/SiO2@L-ArgEt3+I−/Zn(II) (bottom) are illustrated in Fig. 3. According to the top images, the core structure exhibited semi-uniform nano-sized particles with an average size of 25–45 nm. The final nanostructure consisted of more uniform semi-spherical and pseudo-filamentary nano-sized particles with an average size of 15–20 nm. Generally, complete uniformity was not observed in the morphology of the nanostructures throughout the bismuthmagnetite core and the final bionanocomposite.
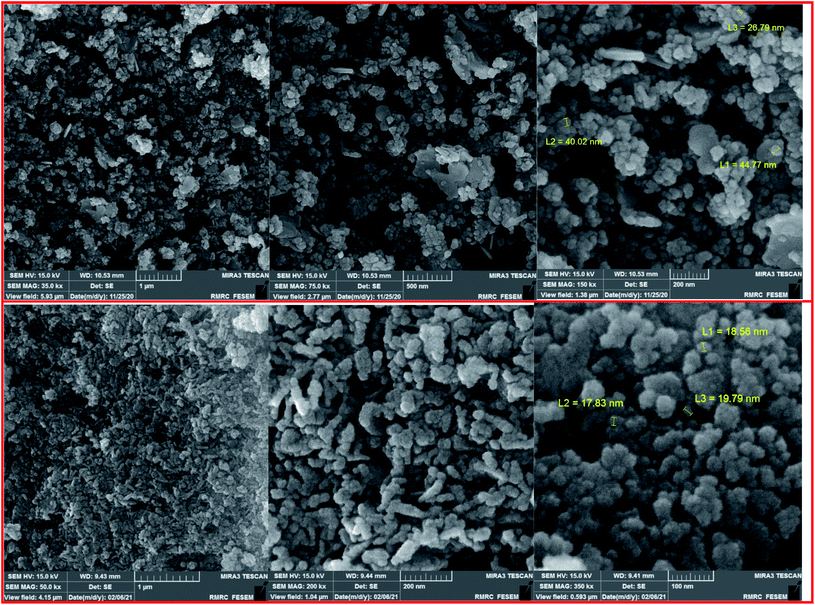 |
| Fig. 3 FESEM images of Fe3−xBixO4 (top) and Fe3−xBixO4/SiO2@L-ArgEt3+I−/Zn(II) (bottom). | |
The XRF analysis of the Fe3−xBixO4 core exhibited the presence of Fe2O3 (16.394%) and Bi (82.565%), confirming the successful synthesis of bismuthmagnetite (Table 1).
Table 1 XRF analysis of Fe3−xBixO4 core
|
Fe2O3 |
Bi |
LOI |
(%) |
16.394 |
82.565 |
0.2953 |
The thermal behavior of the Fe3−xBixO4 MNPs and Fe3−xBixO4/SiO2@L-ArgEt3+I−/Zn(II) nanocomposite was investigated via TGA/DSC up to 1000 °C, as shown in Fig. 4. Based on the top diagram, which relates to the Fe3−xBixO4 core, the initial endothermic weight loss occurred at 260–400 °C (8%). The second weight loss of about 13.3% was observed at 830–870 °C. Heating the Fe3−xBixO4 core up to 1000 °C yielded the endothermic total weight loss of 31.38%. Thus, the core is almost completely thermally stable up to 400 °C. The TGA/DSC curves of Fe3−xBixO4/SiO2@L-ArgEt3+I−/Zn(II) in bottom diagram of Fig. 4 demonstrate the first mass loss of about 2.2% at 150 °C. The second mass loss observed at 267 °C was about 5.2%, corresponding to the decomposition of the outer layers of the bionanocomposite, which are organic compounds (the L-ArgEt3+I− IL). At 710 °C the total wight loss of about 20% was observed, and at 800 °C, the total weight loss was 21.8%. All the steps are endothermic and the wight changes occurred continuously with a mild decrease.
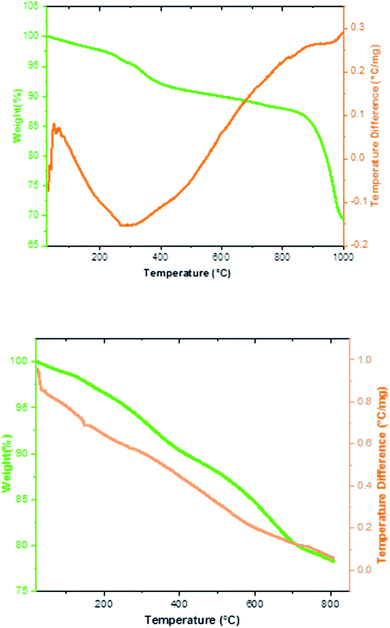 |
| Fig. 4 TGA/DSC curves of Fe3−xBixO4 (top) and Fe3−xBixO4/SiO2@L-ArgEt3+I−/Zn(II) (bottom). | |
The magnetic characteristics of the Fe3−xBixO4 core and each shell of the bionanostructure Fe3−xBixO4/SiO2@L-ArgEt3+I−/Zn(II) were studied through VSM, as shown in Fig. 5. According to the data, the curves belong to (a) Fe3−xBixO4, (b) Fe3−xBixO4/SiO2, (c) Fe3−xBixO4/SiO2@L-Arg, (d) Fe3−xBixO4/SiO2@L-ArgEt3+I−, and (e) Fe3−xBixO4/SiO2@L-ArgEt3+I−/Zn(II), exhibiting the saturation magnetization values of 18.508 emu g−1, 16.603 emu g−1, 7.701 emu g−1, 11.010 emu g−1, and 11.691 emu g−1, respectively. The results confirmed that not only the core but also the final bionanocomposite possessed magnetic properties. Embedding the silica and L-Arginine layers on the core decreased its magnetization due to the covering of the magnetized core with diamagnetic layers. Surprisingly, creating the L-ArgEt3+I− ionic liquid increased the saturation magnetization value (curve d) in comparison to the former layer (curve c). This increase was also observed by embedding Zn(II) on the outer layer of the bionanocomposite (curve e).
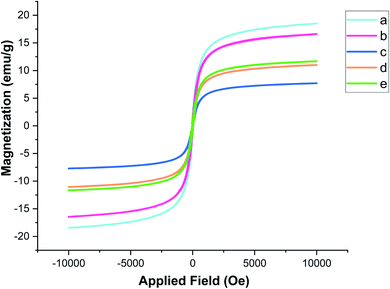 |
| Fig. 5 Magnetization curves of (a) Fe3−xBixO4, (b) Fe3−xBixO4/SiO2, (c) Fe3−xBixO4/SiO2@L-Arg, (d) Fe3−xBixO4/SiO2@L-ArgEt3+I− and (e) Fe3−xBixO4/SiO2@L-ArgEt3+I−/Zn(II). | |
X-ray photoelectron spectroscopy (XPS) was performed on the bionanocomposite to clarify its chemical composition and elemental valence states (Fig. 6). In the survey spectrum of the sample (Fig. 6a), the presence of Si, C, N, Bi, O, Fe, Zn, and I in Fe3−xBixO4/SiO2@L-ArgEt3+I−/Zn(II) was confirmed through the appearance of peaks at the binding energies of 102 eV (Si 2p), 163 eV (Bi 4f), 287 eV (C 1s), 399 eV (N 1s), 439 eV (Bi 4d), 531 (O 1s), 628 (I 3d), 678 (Bi 4p), 726 (Fe 2p), 1022 (Zn 2p3/2), and 1045 (Zn 2p1/2). The atomic ratio of the elements of the bionanostructure are Zn (12.02%), Si (6.02%), Fe (49.22%), I (0.43%), and Bi (5.40%). The chemical states of Fe, Bi, O, Si, N, I, Zn, and C are displayed in their corresponding XPS spectra, as shown in Fig. 6b–i, respectively. According to Fig. 6b, the peak of Fe 2p3/2 at 710.15 eV revealed that both Fe2+ and Fe3+ are present in the bismuthmagnetite core. According to the previous reports, the binding energy of Fe 2p3/2 is about 709 eV for Fe2+ and 711 eV for Fe3+.54 The Fe 2p1/2 peak appeared at 722.89 eV. The Fe 2p1/2 and Fe 2p3/2 peaks of elemental iron [Fe(0)] should appear at 720 eV and 707 eV, respectively.55 Thus, there was no Fe(0) in the bionanocomposite. According to Fig. 6c, the relative symmetrical peaks at 441.58 eV (Bi 4d5/2) and 465.15 eV (Bi 4d3/2) with the splitting of 23.57 eV confirmed the presence of Bi(III) in the structure.56 According to the peak at 531.17 eV in Fig. 6d, the O2− species is present in the structure.57 The Si 2p peak in Fig. 6e at the binding energy of 102.129 eV confirmed the presence of SiO2 in the bionanocomposite. Fig. 6f presents the detailed XPS analysis of N 1s. According to the data, the peaks at 394.06 eV (C–N
), 398.74 eV (C–N), and 403.50 (C
N) verified the presence of the argininium core in the IL layer of the bionanocomposite.58 However, the peak in the region of 401.5–405 eV, which is related to the interactions of carbon and nitrogen, could be affected by another neighboring nitrogen.59 The absence of a peak at 399.4 eV, which corresponds to the NH2 group,60 can be due to the ammonium triethyl iodide moiety of the IL, and also the coordination of the nitrogen part of the ionic liquid with the Zn(II) outer layer of the bionanocomposite. The two peaks at 618.63 eV (I 3d5/2) and 603.72 eV (I 3d3/2) in Fig. 6g validate the presence of I+ in the nanostructure.61 As shown in Fig. 6h, the peaks at 1022.48 eV (Zn 2p3/2) and 1045.57 eV (Zn 2p1/2) are attributed the presence of Zn2+ in the bionanocomposite. The characteristic peak of elemental Zn at 1021 eV was not observed.62 As shown in Fig. 6i, in the C 1s diagram, the peaks at 284.01 eV, 285.62 eV, and 287.67 eV correspond to the sp2 carbon, sp3 carbon, and the C–N and C
O moieties, respectively.63
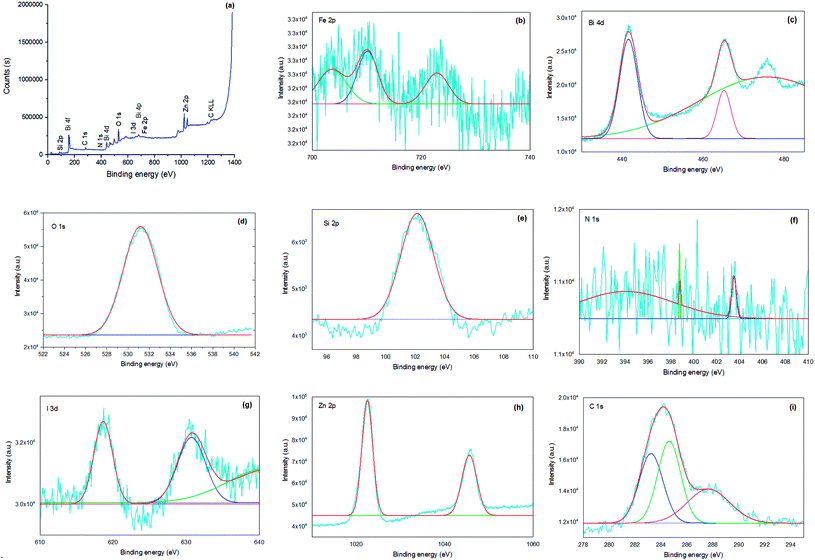 |
| Fig. 6 XPS survey spectra of Fe3−xBixO4-SiO2@L-ArgEt3+I− Zn(II) (a) and high-resolution XPS spectra of Fe 2p (b), Bi 4d (c), O 1s (d), Si 2p (e), N 1s (f), I 3d (g), Zn 2p (h), and C 1s (i). | |
The porous nature of the prepared nano Fe3−xBixO4/SiO2@L-ArgEt3+I−/Zn(II) was investigated via N2 adsorption/desorption isotherm (top) and pore size distribution (bottom) measurements, as shown in Fig. 7. According to the ADS/DES isotherm (top), the Fe3−xBixO4/SiO2@L-ArgEt3+I−/Zn(II) nanostructure can be classified as a type III isotherm (according to IUPAC classification), which illustrated a type H3 hysteresis loop in the p/p0 range of 0.55–0.97, corresponding to slit-shaped pores. The physicochemical properties of the nanostructure are as follows: BET surface area (as,BET) = 12.687 m2 g−1, total pore volume = 0.1699 cm3 g−1, and mean pore diameter = 53.58 nm. The data from the BJH pot (down) are rp,peak (area) = 7.99 nm, ap = 15.43 m2 g−1, and vp = 0.1705 cm3 g−1.
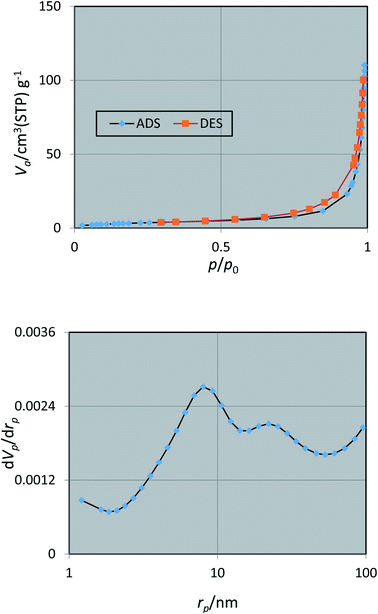 |
| Fig. 7 Nitrogen adsorption/desorption isotherms (top) and pore size distribution (down) of nano Fe3−xBixO4/SiO2@L-ArgEt3+I−/Zn(II). | |
Investigation of the catalytic activity of the nano Fe3−xBixO4/SiO2@L-ArgEt3+I−/Zn(II)
To optimize the reaction conditions, the five-component one-pot reaction of 1,2-phenylenediamine (1a, 1 mmol), Meldrum's acid (2, 1.2 mmol), malononitrile (3, 1.2 mmol), 4-nitrobenzaldehyde (4c, 1.2 mmol) and cyclohexyl isocyanide (5a, 1.2 mmol) was chosen as a model reaction. According to the data in Table 2, different parameters were checked to obtain 2-(2-(cyclohexylamino)-2-(1,2,4,5-tetrahydro-2,4-dioxobenzo[b][1,4]diazepin-3-ylidene)-1-(4-nitrophenyl)ethyl)malononitrile (6d). The reaction was examined under solvent-free conditions (entries 1 and 2) and also utilizing H2O and EtOH (entries 3 and 4, respectively). The results showed that EtOH seems to be a good choice. Performing the reaction at 60 °C did not produce good results (entry 2). The catalyst amount was another investigated parameter (entries 5–8). The presence of 0.012 g of nano Fe3−xBixO4/SiO2@L-Arg-Et3+I−/Zn(II) was sufficient (entry 8). To detect the effect of the mixed green solvent of EtOH–H2O (1
:
1), it was examined at room temperature and at 50 °C (entries 9 and 10, respectively). Polyethylene glycol was another solvent choice that did not produce a good result (entry 11). To check the media temperature, the preparation of 6d was examined in an ice-bath, which did not yield any product (entry 12). It must be mentioned that in each case, the reaction was carried out for up to 24 h, but no further improvement occurred after the reported durations, as shown in Table 2.
Table 2 Screening the reaction parameters for the synthesis of 6d
To evaluate the effect of the presence of the bionanocomposite on promoting the model reaction, the preparation of the product 6d was examined in the absence of the catalyst, and also each layer of Fe3−xBixO4/SiO2@L-ArgEt3+I−/Zn(II) under the optimized reaction conditions for 9 h. The data is presented in Table 3. The model reaction was also examined in the presence of the L-ArgEt3+I− ionic liquid (entry 7) and only EtI (entry 8). According to the data, in the case of using the ionic liquid, many by-products were observed during the reaction (9 h). The observations confirmed that the presence of each shell on the bismuthmagnetite core affected the reaction progress. This can be ascribed to the combined catalytic effect of each component in the whole bionanocomposite, which synergically extended its catalytic potential.
Table 3 Investigation of the catalytic role of Fe3−xBixO4-SiO2@L-ArgEt3+I−/Zn(II) in the synthesis of 6d
Entry |
Catalyst (0.012 g)/EtOH (3 mL)/rt |
Yield (%) |
1 |
— |
∼5 |
2 |
Fe3−xBixO4 |
12 |
3 |
Fe3−xBixO4/SiO2 |
23 |
4 |
Fe3−xBixO4/SiO2@L-Arg |
30 |
5 |
Fe3−xBixO4/SiO2@L-ArgEt3+I− |
42 |
6 |
Fe3−xBixO4/SiO2@L-ArgEt3+I−/Zn(II) |
83 |
7 |
L-ArgEt3+I− |
10 |
8 |
EtI |
— |
Based on the optimized reaction conditions, different derivatives of the 1,2,4,5-tetrahydro-2,4-dioxobenzo[b][1,4]diazepine malononitrile were successfully prepared according to the data in Table 4 (method A). 1,2-Phenylenediamine 1a reacted with benzaldehyde and its derivatives (4a–d) in the presence of cyclohexyl isocyanide 5a to obtain their corresponding products within 9–17 h in 66–91% yield (entries 1, 2, 4, and 5, respectively). t-Butyl isocyanide 5b achieved satisfactory results as another alkyl isocyanide candidate (entry 3). Terephthalaldehyde, as a bifunctional aldehyde resulted in the formation of 2-(2-(cyclohexylamino)-2-(2,4-dioxo-4,5-dihydro-1H-benzo[b][1,4]diazepin-3(2H)-ylidene)-1-(4-formylphenyl)ethyl)malononitrile 6f chemoselectively, as only one of the aldehydic moieties reacted (entry 6). The reaction was performed in the presence of 1a, 2, 3, 4i, and 5a (in a 1
:
1.2
:
1.2
:
0.6
:
1.2 molar ratio) and nano Fe3−xBixO4/SiO2@L-ArgEt3+I−/Zn(II) (0.012 g). Performing the reaction in the presence of 4-methyl-1,2-phenylenediamine 1b yielded 6g successfully. To further improve the results, the reaction was examined under sonication and the results are summarized in Table 3 (method B). As seen, the reaction times decreased significantly (20–60 min), but an overall increase in the yield was not observed, which can be explained by the cavitation effects of ultrasound. The known compounds were characterized by comparing the obtained data with their authentic reports.64 The spectral data of the new derivatives (6e and 6f) are presented in the Experimental section.
Table 4 Synthesis of 1,2,4,5-tetrahydro-2,4-dioxobenzo[b][1,4]diazepine malononitriles 6a–g catalyzed by nano Fe3−xBixO4/SiO2@L-ArgEt3+I−/Zn(II) (0.012 g) in EtOH (3 mL)
Entry |
Product |
Method A |
Method B |
Time (h)/yield (%) |
Time (min)/yield (%) |
1 |
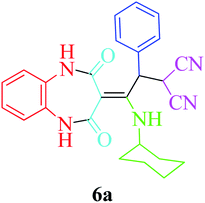 |
13/91 |
50/62 |
2 |
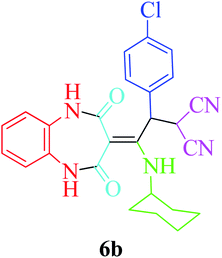 |
15/66 |
50/72 |
3 |
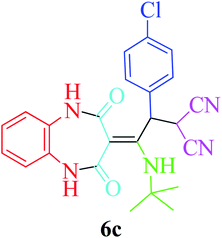 |
15/72 |
50/74 |
4 |
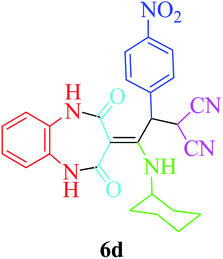 |
9/83 |
60/71 |
5 |
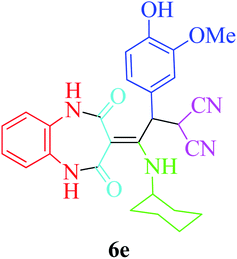 |
17/73 |
50/84 |
6 |
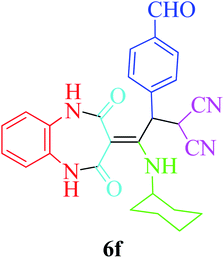 |
17/77 |
20/63 |
7 |
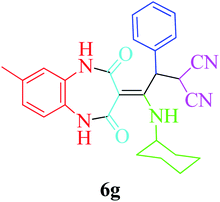 |
12/96 |
20/73 |
Furthermore, to extend the efficacy of the novel nano Fe3−xBixO4/SiO2@L-ArgEt3+I−/Zn(II) the preparation of malonamides 7a–f through a pseudo six-component condensation was performed successfully under the optimal reaction conditions within 10–19 h in 65–98% yield Table 5 (method A). Heterocycles 7a–e were obtained through the reaction of 1,4-phenylenediamine 1c, Meldrum's acid 2, malononitrile 3, aldehydes 4f–h, and isocyanides 5a and b (entries 1–5). Indole-3-carbaldehyde 4i, as a fused bicyclic heteroaromatic aldehyde candidate, achieved the corresponding adduct 7f within 17 h (entry 6). Concerning the results, performing the reactions under sonication decreased the duration notably (Table 5, method B). Also, no significant substituent effect was demonstrated. The known compounds were characterized by comparing their data with the literature.64 The spectral data of product 7f is displayed in the Experimental section.
Table 5 Synthesis of malonamides 7a–f in the presence of nano Fe3−xBixO4/SiO2@L-ArgEt3+I−/Zn(II) (0.012 g) in EtOH (3 mL)
Entry |
Product |
Method A |
Method B |
Time (h)/yield (%) |
Time (min)/yield (%) |
1 |
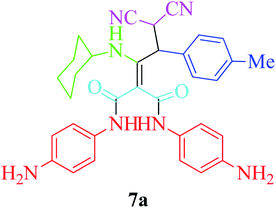 |
19/72 |
50/87 |
2 |
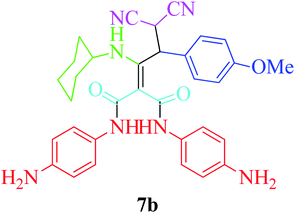 |
10/98 |
50/82 |
3 |
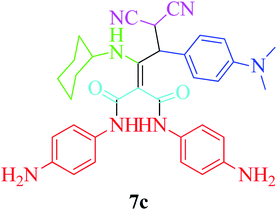 |
10/91 |
20/90 |
4 |
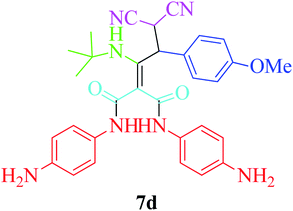 |
10/98 |
40/70 |
5 |
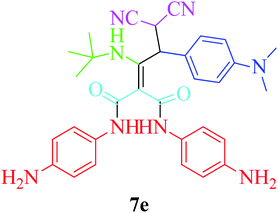 |
12/72 |
50/71 |
6 |
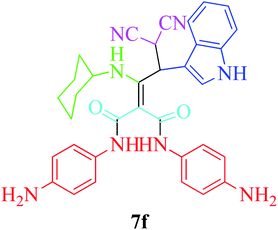 |
17/65 |
60/62 |
To clarify the possibility of recycling and reusing the bionanostructure, the model reaction was examined with 2 runs. Product 4d was obtained in 82% and 80% yield in the first and second runs, respectively. After each cycle, the bio nano core–shell was separated with an external magnet, washed with MeOH (2 × 3 mL), and air-dried. The reused catalyst was characterized thought EDAX analysis (Fig. 8) and FESEM imaging (Fig. 9). The EDAX data confirmed that Fe3−xBixO4/SiO2@L-ArgEt3+I−/Zn(II) consisted of iron (17.91 wt%), bismuth (30.45 wt%), oxygen (23.09 wt%), silicon (16.25 wt%), carbon (9.05 wt%), nitrogen (3.16 wt%), iodine (0.08 wt%), and zinc (0.13 wt%). The FESEM spectra also demonstrated that some agglomeration happed in the bionanocomposite compared to the fresh nanocatalyst. The average size of the nanoparticles reached 30–40 nm.
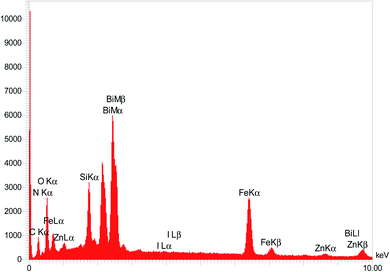 |
| Fig. 8 EDAX analysis of the reused Fe3−xBixO4/SiO2@L-ArgEt3+I−/Zn(II). | |
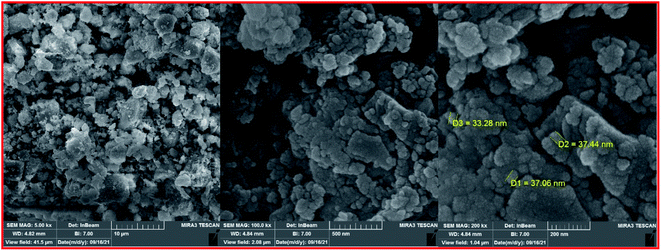 |
| Fig. 9 FESEM images of the recovered Fe3−xBixO4/SiO2@L-ArgEt3+I−/Zn(II). | |
The probable mechanism for the synthesis of 1,2,4,5-tetrahydro-2,4-dioxobenzo[b][1,4]diazepine derivatives 6 is shown in Scheme 3. The condensation reaction of 1,2-phenylenediamines 1 with Meldrum's acid 2 in the presence of nano Fe3−xBixO4-SiO2@L-ArgEt3+I−/Zn(II) through release of acetone and aqua formed the intermediates 3-((2-aminophenyl)amino)-3-oxopropanoic acid A and benzodiazepine-2,4-dione B, respectively. Alternatively, 2-(arylomethylene)malononitrile C was formed via nucleophilic attack of malononitrile 3 to activated aldehyde 4 through a condensation reaction. The nucleophilic attack of isocyanide 5 to C via a Michel-type addition reaction led to intermediate D, which absorbed a proton from the acidic methylene of B to form dioxo-tetrahydro-1H-benzodiazepin-3-ides E and F, respectively. The nucleophilic attack of E to F yielded G, which followed by [1,3]-H shift (analogues to imine-enamine tautomerization), produced the final products 6.
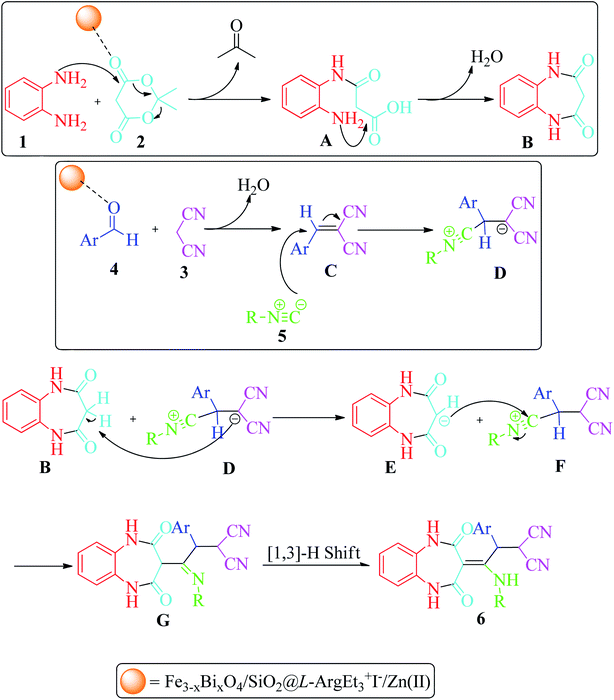 |
| Scheme 3 Proposed mechanism for the formation of 1,2,4,5-tetrahydro-2,4-dioxobenzo[b][1,4]diazepines. | |
In the final part, the comparison of the data for the synthesis of 2-(2-(cyclohexylamino)-2-(2,4-dioxo-4,5-dihydro-1H-benzo[b][1,4]diazepin-3(2H)-ylidene)-1-(4-nitrophenyl)ethyl)malononitrile 6d is provided in Table 6. The significant feature of the current report is the remarkable reduction in the reaction time, not only with the traditional stirring method but also under sonication (comparing entries 2 and 3 with 1). The yield enhancement is another noteworthy aspect of the present procedure. Performing the mentioned MCR in green media (EtOH) is another important feature of this report compared to the previous route (which utilized CH2Cl2 as the solvent).
Table 6 Comparison of the synthesis of 6d with the previously reported procedure
Entry |
Conditions |
Time/yield (%) |
Ref. |
1 |
CH2Cl2/rt |
24 h/48 |
60 |
2 |
Fe3−xBixO4/SiO2@L-ArgEt3+I−/Zn(II) (0.012 g)/EtOH (3 mL)/rt |
9 h/83 |
This work |
3 |
Fe3−xBixO4/SiO2@L-ArgEt3+I−/Zn(II) (0.012 g)/EtOH (3 mL)/rt/Us |
60 min/71 |
This work |
Experimental
General
The utilized chemicals were obtained from Aldrich, Merck, and Alfa-Aesar and used as received without further purification. Melting points were obtained using an Electrothermal 9200 apparatus and reported uncorrected. FT-IR spectra were obtained using KBr disks of the samples on a Bruker FT-IR (Tensor 27) spectrometer. Magnetization properties were measured using a VSM (LBKFB model) apparatus. Homogenization of the nanostructure was performed in a WiseClean (power of 90 W). Morphology and size were estimated via FESEM (VEGA\\TESCAN-LMU). Mass spectra were obtained using a Gc-Mass 5973 network mass-selective detector on a Gc6690 Agilent device. The EDAX analysis was performed using a TESCAN MIRA III machine. Elemental investigations were performed using a Philips-PW2404 XRF spectrometer. A UNIVERSAL 320 centrifuge apparatus (5000–10
000 rpm) was utilized for the preparation of the nanostructure. The XPS analysis was performed using a PHOIBOS machine with a Zr X-ray source (1486.71 eV). The peak fitting was performed using SpecsLab Prodigy Version 4.43.2-r73078. BET measurements were performed on a Belsorp Mini Japan, Finetech 40 point apparatus. The ICP-OES analysis was done using a Spectro Arcos instrument. Sonication was performed using an HD 3100 ultrasonic homogenizer (Bandelin Company, Germany). A standard horn SH 70 G emitting 20 kHz ± 500 Hz ultrasound at intensity levels tunable up to a maximum sonic power density of 100 W cm−2 was used. Sonication was carried out at 36% amplitude. An MS73 probe of 13 mm diameter was immersed directly into the reaction mixture.
Synthetic procedure for Fe3−xBixO4/SiO2@L-ArgEt3+I−/Zn(II) bionanocomposite
Synthesis of nano bismuthmagnetite (Fe3−xBixO4). The bismuthmagnetite nanostructure was prepared through a modified precipitation–oxidation method, which was reported previously for the synthesis of Fe3−xCrxO4.65 In a typical procedure, solutions of FeSO4·7H2O (0.9 M) and BiCl3 (0.9 M) were prepared in HCl solution (1 M). In a flask, to 10 mL of the prepared solution of Fe2+ and Bi3+ in equal amounts, hydrazine hydrate (1 mL) was added to reach pH < 1 to prevent the oxidation of Fe2+ and hydroxide precipitation. The resulting solution was refluxed for 30 min at 90 °C under an N2 atmosphere (to prevent oxidation of iron by air). In the next step, a mixture of NaOH (4 M, 5 mL) and NaNO3 (0.9 M, 5 mL) was added dropwise (within 5 min) to the reaction mixture and stirred magnetically for 2 h at 90 °C. The mixture was cooled, the residue separated through an external magnet and washed with water (3 × 10 mL). Air-drying the solid for 10 h yielded Fe3−xBixO4 as a black solid.
Preparation of nano Fe3−xBixO4/SiO2. The process was accomplished through a modified procedure.51e Typically, a mixture of Fe3−xBixO4 nanoparticles (1 g), was dissolved in a mixture of 25 wt% ammonia (2 mL), deionized water (20 mL) and absolute ethanol (60 mL) and sonicated in a sonic-bath for 30 min. Then a solution TEOS (0.5 mL) in absolute ethanol (1 mL) was added dropwise to the above mixture and stirred continuously for 20 h at ambient temperature. Finally, the was solid separated using an external magnet and washed with absolute ethanol (3 × 5 mL). The product was dried at 70 °C for 5 h to produce nano Fe3−xBixO4/SiO2.
Embedding L-Arginine amino acid on nano Fe3−xBixO4/SiO2 layer (Fe3−xBixO4/SiO2@L-Arg). To a mixture of nano Fe3−xBixO4/SiO2 (1 g) and L-arginine (1 g) in acetonitrile (10 mL), diluted H2SO4 (0.01 M) was added dropwise to regulate the pH to ∼4. The mixture was refluxed for 7 h. The residue was separated using an external magnet and washed with a mixture of acetonitrile/absolute ethanol (1
:
1) (2 × 10 mL). The obtained solid was dried at 100 °C for 4 h to get Fe3−xBixO4/SiO2@L-Arg.
In situ fabrication of triethylargininium iodide on the silicated-bismuthmagnetite core (Fe3−xBixO4/SiO2@L-ArgEt3+I−). A mixture of Fe3−xBixO4/SiO2@L-Arg nanocomposite (1 g) and ethyl iodide (2 g) in a water/ethanol (1
:
1) mixture (20 mL) was refluxed at 90 °C for 3 h. After completion and cooling, the residue was separated using an external magnet and washed with water/absolute ethanol (1
:
1) (3 × 10 mL). The black solid was dried at 50 °C for 2 h to obtain Fe3−xBixO4/SiO2@L-ArgEt3+I−.
Preparation of nano Fe3−xBixO4/SiO2@L-ArgEt3+I−/Zn(II). A mixture of Fe3−xBixO4/SiO2@L-ArgEt3+I− nanostructure (1 g) and zinc acetate (1 g) in absolute ethanol (20 mL) was refluxed for 7 h. Next, the solid was separated using an external magnet and washed with absolute ethanol (3 × 10 mL). After air-drying the solid residue for 2 h followed by heating in an oven 50 °C for 2 h, the final bionanocomposite Fe3−xBixO4/SiO2@L-ArgEt3+I−/Zn(II) was obtained.
Preparation of L-ArgEt3+I− ionic liquid. The ionic liquid was prepared based on a previously reported procedure with some modifications.33c To a mixture of L-arginine (1.74 g, 10 mmol) in a water/absolute ethanol mixture (1
:
1) (6 mL), EtI (4.679 g, 30 mmol) was added and refluxed for 7 h. Then, the mixture was cooled to room temperature. The mixture was concentrated through mild heating, followed by the addition of MeOH (5 mL), vaporizing, and air-drying. The pure L-ArgEt3+I− ionic liquid was obtained as a pale blue viscous liquid. IR (KBr, cm−1): 3336, 3161, 2968, 1647, 1454, 1396, 1043, 871, 790, 663, 549. 1H NMR (300 MHz, DMSO-d6): 0.98 (brs, 9H, 3CH3), 1.11 (m, 2H, CH2), 1.52 (m, 2H, CH2), 2.49 (brs, 1H, NH), 2.50–3.09 (m, 4H, CH2), 3.36–3.9 (m, 5H, 2CH2 + CH), 7.44 (brs, 4H, NH + OH). 13C NMR (75 MHz, DMSO-d6): 11.33, 14.00, 18.63, 24.83, 26.55, 41.15, 45.05, 48.74, 56.17, 157.12, 171.48. MS (ESI) m/z 386 [M]+, 357 ([M]+–Et), 343 ([M]+–Me, –Et), 327 ([M]+–2Et), 283 ([M]+–3Et, –CO2H), 267 ([M]+–2Et, –Me, –CO2H), 253 ([M]+–3Et, –CO2H), 175 [Arg]+, 156 [Arg–NH2]+, 113 [Arg–NH2, –CO2]+, 100 [NHEt3]+, 84 [CH2CH2NHCNHNH2]+, 56 [guanidinium]+, 44 [CO2].
General procedure for the synthesis of 1,2,4,5-tetrahydro-2,4-dioxobenzo[b][1,4]diazepine malononitriles 6a–g via five-component reaction
To a solution of aromatic 1,2-diamines (1a–b, 1 mmol), Meldrum's acid (2, 1.2 mmol), malononitrile (3, 1.2 mmol), aromatic aldehydes (4a–e, 1.2 mmol), and isocyanides (5a–b, 1.2 mmol) in ethanol (3 mL), the nano Fe3−xBixO4/SiO2@L-ArgEt3+I−/Zn(II) (0.012 g) was added. This mixture was stirred magnetically at room temperature (method A) or under sonication (100 W cm−2) (method B) for the appropriate time and monitored by TLC (n-hexane/EtOAc 1
:
1). After completion, the bionanocatalyst was separated using an external magnet. The pure products 6a–g were obtained by preparative thin-layer chromatography (PLC).
2-(2-(Cyclohexylamino)-2-(2,4-dioxo-4,5-dihydro-1H-benzo[b][1,4]diazepin-3(2H)-ylidene)-1-(4-hydroxy-3-methoxyphenyl)ethyl)malononitrile (6e). Brown powder; m. p. = 80 °C; IR (KBr): 3445, 2926, 2854, 2194, 1736, 1700, 1650, 1559, 1521, 1457, 1396, 1339, 1034, 818, 748 cm−1. 1H NMR (300 MHz, DMSO-d6) δ: 0.9–1.22 (m, 6H, 3CH2), 1.39–1.63 (m, 4H, 2CH2), 1.92–2.00 (m, 1H, CH), 2.89 (brs, 1H, NH), 3.32 (s, 3H, OMe), 3.67–3.71 (m, 1H, CH), 3.92–3.97 (m, 1H, CH), 6.52–6.76 (m, 2H, Ar), 6.80–7.13 (m, 5H, Ar), 7.41 (brs, 1H, NH), 7.52–7.73 (m, 1H, OH), 7.99 (brs, 1H, NH). MS (ESI) m/z 485 [M]+, 355 ([M]+–cyclohexyl, –NH2, –OMe), 327 ([M]+–(methoxyphenyl)propanenitrilium), 281 ([M]+–hydroxy-methoxybenzylmalononitrile), 266 ([M]+–cyclohexyl, –NH2, –methoxyphenol), 237 ([M]+–cyclohexyl, –NH2, –methoxyphenol, –CN), 210 ([M]+–cyclohexyl, –NH2, –methoxyphenol, –2CN), 182 [methylene-1H-benzo[b][1,4]diazepine-2,4(3H,5H)-dione]+, 141 [methoxymethylphenol]+, 115 [N-methylcyclohexanamine]+, 98 [cyclohexanamine]+, 67 [malononitrile]+.
2-(2-(Cyclohexylamino)-2-(2,4-dioxo-4,5-dihydro-1H-benzo[b][1,4]diazepin-3(2H)-ylidene)-1-(4-formylphenyl)ethyl)malononitrile (6f). Cream powder; m. p. = 130 °C; IR (KBr): 3423, 2929, 2856, 1638, 1454, 1370, 1316, 1103, 898, 799, 750, 580, 468 cm−1;. 1H NMR (300 MHz, DMSO-d6) δ: 0.9–1.34 (m, 6H, 3CH2), 1.37–1.63 (m, 4H, 2CH2), 1.92–2.07 (m, 1H, CH), 2.82 (brs, 1H, NH), 3.16–3.67 (m, 2H, CH), 6.99–7.18 (m, 4H, Ar), 7.31–7.45 (m, 1H, Ar), 7.52–7.55 (m, 3H, Ar), 8.00 (brs, 1H, NH), 8.16 (brs, 1H, NH), 8.18 (s, 1H, aldehydic). MS (ESI) m/z 467 [M]+, 428 ([M]+–CH2CN), 405 ([M]+–CHCN2), 377 ([M]+–aniline), 357 ([M]+–phenylenediamine), 331 ([M]+–2CN, –cyclohexyl), 316 ([M]+–cyclohexylamine, –2CN), 206 [ethyl-1H-benzo[b][1,4]diazepine-2,4(3H,5H)-dione]+, 189 [methyl-1H-benzo[b][1,4]diazepine-2,4(3H,5H)-dione]+, 133 [ethylbenzaldehyde]+, 92 [toluene]+.
General procedure for the synthesis malonamides 7a–f via pseudo six-component reaction
To a solution of para phenylenediamine (1c, 2 mmol), Meldrum's acid (2, 1 mmol), malononitrile (3, 1.1 mmol), aromatic aldehydes (4f–i, 1.1 mmol), isocyanides (5a–b, 1 mmol) in ethanol (3 mL), nano Fe3−xBixO4/SiO2@L-ArgEt3+I−/Zn(II) (0.012 g) was added and the was mixture stirred at room temperature (method A) or under ultrasonication (100 W cm−2) (method B). After completion of the reaction, as observed by TLC (n-hexane/EtOAc 1
:
1), the catalyst was separated using an external magnet. The pure products 7a–f were obtained via the PLC technique.
N1,N3-Bis(4-aminophenyl)-2-(3,3-dicyano-1-(cyclohexylamino)-2-(1H-indol-3-yl)propylidene)malonamide (7f). Brown powder; m. p. = 145 °C; IR (KBr): 3567, 3421, 2925, 2854, 2169, 1650, 1542, 1514, 1421, 1069, 830, 745, 592, 516, 471, 520 cm−1. 1H NMR (300 MHz, DMSO-d6) δ: 0.84–1.9 (m, 10H, 5CH2), 3.74–3.83 (m, 3H, CH), 4.78–4.79 (brs, 4H, 2NH2), 6.13–6.55 (m, 7H, Ar), 6.56–6.71 (m, 3H, Ar), 6.90–6.99 (m, 3H, Ar), 7.47 (s, 1H, NH), 7.80–7.94 (m, 1H, NH), 10.84 (brs, 1H, NH), 10.87 (brs, 1H, NH). MS (ESI) m/z 586 [M]+, 405 ([M]+–CHCN2, –indolyl), 346 ([M]+–CO, –2phenylediamine), 331 ([M]+–phenylenediamine, –CO, –indolyl), 254 ([M]+–phenylenediamine, –CO, –indolyl, –cyclohexyl), 127 [N-ethylcyclohexanamine]+, 117 [indolyl]+, 108 [phenylenediamine]+, 99[cyclohexylamine]+, 91[aniline]+.
Conclusions
In summary, a novel magnetic inorganic–bioorganic nanocomposite, Fe3−xBixO4/SiO2@L-ArgEt3+I−/Zn(II), was successfully obtained via a multi-step simple synthetic procedure, which was characterized by the FT-IR, XRF, VSM, FESEM, EDAX, TGA/DSC, XPS, BET, and ICP-OES techniques. The nanoscale biocomposite was made up of a bismuthmagnetite core, which was covered by silica, triethylargininium iodide IL, and Zn(II), respectively. The catalytic performance of the multi-layered core–shell structure was investigated for the synthesis of 1,2,4,5-tetrahydro-2,4-dioxobenzo[b][1,4]diazepine malononitriles via five-component reactions and also malonamide derivatives via pseudo six-component reactions at room temperature in ethanol. The examination of the two above-mentioned MCRs under sonication resulted in significant reduction in the reaction time. The recovery and reusability of the bionanocomposite were also checked successfully within 3 runs, and its FESEM and EDAX analysis demonstrated the stability of its structure.
Conflicts of interest
There are no conflicts to declare.
Acknowledgements
The authors thank to Alzahra University for support of this work.
References
- A. Strecker, Justus Liebigs Ann. Chem., 1850, 75, 27–45 CrossRef.
-
(a) V. V. Kouznetsov and C. E. P. Galvi, Tetrahedron, 2018, 74, 773–810 CrossRef CAS;
(b) L. Weber, Drug Discovery Today, 2002, 7, 143–147 CrossRef CAS PubMed.
-
(a) H. Eckert, Molecules, 2012, 17, 1074–1102 CrossRef CAS PubMed;
(b) S. Brauch, S. S. van Berkel and B. Westermann, Chem. Soc. Rev., 2013, 42, 4948–4962 RSC;
(c) B. H. Rotstein, S. Zaretsky, V. Rai and A. K. Yudin, Chem. Rev., 2014, 114(16), 8323–8359 CrossRef CAS PubMed;
(d) R. C. Cioc, E. Ruijter and R. V. A. Orru, Green Chem., 2014, 16, 2958–2975 RSC.
-
(a) E. Ruijter and R. V. A. Orru, Drug Discovery Today, 2018, 29, 1–2 CrossRef PubMed;
(b) H. A. Younus, M. Al-Rashida, A. Hameed, M. Uroos, U. Salar, S. Rana and Kh. M. Khan, Expert Opin. Ther. Pat., 2021, 31, 267–289 CrossRef CAS PubMed.
-
(a) T. Ahmadi, G. Mohammadi Ziarani, P. Gholamzadeh and H. Mollabagher, Tetrahedron: Asymmetry, 2017, 28, 708–724 CrossRef CAS;
(b) P. S. G. Nunes, H. D. A. Vidal and A. G. Corrêa, Org. Biomol. Chem., 2020, 18, 7751–7773 RSC.
-
(a) P. Brandão, C. Marques, A. J. Burke and M. Pineiro, Eur. J. Med. Chem., 2021, 211, 113102 CrossRef PubMed;
(b) C. S. Graebin, F. V. Ribeiro, K. R. Rogério and A. E. Kümmerle, Curr. Org. Synth., 2019, 16, 855–899 CrossRef CAS PubMed;
(c) D. Insuasty, J. Castillo, D. Becerra, H. Rojas and R. Abonia, Molecules, 2020, 25, 505–575 CrossRef PubMed;
(d) N. J. Poonam, S. Dhadda and D. K. Jangid, ChemistrySelect, 2022, 7, e202103139 Search PubMed.
- L. Reguera, Y. Méndez, A. R. Humpierre, O. Valdés and D. G. Rivera, Acc. Chem. Res., 2018, 51, 1475–1486 CrossRef CAS PubMed.
-
(a) I. A. Ibarra, A. Islas-Jácome and E. González-Zamora, Org. Biomol. Chem., 2018, 16, 1402–1418 RSC;
(b) S. Zhi, X. Ma and W. Zhang, Org. Biomol. Chem., 2019, 17, 7632–7650 RSC;
(c) V. G. Nenajdenko, Russ. Chem. Rev., 2020, 89, 1274–1336 CrossRef CAS;
(d) F. Molaei Yielzoleh and K. Nikoofar, Curr. Green Chem., 2021, 8, 127–146 CrossRef;
(e) K. Nikoofar and F. Molaei Yielzoleh, Curr. Org. Synth., 2022, 19, 115–147 CrossRef PubMed;
(f) N. Kerru, L. Gummidi, S. Maddila and S. B. Jonnalagadda, Curr. Org. Chem., 2021, 25, 4–39 CrossRef CAS.
- L. Reguera and D. G. Rivera, Chem. Rev., 2019, 119, 9836–9860 CrossRef CAS PubMed.
- L. Reguera, C. I. Attorresi, J. A. Ramírez and D. G. Rivera, Beilstein J. Org. Chem., 2019, 15, 1236–1256 CrossRef CAS PubMed.
-
(a) T. Zarganes-Tzitzikas, A. L. Chandgude and A. Dömling, Chem. Rec., 2015, 15, 981–996 CrossRef CAS PubMed;
(b) N. Elders, D. van der Born, L. J. D. Hendrickx, B. J. J. Timmer, A. Krause, E. Janssen, F. J. J. de Kanter, E. Ruijter and R. V. A. Orru, Angew. Chem., Int. Ed., 2009, 121, 5970–5973 CrossRef.
-
(a) B. Jiang, T. Rajale, W. Wever, Sh.-J. Tu and G. Li, Chem.–Asian J., 2010, 5, 2318–2335 CrossRef CAS PubMed;
(b) O. Ghashghaei, F. Seghetti and R. Lavilla, Beilstein J. Org. Chem., 2019, 15, 521–534 CrossRef CAS PubMed;
(c) I. Ugi, A. Dömling and W. Hörl, Endeavour, 1994, 18, 115–122 CrossRef CAS;
(d) G. van der Heijden, E. Ruijter and R. V. A. Orru, Synlett, 2013, 24, 666–685 CrossRef CAS.
-
(a) Sh. Verma and S. Kumar, Mini-Rev. Org. Chem., 2017, 14, 453–468 CAS;
(b) L. H. Sternbach, J. Med. Chem., 1979, 22, 1–7 CrossRef CAS PubMed.
-
(a) N. Arora, P. Dhiman, Sh. Kumar, G. Sing and V. Monga, Bioorg. Chem., 2020, 97, 103668 CrossRef CAS PubMed;
(b) C. Bellantuono, V. Reggi, G. Tognoni and S. Garattini, Drugs, 1980, 19, 195–219 CrossRef CAS PubMed;
(c) A. V. da Silva, S. M. P. Meneghetti and M. R. Meneghetti, Molecules, 2021, 26, 2796–2811 CrossRef CAS PubMed.
- R. E. Johnson, E. R. Baizman, C. Becker, E. A. Bohnet, R. H. Bell, N. C. Birsner, C. A. Busacca, Ph. M. Carabateas and Ch. C. Chadwick, J. Med. Chem., 1993, 36, 3361–3370 CrossRef CAS PubMed.
- F. Petty, M. H. Trivedi, M. Fulton and A. J. Rush, Biol. Psychiatry, 1995, 38, 578–591 CrossRef CAS PubMed.
- J. R. M. Haigh and M. Feely, Trends Pharmacol. Sci., 1988, 9, 361–366 CrossRef CAS PubMed.
- S. Reddy and R. B. Patt, J. Pain Symptom Manage., 1994, 9, 510–514 CrossRef CAS PubMed.
- M. M. K. Kumar, T. Mohan, G. K. Mai, G. P. V. Sangeeta and K. P. Nagasree, J. Young Pharm., 2018, 10, 267–271 CrossRef CAS.
- A. D. Aderibigbe and D. P. Day, Chemsitryselect, 2020, 5, 15222–15232 CrossRef CAS.
- T. Katagi, M. Aoki, M. Kashiwagi, K. Ohata, S. Kohno, T. Murata and T. Inoi, Chem. Pharm. Bull., 1985, 33, 4878–4888 CrossRef CAS PubMed.
- E. J. Park, Y. G. Ahn, S. H. Jung, H. J. Bang, M. Kim, D. J. Hong, J. Kim, K. H. Suh, Y. J. Kim, D. Kim, E. Kim, K. Lee and K. H. Min, Bioorg. Med. Chem. Lett., 2014, 24, 4271–4275 CrossRef CAS PubMed.
- G. Chu, M. Gu, J. A. Cassel, S. Belanger, T. M. Graczyk, R. N. DeHaven, N. Conway-James, M. Koblish, P. J. Little, D. L. De Haven-Hudkins and R. E. Dolle, Bioorg. Med. Chem. Lett., 2007, 17, 1951–1955 CrossRef CAS PubMed.
-
(a) M. Sh. Islam, A. Barakat, A. M. Al-Majid, H. A. Ghabbour, A. F. M. M. Rahman, K. Javaid, R. Imad, S. Yousuf and M. I. Choudhary, Bioorg. Med. Chem., 2016, 24, 1675–1682 CrossRef CAS PubMed;
(b) A. Barakat, M. Sh. Islam, A. M. Al-Majid, S. M. Soliman, H. A. Ghabbour, S. Yousuf, M. I. Choudhary and Z. Ul-Haq, J. Mol. Struct., 2017, 1134, 253–264 CrossRef CAS.
- M. G. Yang, J.-L. Shi, D. P. Modi, J. Wells, B. M. Cochran, M. A. Wolf, L. A. Thompson, M. M. Ramanjulu, A. H. Roach, R. Zaczek, D. W. Robertson, R. R. Wexler and R. E. Olson, Bioorg. Med. Chem. Lett., 2007, 17, 3910–3915 CrossRef CAS PubMed.
- J.-C. Su, Y.-T. Huang, C.-S. Chen, H.-C. Chiu and C.-W. Shiau, Molecules, 2018, 23, 27–40 CrossRef PubMed.
- M. Jokic, V. Caplar, T. Portada, J. Makarevic, N. S. Vujicic and M. Zinic, Tetrahedron Lett., 2009, 50, 509–513 CrossRef CAS.
-
(a) F. Testard, P. Bauduin, L. Martinet, B. Abécassis, L. Berthon, C. Madic and T. Zemb, Radiochim. Acta, 2008, 96, 265–272 CrossRef CAS;
(b) A. Vansteene, J.-P. Jasmin, G. Cote and C. Mariet, Ind. Eng. Chem. Res., 2018, 57, 11572–11582 CrossRef CAS.
- R. Boyd, C. Carroll, S. Dandil, C. Acikgoz, R. Ruhela and P. Nockemann, ACS Sustainable Chem. Eng., 2020, 8, 18706–18711 CrossRef CAS.
- G. Li, B. J. Z. Chai, L. Liao, Sh. Chu and R. Peng, Polym. Test., 2020, 86, 106493 CrossRef CAS.
-
(a) M. H. Kim, Y. Kitamoto, S. Kantake, M. Abe, K. H. Kim and I. H. Son, J. Magn. Soc. Jpn., 1998, 22, 182–184 Search PubMed;
(b) D. Venkateshvaran, M. Althammer, A. Nielsen, S. Geprägs, M. S. R. Rao, S. T. B. Goennenwein, M. Opel and R. Gross, Phys. Rev. B, 2009, 79, 134405 CrossRef;
(c) R. Alrozi, N. A. Zubir, N. Amir, N. N. A. Abdul Rahman and M. A. Kamaruddin, J. Phys.: Conf. Ser., 2019, 1349, 012142 CrossRef CAS;
(d) X. Liang, Y. Zhong, S. Zhu, H. He, P. Yuan, J. Zhu and Zh. Jiang, Solid State Sci., 2013, 15, 115–122 CrossRef CAS;
(e) L. Menini, M. J. da Silva, M. F. F. Lelis, J. D. Fabris, R. M. Lago and E. V. Gusevskaya, Appl. Catal., A, 2004, 269, 117–121 CrossRef CAS.
- Sh. Yang, H. He, D. Wu, D. Chen, X. Liang, Z. Qin, M. Fan, J. Zhu and P. Yuan, Appl. Catal., B, 2009, 89, 527–535 CrossRef CAS.
-
(a) D. Azarifar, R. Asadpoor, O. Badalkhani, M. Jaymand, E. Tavakoli and M. Bazouleh, ChemistrySelect, 2018, 3, 13722–13728 CrossRef CAS;
(b) D. Azarifar and Y. Abbasi, Synth. Commun., 2016, 46, 745–758 CrossRef CAS;
(c) K. Nikoofar and F. Molaei Yielzoleh, J. Chin. Chem. Soc., 2021, 68, 1549–1562 CrossRef CAS.
- F. Walz, J. H. V. J. Brabers and V. A. M. Brabers, Z. Metallkd., 2002, 93, 1095–1102 CrossRef CAS.
- X. Pan, A. Sun, Y. Han, W. Zhang and X. Zhao, J. Mater. Sci.: Mater. Electron., 2019, 30, 4644–4657 CrossRef CAS.
- K. Praveena, B. Radhika and S. Srinath, Procedia Eng., 2014, 76, 1–7 CrossRef CAS.
-
(a) K. Y. Cai, Y. Sh. Liu, Y. Xu, H. Zhou, L. Zhang and Y. Cui, Bull. Chem. React. Eng. Catal., 2017, 12, 89–95 CrossRef CAS;
(b) A. Hasanpour, M. Gheisari, J. Amighian, M. Niyaifar and M. Darabi, J. Magn. Magn. Mater., 2013, 346, 38–43 CrossRef CAS;
(c) X. Wang, F. Xia, X. Li, X. Xu, H. Wang, N. Yang and J. Gao, J. Nanopart. Res., 2015, 17, 436–447 CrossRef.
-
(a) M. B. Gawande, Y. Monga, R. Zboril and R. K. Sharma, Coord. Chem. Rev., 2015, 288, 118–143 CrossRef CAS;
(b) Ch. Li, Ch. Ma, F. Wang, Zh. Xi, Zh. Wang, Y. Deng and N. He, J. Nanosci. Nanotechnol., 2012, 12, 2964–2972 CrossRef CAS PubMed.
-
(a) H. Husain, B. Hariyanto, M. Sulthonul, W. Klysubun, D. Darminto and S. Pratapa, Mater. Res. Express, 2019, 6, 086117 CrossRef;
(b) S. K. Panda, I. Aggarwal, H. Kumar, L. Prasad, A. Kumar, A. Sharma, D. V. N. Vo, D. V. Thuan and V. Mishra, Environ. Chem. Lett., 2021, 19, 2487–2525 CrossRef CAS;
(c) Y. Cai, W. Peng, S. Demeshko, J. Tian and Ph. Vana, Macromol. Rapid Commun., 2018, 39, 1800226 CrossRef PubMed;
(d) X. Pham, E. Hahm, H. Kim, B. S. Son, A. Jo, J. An, T. A. T. Thi, D. Q. Nguyen and B. Jun, Nanomaterials, 2020, 10, 117–130 CrossRef CAS PubMed;
(e) G. Asab, E. A. Zereffa and T. A. Seghne, Int. J. Biomater., 2020, 2020, 4783612 Search PubMed;
(f) Z. Gao, H. L. Ring, A. Sharma, B. Namsrai, N. Tran, E. B. Finger, M. Garwood, Ch. L. Haynes and J. O. C. Bischof, Adv. Sci., 2020, 7, 1901624 CrossRef CAS PubMed;
(g) L. Qiu, Sh. Zhou, Y. Li, W. Rui, P. Cui, Ch. Zhang, Y. Yu, Ch. Wang, X. Wang, J. Wang and P. Jiang, Technol. Cancer Res. Treat., 2021, 20, 1–9 Search PubMed.
-
(a) A. Mobinikhaledi, H. Moghanian and M. Ghanbari, Appl. Organomet. Chem., 2018, 32, e4108 CrossRef;
(b) R. Karimi-Chayjani, N. Daneshvar, M. Safarpoor Nikoo Langarudi, F. Shirini and H. Tajik, J. Mol. Struct., 2020, 1199, 126891 CrossRef CAS;
(c) R. Baharfar, S. Peiman and B. Maleki, J. Heterocycl. Chem., 2021, 58, 1302–1310 CrossRef CAS;
(d) Q. Han, H. Zhang, J. Sun, Zh. Liu, W. Huang, Ch. Xue and X. Mao, Catalysts, 2019, 9, 361–372 CrossRef CAS;
(e) S. Sonei, F. Taghavi, A. Khojastehnezhad and M. Gholizadeh, ChemistrySelect, 2021, 6, 359–368 CrossRef CAS.
-
(a) R. Narayan, Bio-based nanostructured materials, Nanobiomaterials nanostructured materials for biomedical applications, ed. M. Razavi, Woodhead Publishing, 1st edn, 2018, ch. 2, pp. 17–39 Search PubMed;
(b) E. Nazarzadeh Zare, P. Makvandi, A. Borzacchiello, F. R. Tay, B. Ashtari and V. V. T. Padil, Chem. Commun., 2019, 55, 14871–14885 RSC;
(c) J. T. Martins, Ó. L. Ramos, A. C. Pinheiro, A. I. Bourbon, H. D. Silva, M. C. Rivera, M. A. Cerqueira, L. Pastrana, F. X. Malcata, Á. González-Fernández and A. A. Vicente, Food Eng. Rev., 2015, 7, 491–513 CrossRef CAS;
(d) A. Al Rai and M. Yanilmaz, Cellulose, 2021, 28, 5169–5218 CrossRef CAS PubMed;
(e) Y. Li, X. Zheng and Q. Chu, Nano Today, 2021, 38, 101134 CrossRef CAS.
-
(a) K. Fukumoto, M. Yoshizawa and H. Ohno, J. Am. Chem. Soc., 2005, 127, 2398–2399 CrossRef CAS PubMed;
(b) G. Tao, L. He, W. Liu, L. Xu, W. Xiong, T. Wang and Y. Kou, Green Chem., 2006, 8, 639–646 RSC;
(c) S. Kirchhecker and D. Esposito, Curr. Opin. Green Sustain. Chem., 2016, 2, 28–33 CrossRef;
(d) P. Chakraborty and E. Gazit, ChemNanoMat, 2018, 4, 730–740 CrossRef CAS PubMed;
(e) X. Yan, P. Zhu and J. Li, Chem. Soc. Rev., 2010, 39, 1877–1890 RSC;
(f) X. Liu, Sh. Dong, L. Lin and X. Feng, Chin. J. Chem., 2018, 36, 791–797 CrossRef CAS.
- D. G. Hayes, D. K. Y. Solaiman and R. D. Ashby, Arginine-based surfactants: synthesis, aggregation properties, and applications, Biobased surfactants synthesis, properties, and applications, ed. A. Pinazo, L. Pérez, M. C. Morán and R. Pons, AOCS Press, 2019, ch. 13, pp. 413–445 Search PubMed.
- P. Sengupta and B. L. V. Prasad, ACS Omega, 2018, 3, 4242–4251 CrossRef CAS PubMed.
- D. Venturi, A. Chrysanthou, B. Dhuiège, K. Missoum and M. G. Baschetti, Nanomaterials, 2019, 9, 877–893 CrossRef CAS PubMed.
- A. Hsini, M. Benafqir, Y. Naciri, M. Laabd, A. Bouziani, M. Ez-zahery, R. Lakhmiri, N. El Alem and A. Albourine, Colloids Surf., A, 2021, 617, 126274 CrossRef CAS.
-
(a) H. C. Erythropel, J. B. Zimmerman, T. M. de Wintera, L. Petitjean, F. Melnikov, Ch. H. Lam, A. W. Lounsbury, K. E. Mellor, N. Z. Janković, Q. Tu, L. N. Pincus, M. M. Falinskia, W. Shi, Ph. Coish, D. L. Plata and P. T. Anastas, Green Chem., 2018, 20, 1929–1961 RSC;
(b) P. T. Anastas and J. B. Zimmerman, Green Chem., 2019, 21, 6545–6566 RSC.
-
(a) P. Cintas, Ultrason. Sonochem., 2016, 28, 257–258 CrossRef CAS PubMed;
(b) G. Chatel and R. S. Varma, Green Chem., 2019, 21, 6043–6050 RSC;
(c) K. J. Ardila-Fierro and J. G. Hernández, ChemSusChem, 2021, 14, 2145–2162 CrossRef CAS PubMed.
-
(a) H. Jahangirian, E. Ghasemian Lemraski, T. J. Webster, R. Rafiee-Moghaddam and Y. Abdollahi, Int. J. Nanomed., 2017, 12, 2957–2978 CrossRef CAS PubMed;
(b) H. Mousavi, Int. J. Biol. Macromol., 2021, 186, 1003–1166 CrossRef CAS PubMed;
(c) H. R. El-Seedi, R. M. El-Shabasy, S. A. M. Khalifa, A. Saeed, A. Shah, R. Shah, F. J. Iftikhar, M. M. Abdel-Daim, A. Omri, N. H. Hajrahand, J. S. M. Sabir, X. Zou, M. F. Halabi, W. Sarhann and W. Guo, RSC Adv., 2019, 9, 24539–24559 RSC.
-
(a) S. Zangade and P. Patil, Curr. Org. Chem., 2019, 23, 2295–2318 CrossRef CAS;
(b) M. Tavakolian, S. Vahdati-Khajeh and S. Asgari, ChemCatChem, 2019, 11, 2943–2977 CrossRef CAS;
(c) A. Dhakshinamoorthy, A. M. Asiri, M. Alvaro and H. Garcia, Green Chem., 2018, 20, 86–107 RSC.
-
(a) K. Nikoofar, Z. Arian and H. Djahaniani, Inorg. Nano-Met. Chem., 2022, 52, 365–374 CAS;
(b) K. Nikoofar and N. Saheb Ekhtiari, Mol. Diversity, 2021 DOI:10.1007/s11030-021-10268-6;
(c) F. Molaei Yielzoleh and K. Nikoofar, Polycyclic Aromat. Compd., 2021 DOI:10.1080/10406638.2021.1878248;
(d) F. Molaei Yielzoleh and K. Nikoofar, Appl. Organomet. Chem., 2021, 35, e6043 CrossRef CAS;
(e) K. Nikoofar and S. S. Peyrovebaghi, J. Chin. Chem. Soc., 2020, 67, 1303–1313 CrossRef CAS.
-
(a) W. Wei, W. Sun, H. Hu, Zh. Jiang, L. Ma and J. Xie, J. Mater. Sci., 2018, 53, 13886–13899 CrossRef CAS;
(b) K. K. Bera, R. Majumdar, M. Chakraborty and S. K. Bhattacharya, J. Hazard. Mater., 2018, 352, 182–191 CrossRef CAS PubMed.
- K. Scherer, L. Nouvelot, P. Lacan and R. Bosmans, Appl. Opt., 1996, 35, 5067–5072 CrossRef CAS PubMed.
- P. Li, E. Y. Jiang and H. L. Bai, J. Phys. D: Appl. Phys., 2011, 44, 075003 CrossRef.
- S. Lawson, B. J. Tielsch and J. E. Fulghum, Surf. Sci. Spectra, 1996, 4, 316–344 CrossRef.
- X. Zhang, Y. Liu, G. Zhang, Y. Wang, H. Zhang and F. Huang, ACS Appl. Mater. Interfaces, 2015, 7, 4442–4448 CrossRef CAS PubMed.
- J. Zhanga, Y. Lu, L. Gea, Ch. Han, Y. Li, Y. Gao, S. Li and H. Xu, Appl. Catal., B, 2017, 204, 385–393 CrossRef.
- H. Zhao, Y. Qiao, X. Du, S. Wang, Q. Zhang, Y. Zang and Z. Cai, Appl. Sci., 2019, 9, 5500–5514 CrossRef CAS.
- S. Kabir, K. Artyushkova, A. Serov, B. Kiefer and P. Atanassova, Surf. Interface Anal., 2016, 48, 293–300 CrossRef CAS.
- S. Ravia, S. Zhanga, Y. Leea, K. Kangb, J. Kimc, J. Ahnd and W. Ahna, J. Ind. Eng. Chem., 2018, 67, 210–218 CrossRef.
- K. Li, Y. Zhao, P. Zhang, Ch. He, J. Deng, Sh. Ding and W. Shi, Appl. Surf. Sci., 2016, 390, 412–421 CrossRef CAS.
-
(a) Zh. Wang, J. Xu, H. Zhou and X. Zhang, Rare Met., 2019, 38, 459–467 CrossRef CAS;
(b) J. Hao, X. Wang, F. Liu, Sh. Han, J. Lian, and Q. Jiang, Sci. Rep., 2017, 7, 3021 Search PubMed.
-
(a) X. Yan, T. Xu, G. Chen, Sh. Yang, H. Liu and Q. Xue, J. Phys. D: Appl. Phys., 2004, 37, 907–913 CrossRef CAS;
(b) A. Dager, T. Uchida, T. Maekawa and M. Tachibana, Sci. Rep., 2019, 9, 14004 CrossRef PubMed;
(c) S. Jerng, D. S. Yu, J. H. Lee, Ch. Kim, S. Yoon and S. Chun, Nanoscale Res. Lett., 2011, 6, 565 CrossRef PubMed.
- A. Rahmati, S. Ahmadi and M. Ahmadi-Varzaneh, Tetrahedron, 2014, 7, 9512–9521 CrossRef.
- X. L. Liang, Y. H. Zhong, H. P. He, P. Yuan, J. X. Zhu, S. Y. Zhu and Z. Jiang, Chem. Eng. J., 2012, 191, 177–184 CrossRef CAS.
Footnote |
† Electronic supplementary information (ESI) available. See DOI: 10.1039/d2ra00212d |
|
This journal is © The Royal Society of Chemistry 2022 |
Click here to see how this site uses Cookies. View our privacy policy here.