DOI:
10.1039/D2RA01913B
(Paper)
RSC Adv., 2022,
12, 13820-13825
Electron transport layer assisted by nickel chloride hexahydrate for open-circuit voltage improvement in MAPbI3 perovskite solar cells†
Received
24th March 2022
, Accepted 3rd May 2022
First published on 9th May 2022
Abstract
SnO2 is a promising electron transport layer (ETL) material with important applications in planar perovskite solar cells (PSCs). However, electron–hole recombination and charge extraction between SnO2 and the perovskite layer necessitates further exploration. Nickel chloride hexahydrate (NiCl2·6H2O) was introduced into the SnO2 ETL, which significantly increased the power conversion efficiency (PCE) from 15.49 to 17.36% and the open-circuit voltage (VOC) from 1.078 to 1.104 V. The improved PCE and VOC were attributed to the reduced defect states and increased energy level of the conduction band minimum. This work provides new insights into optimizing the VOC and PCE of PSCs.
Introduction
In recent years, the perovskite solar cell (PSC) has been developed as a promising type of solar cell with outstanding advantages such as high photoelectric conversion efficiency, low cost, and simple fabrication.1–3 At present, PSCs have achieved a high power conversion efficiency (PCE) of 25.5%.4–12 The main technical approach used to improve the PCE of PSCs is through component engineering of the perovskite layer, hole transport layer (HTL), and electron transport layer (ETL).13–18 The other method is to optimize the interface performance by adjusting the structure or adding a buffer layer between the interlayers of PSCs.19–28
The quality of the ETL plays an important role in perovskite device performance. TiO2, one of the most widely used ETL materials, has deep electron trap states, which easily leads to strong electron–hole recombination. ZnO has also been used as an ETL in PSCs due to its low-temperature film preparation and higher charge mobility. However, the PSC readily decomposes owing to the polyhydroxy groups on the surface of the ZnO. The SnO2 ETL material is widely used in PSCs for high charge mobility and high perovskite compatibility.29 However, the oxygen vacancy in SnO2 greatly reduces the hole blocking effect, resulting in significant carrier recombination.30 Therefore, the SnO2 ETL requires modification to reduce ETL defects and improve the performance of PSCs. For example, Liu et al. incorporated EDTA into the SnO2 ETL to enhance the device performance,31 whereas Yang et al. doped Ga3+ ions into SnO2 to passivate the defect state in the ETL.32
Herein, we report a simple and effective method to passivate SnO2 defects by introducing nickel chloride hexahydrate (NiCl2·6H2O) into a SnO2 precursor solution. NiCl2·6H2O not only reduced the trap density, but also increased the energy level of the conduction band minimum of SnO2 and caused the energy level to better match that of the perovskite. Consequently, a champion PCE of 17.36% with a VOC of 1.104 V was achieved, remarkably higher than the relevant reference devices which provided a PCE of 15.49% and VOC of 1.078 V.
Experimental section
Materials
All the materials and solvents were used as received without further purification. Fluorine-doped tin oxide (FTO) glasses (1.5 cm × 1.5 cm) were purchased from OPVtech (China). The SnO2 solution (tin(IV) oxide, 15 wt% hydrocolloid dispersion) was purchased from Alfa Aesar. Lead iodide (PbI2), methylammonium iodide (MAI), and 2,2′,7,7′-tetrakis (N,N-di-p-methoxyphenylamine)-9,9′-spirobifluorene (spiro-OMeTAD) were obtained from Xi'an Polymer Light Technology. N,N-dimethylformamide (DMF), dimethyl sulfoxide (DMSO), chlorobenzene (CB), and 4-tert-butylpyridine were acquired from J&K. Lithium bis(trifluoromethylsulfonyl) imide (Li-TFSI) and cobalt(III) FK209 were obtained from Sigma-Aldrich. Nickel dichloride hexahydrate (NiCl2·6H2O) and ammonium (NH3·H2O) were purchased from Aladdin.
Device fabrication
The FTO glass was sequentially cleaned with detergent water, deionized water, acetone, and isopropanol for 30 min in an ultrasonic system. Plasma treatment was performed for 3 min to enhance the surface wettability before spin coating the ETL solution. The pristine SnO2 precursor solution was prepared from the SnO2 colloidal solution by diluting 5 times with deionized water. The sample ETL solution was prepared by adding 4, 12 and 20 mg NiCl2·6H2O into the 4 mL mixed solvent, which comprise of 1 mL SnO2 colloidal solution, 2 mL H2O and 1 mL NH3·H2O. Ultimately the concentration of the NiCl2·6H2O was 1, 3 and 5 mg mL−1. The sample ETL (SnO2–NiCl2) solution was deposited on the FTO substrates at 4000 rpm for 30 s. The substrates were subsequently annealed in the air at 150 °C for 30 min to improve the electrical properties. After plasma treatment, the FTO substrates were transferred into a glove box. A perovskite precursor solution was prepared from the solution mixture of PbI2 (1.67 M) and CH3NH3I (1.67 M) in DMF/DMSO (7
:
3 by volume). The perovskite solution was spin coated on the substrate at 1000 rpm for 13 s and at 5000 rpm for 25 s. Three hundred microliters of CB was dropped on the substrates during the second step after 12–15 s, and the films were then annealed on the hot plate at 100 °C for 30 min. After the substrates cooled to room temperature, a HTL was formed by spin coating the spiro-OMeTAD precursor solution onto the surface of the perovskite film at 5000 rpm for 30 s, which was prepared by dissolving 58.4 mg of spiro-OMeTAD, 23 μL of tBp, 14 μL of LiTFSI (520 mg mL−1 acetonitrile), and 23 μL of FK209 (300 mg mL−1 acetonitrile) in 800 μL of chlorobenzene. Finally, an 80 nm gold electrode was deposited on the surface of the HTL under high-vacuum conditions.
Measurements and characterization
Scanning electron microscopy (SEM) images were obtained by a field emission scanning electron microscope (FE-SEM, Hitachi SU8010). X-ray diffraction (XRD) was conducted on a Shimadzu XRD7000 diffractometer using Cu Kα radiation in the 2θ range from 10° to 60° at a scan rate of 2° min−1. X-ray photoelectron spectroscopy (XPS) was performed using a Thermo KAlpha + instrument with an Al Kα (1486.8 eV) X-ray source. UV-vis absorption spectra were recorded on an Agilent Cary 60 spectrometer. Steady-state photoluminescence (PL) and time-resolved PL (TRPL) spectroscopies were performed by an Edinburgh FLS 980 spectrometer. The current density–voltage (J–V) curves were measured by a Keithley 2400 source meter under simulated standardized sunlight (AM 1.5 G, 100 mW cm−2) in ambient air. The devices were covered by a metal aperture mask to determine the 0.1 cm2 active area. The incident photon-to-current efficiency (IPCE) was characterized by the photoelectric conversion test system (Enlitech QE-R). Electrochemical impedance spectroscopy (EIS) was performed using ZAHNER PP211 with a frequency range from 0.1 to 106 Hz. The space charge limited current (SCLC) of the devices with FTO/SnO2/perovskite/spiro/Au was measured on a Keithley 4200 from 0 to 2 V in the dark. In Transient photovoltage (TPV) measurements, the devices were illuminated by a light-emitting diode (LED, 520 ± 5 nm) to generate a different bias photovoltage (Vph). A weak laser pulse (532 nm, 7 ns) was applied to generate a small perturbation. A series of desired Vph values were collected by adjusting the intensity of the LED. The results were recorded using a digital oscilloscope (Lecroy HDO4054A, coupling impedance: 1 MΩ). Transient photocurrent (TPC) results were recorded using the digital oscilloscope (input impedance of 50 Ω) after the TPV measurements.
Results and discussion
As shown in Fig. S1,† the introduction of NiCl2·6H2O did not change the crystal structure of SnO2. The higher transmittance of SnO2–NiCl2 film in the visible region indicates that NiCl2·6H2O could reduce the light loss when light passing through the substrate. FTO/SnO2 films became smoother when the FTO substrate covered with a thin SnO2 film. The smoother surface should be beneficial to the light transmission. SnO2 films can improve the optical transmission properties of FTO substrates.33,34 Compared with FTO/SnO2, FTO/SnO2–NiCl2 films were denser, and Ni2+ had the effect of antireflection, which promoted the increase of transmittance of FTO/SnO2–NiCl2 films in the visible region.35,36 Fig. 1a and b show the SEM images of the SnO2 films without and with NiCl2, respectively. The introduction of NiCl2·6H2O improved surface compactness of the ETL, which can promote the formation of perovskite grains and performance of the device.37
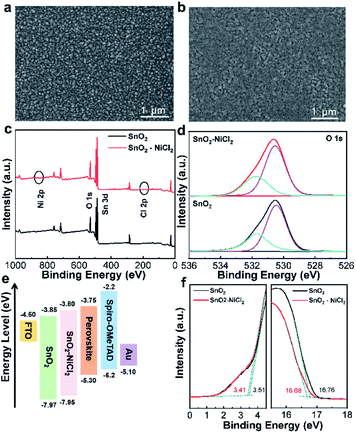 |
| Fig. 1 ETLs using (a) pristine SnO2 and (b) SnO2–NiCl2. (c) The XPS spectrum of SnO2 and SnO2–NiCl2 on FTO substrates. (d) XPS of O 1s. (e) Energy level diagram of PSCs. (f) UPS spectra of SnO2 and SnO2–NiCl2 films. | |
To confirm the successful introduction of nickel and chloride ions into the ETL, XPS was performed on different substrates. Fig. 1c shows the full XPS spectra of the corresponding ETLs. Fig. S4† shows energy dispersive spectroscopy (EDS) characterization of SnO2–NiCl2 films. The results show that the Ni, Cl, Sn, and O elements are uniformly distributed on the surface of the film. The enlarged XPS spectra are shown in Fig. S2 and S3†.38 The XPS spectra of SnO2–NiCl2 show characteristic peaks of Ni (855 eV) and Cl (198 eV) compared with unmodified SnO2. SnO2 was deposited on FTO substrate, and it was annealed in the air at 150 °C for 30 min. In the annealing process, [Ni(NH3)6]2+ by reaction with Ni2+ and NH3·H2O would almost loses the NH3 and H2O when heated above 140 °C. The presence of Ni 2p3/2 at 855.6 eV, based on the binding energy position of XPS Ni 2p, indicates the transformation of Ni2+ into Ni(OH)2. Ni(OH)2 cannot further lose water to form NiO during annealing at 150 °C. The photoelectron binding energy of the Sn 3d level of SnO2–NiCl2 is slightly higher than that of the unmodified SnO2, indicating an interaction between SnO2 and NiCl2. According to previous reports, the Ni2+ may play a more important role than Cl− in improving the device performance of PSCs.39 We presumed that the Ni2+ from NiCl2 can interact with the hydroxyl groups (-OH) on the surface of the SnO2 film, which can lead to a trap state, causing nonradiative recombination.40 As shown in Fig. 1d and Table S1,† the peak at 530.46 eV was O–Sn bonds (or O2− state), and the higher binding energy at 531.70 eV corresponded to hydroxyl groups (or oxygen vacancies). After the introduction of NiCl2·6H2O, the intensity of hydroxyl (or oxygen vacancies) decreased significantly, indicating that the oxygen vacancies were effectively passivated.41 The reduction of oxygen vacancy defects will improve VOC. Therefore, the successful introduction of NiCl2·6H2O will considerably improve the ETL.
Further characterizations were conducted to better understand the ETL/perovskite interface. Ultraviolet photoelectron spectroscopy (UPS) was performed to investigate the band structure of SnO2 and SnO2–NiCl2. We tested the optimal concentration 3 mg mL−1. The possible energy band alignment of planar PSCs was depicted in Fig. 1e. Combining with the high binding energy cutoff (Ecutoff) on the right side of Fig. 1f and the Fermi edge (EF,edge) region on the left side of Fig. 1f, the value of EVB could be calculated for SnO2 and SnO2–NiCl2 at about −7.97 and −7.95 eV, respectively. We further confirmed the band gaps (Eg) of ETLs by the Tauc plots of UV-vis absorption spectra (Fig. S5†). The energy levels of the conduction band (ECB) of SnO2 and SnO2–NiCl2 were calculated to be −3.85 and −3.80 eV, respectively. According to the relative energy levels of the different PSC parts, the ECB of the SnO2–NiCl2 ETL was higher than that of the SnO2 ETL.42 The VOC depends on the difference in energy between electron quasi Fermi level and hole quasi Fermi level.43 More appropriate energy level would make the solar cell based on SnO2–NiCl2 show higher VOC.
SEM characterization of the perovskite films prepared on SnO2 and SnO2–NiCl2 substrates was performed, and the results are shown in Fig. 2a and b. The perovskite films prepared on the SnO2–NiCl2 substrates have larger grains and are generally compact, compared with the perovskite films prepared on SnO2. In addition, cracks obviously exist on the perovskite films prepared on SnO2, which lead to the recombination of electrons and holes.44 The UV-vis absorption spectrum shown in Fig. 2c indicates that the bandgap of perovskite growing on the NiCl2-modified ETL has not changed. Fig. S6† presents the bandgap of perovskite grown on ETL with and without NiCl2·6H2O, and the calculated bandgap value is 1.61 eV. XRD analyses were performed to characterize the crystal structure of perovskite, as shown in Fig. 2d. The results show that the characteristic diffraction peaks of CH3NH3PbI3 appear near 14.1°, 24.5°, 28.5°, 31.6°, and 40.4°, which correspond to the crystal planes of (110), (202), (220), (310), and (224) of CH3NH3PbI3, respectively.45,46 These results confirm that perovskite films with good crystallinity were prepared by introducing NiCl2·6H2O.
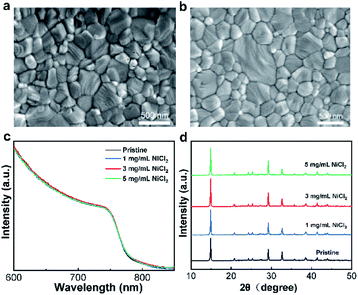 |
| Fig. 2 Top view SEM images of perovskite films (a) with pristine SnO2 and (b) SnO2–NiCl2. (c) The absorbance of perovskite films based on SnO2 and SnO2–NiCl2. (d) The XRD pattern of perovskite films with different concentrations of SnO2–NiCl2. | |
To study the carrier transport in PSCs, steady-state fluorescence spectroscopy (PL) experiments were performed on the perovskite films on SnO2 and SnO2–NiCl2 substrates.47,48 As shown in Fig. 3a, carrier quenching was slower for the samples on the SnO2–NiCl2 substrates, which is detrimental to increasing JSC of the solar cell. However, the transmittance of the tin dioxide layer was increased after modification by NiCl2·6H2O, the utilization rate of photons will be improved and JSC will increase. Overall, the JSC of the device based on SnO2–NiCl2 was a little better than that of the SnO2 solar cell. The reason of the carrier quenching was slow for the samples on he SnO2–NiCl2 substrates was the passivation of chloride ions, which suppressed the nonradiative recombination of perovskite films for the enhancing intensity and the larger perovskite grain size.40 In Fig. 3b, the biexponential decay behavior is evident on the TRPL spectrum. We calculated the decay times (e.g., τ1 and τ2) of the perovskite film deposited on the SnO2 and SnO2–NiCl2 substrates; the fitting parameters are summarized in Table S2.† PL decay process consists of a carrier recombination process in the bulk region, as characterized by the slow decay lifetime τ2, as well as a charge-transfer process at the interface, as characterized by the fast lifetime τ1. According to the results of the double exponential fitting in TRPL τ1 increases, show that charge extraction at a slower pace and it will cause a decline in JSC. τ2 increases, and combining with SCLC and EIS data, shows that tin oxide layer of oxygen vacancy defects passivation, VOC increases. The average decay time of the SnO2/perovskite film is 70.62 ns, and the perovskite film deposited on SnO2–NiCl2 exhibited slower fluorescence quenching with an average decay time of 107.1 ns. The results were consistent with PL conclusions.
 |
| Fig. 3 (a) The PL and (b) TRPL spectra of MAPbI3 without and with NiCl2. | |
To justify the photoelectric performance of the PSCs caused by the introduction of NiCl2·6H2O, SCLC analyses were performed on the SnO2 and SnO2–NiCl2 ETLs. The results are shown in Fig. 4a and b, including the calculated density of defect states. The defect density between the interface of ETL and the perovskite layer can partially reflect the contact and matching degree between interfaces. In the SCLC analyses, there is a clear linear relationship between the current and low bias voltage, which becomes non-linear as the voltage increases. The bias voltage of different substrates can be obtained from the SCLC curve, and the defect density can be subsequently calculated according to the formula of Nt = 2εε0VTFL/eL2, where VTFL is the trap-filled-limit voltage marked in Fig. 4a and b, e is the elementary charge, L is the thickness of the perovskite film, ε is the dielectric constant, and ε0 is the vacuum permittivity. According to the single calculation of the SCLC results, the bias voltage of the SnO2–NiCl2 substrate decreased from 0.930 V to 0.810 V, indicating a tendency of the defect state between the ETL and the perovskite layer to decrease after NiCl2 modification. After further calculation obtained defect concrete values, the trap densities of perovskite film deposited on pristine SnO2 and SnO2–NiCl2 ETL are 1.45 × 1016 cm−3 and 1.26 × 1016 cm−3.
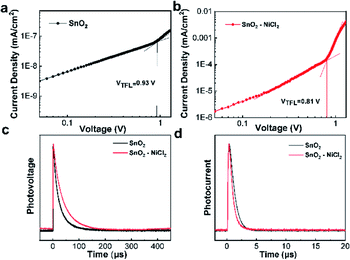 |
| Fig. 4 SCLC measurements of devices (a) with pristine SnO2 and (b) SnO2–NiCl2. (c) Transient photovoltage decay curves of PSCs based on pristine SnO2 and SnO2–NiCl2. (d) Transient photocurrent decay curves of PSCs based on pristine SnO2 and SnO2–NiCl2. | |
We further elucidated the effect of NiCl2 on the defects of the SnO2 film by measuring the recombination dynamics, transient photovoltage (TPV), and transient photocurrent (TPC) decay. As shown in Fig. 4c, the carrier recombination lifetime of the device with SnO2–NiCl2 increased from 22.65 to 35.33 μs, confirming the suppressed charge recombination at the ETL and perovskite interface. For the transport time constants (τt) measured from TPC (Fig. 4d), PSCs based on SnO2–NiCl2 exhibited 0.67 μs compared to that of the pristine SnO2-based PSCs (0.93 μs).49,50
To investigate the effect of SnO2–NiCl2 ETL on device performance, we fabricated PSCs with the FTO/ETL/MAPbI3/Spiro-OMeTAD/Au (Fig. 5a) structure and investigated the doping concentration of NiCl2·6H2O in SnO2. The devices were prepared with the concentration of SnO2 precursor solution (1, 3 and 5 mg mL−1) and annealing at 150 °C for 30 min. Electrochemical impedance spectroscopy (EIS) analyses were performed on devices composed of SnO2 and SnO2–NiCl2 substrates,51–53 and the results are shown in Fig. 5b. In the dark state condition, within the frequency range of 0.1 to 106 Hz, the transmission electricity of the SnO2-based cell was measured. By fitting the measured results, the contact resistance (Rs) and recombination resistance (Rrec) values of the PSCs employing pristine SnO2 and SnO2–NiCl2 are summarized in Table S3.† The small semicircle at high frequency corresponds to the charge transfer process between perovskite and selective contacts while the large semicircle at low frequency demonstrates the recombination of charge carriers in the perovskite layer.54 Through EIS test, the results show that the transmission resistance Rs and the composite resistance Rrec increased, indicating that the charge transmission speed slowed down and the defect was effectively passivated. However, because the transmittance of the tin dioxide layer increased after modification, the utilization rate of photons will be improved and JSC will increase. The results were consistent with the fluorescence and efficiency data.52 Simultaneously, it was beneficial to improve VOC of the device.53
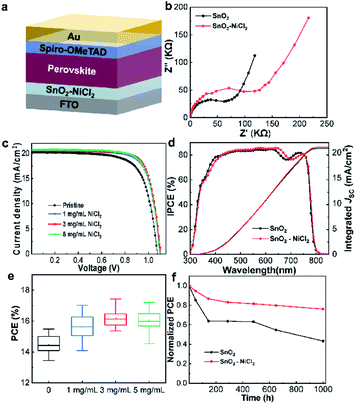 |
| Fig. 5 (a) Device architecture. (b) Nyquist plots. (c) J–V characteristics of the best devices with different nickel chloride concentrations. (d) External quantum efficiency of PSCs based on pristine SnO2 and SnO2–NiCl2. (e) Device performance of PSCs based on SnO2 with different concentrations of NiCl2. (f) Stability measurements of PSCs based on pristine SnO2 and SnO2–NiCl2 under a N2 atmosphere. | |
The corresponding J–V curves are shown in Fig. 5c and S7.† The results show that the device performance slightly improved with SnO2–NiCl2 concentration of 1 mg mL−1. The highest open-circuit voltage (VOC), FF, and PCE values of the PSCs were obtained with SnO2–NiCl2 concentration of 3 mg mL−1. When the doping NiCl2·6H2O amount further increased, the photoelectric performance of the device decreased, and thus the optimum concentration of SnO2–NiCl2 was 3 mg mL−1.
Subsequently, the IPCE of the optimum NiCl2·6H2O and control devices were tested. As shown in Fig. 5d, the light absorption capacity of the NiCl2-modified devices in the range of 400–700 nm was stronger than that of the SnO2-based devices, corresponding to a higher current. The results show that the introduction of NiCl2·6H2O could effectively improve the ability of the ETL to light transmission. The integrated JSC value measured by IPCE was consistent with the JSC obtained by the J–V curves.
The statistical distribution of PCE for PSCs based on doped ETLs of different NiCl2·6H2O concentrations are shown in Fig. 5e and S8.† The PCE of the SnO2-based devices ranged between 14%-16% and the PCE of the SnO2–NiCl2-based devices was in the range of 16–18%. As shown in Table 1, the modified device exhibits a PCE of 17.36% with a JSC of 20.59 mA cm−2, VOC of 1.104 V, and FF of 76.4%. Devices based on the SnO2–NiCl2 ETL also exhibit greater stability than the reference devices (Fig. 5f). The SnO2–NiCl2-based PSCs can retain 76% of their initial efficiency after being stored for 1000 h at the room temperature (RT) of 25 °C for 1000 h. In contrast, the reference devices only retained 43% of their initial efficiency. This may be due to the larger grain size with fewer grain boundaries which offers additional protection against oxygen and moisture.
Table 1 Photovoltaic parameters of cells based on SnO2 and SnO2–NiCl2 films with different additive concentrations
Samples |
VOC (V) |
JSC (mA cm−2) |
FF (%) |
PCE (%) |
Pristine |
1.078 |
20.21 |
0.711 |
15.49 |
1 mg mL−1 NiCl2 |
1.098 |
20.70 |
0.749 |
17.02 |
3 mg mL−1 NiCl2 |
1.104 |
20.59 |
0.764 |
17.36 |
5 mg mL−1 NiCl2 |
1.100 |
20.70 |
0.756 |
17.21 |
Conclusions
In this study, we provide a simple and effective method to modify SnO2 ETL with NiCl2·6H2O, which reduced the oxygen vacancies of SnO2 and improved the energy level alignment. This modification ultimately dramatically passivated defects, which effectively improved the VOC and PCE. Notably, the stability of the SnO2–NiCl2-based PSCs increased, and was able to maintain 76% of the original PCE after 1000 h of storage, compared to 43% for the device without NiCl2·6H2O.
Conflicts of interest
There are no conflicts to declare.
Acknowledgements
The authors acknowledge financial support from the National Key R&D Program of China (Grant no. 2018YFA0208701), National Natural Science Foundation of China (Grant no. 21773308), the Research Funds of Renmin University of China (Grant no. 201903020).
Notes and references
- Q. Chen, N. De Marco, Y. Yang, T.-B. Song, C.-C. Chen, H. Zhao, Z. Hong, H. Zhou and Y. Yang, Nano Today, 2015, 10, 355–396 CrossRef CAS.
- M. A. Green, A. Ho-Baillie and H. J. Snaith, Nat. Photonics, 2014, 8, 506–514 CrossRef CAS.
- G. Xing, N. Mathews, S. Sun, S. S. Lim, Y. M. Lam, M. Gratzel, S. Mhaisalkar and T. C. Sum, Science, 2013, 342, 344–347 CrossRef CAS PubMed.
- H. Min, M. Kim, S. U. Lee, H. Kim, G. Kim, K. Choi, J. H. Lee and S. I. Seok, Science, 2019, 366, 749–753 CrossRef CAS PubMed.
- M. Abdi-Jalebi, Z. Andaji-Garmaroudi, S. Cacovich, C. Stavrakas, B. Philippe, J. M. Richter, M. Alsari, E. P. Booker, E. M. Hutter, A. J. Pearson, S. Lilliu, T. J. Savenije, H. Rensmo, G. Divitini, C. Ducati, R. H. Friend and S. D. Stranks, Nature, 2018, 555, 497–501 CrossRef CAS PubMed.
- W. S. Yang, B. W. Park, E. H. Jung, N. J. Jeon, Y. C. Kim, D. U. Lee, S. S. Shin, J. Seo, E. K. Kim, J. H. Noh and S. I. Seok, Science, 2017, 356, 1376–1379 CrossRef CAS PubMed.
- W. S. Yang, J. H. Noh, N. J. Jeon, Y. C. Kim, S. Ryu, J. Seo and S. I. Seok, Science, 2015, 348, 1234–1237 CrossRef CAS PubMed.
- K. Xiao, R. Lin, Q. Han, Y. Hou, Z. Qin, H. T. Nguyen, J. Wen, M. Wei, V. Yeddu, M. I. Saidaminov, Y. Gao, X. Luo, Y. Wang, H. Gao, C. Zhang, J. Xu, J. Zhu, E. H. Sargent and H. Tan, Nat. Energy, 2020, 5, 870–880 CrossRef CAS.
- M. Jeong, I. W. Choi, E. M. Go, Y. Cho, M. Kim, B. Lee, S. Jeong, Y. Jo, H. W. Choi, J. Lee, J. H. Bae, S. K. Kwak, D. S. Kim and C. Yang, Science, 2020, 369, 1615–1620 CrossRef CAS PubMed.
- R. Lin, K. Xiao, Z. Qin, Q. Han, C. Zhang, M. Wei, M. I. Saidaminov, Y. Gao, J. Xu, M. Xiao, A. Li, J. Zhu, E. H. Sargent and H. Tan, Nat. Energy, 2019, 4, 864–873 CrossRef CAS.
- M. Saliba, T. Matsui, J. Y. Seo, K. Domanski, J. P. Correa-Baena, M. K. Nazeeruddin, S. M. Zakeeruddin, W. Tress, A. Abate, A. Hagfeldt and M. Gratzel, Energy Environ. Sci., 2016, 9, 1989–1997 RSC.
- H. Min, D. Y. Lee, J. Kim, G. Kim, K. S. Lee, J. Kim, M. J. Paik, Y. K. Kim, K. S. Kim, M. G. Kim, T. J. Shin and S. Il Seok, Nature, 2021, 598, 444–450 CrossRef CAS PubMed.
- W. Xu, X. Yao, H. Wu, T. Zhu and X. Gong, Emergent Mater., 2020, 3, 727–750 CrossRef CAS.
- X. Zhao, L. Tao, H. Li, W. Huang, P. Sun, J. Liu, S. Liu, Q. Sun, Z. Cui, L. Sun, Y. Shen, Y. Yang and M. Wang, Nano Lett., 2018, 18, 2442–2449 CrossRef CAS PubMed.
- Y. Wu, X. Yang, W. Chen, Y. Yue, M. Cai, F. Xie, E. Bi, A. Islam and L. Han, Nat. Energy, 2016, 1, 16148 CrossRef CAS.
- Z. Zhu, C. C. Chueh, F. Lin and A. K. Jen, Adv. Sci. (Weinh.), 2016, 3, 1600027 Search PubMed.
- G. Grancini, C. Roldan-Carmona, I. Zimmermann, E. Mosconi, X. Lee, D. Martineau, S. Narbey, F. Oswald, F. De Angelis, M. Graetzel and M. K. Nazeeruddin, Nat. Commun., 2017, 8, 15684 CrossRef CAS PubMed.
- P. W. Liang, C. Y. Liao, C. C. Chueh, F. Zuo, S. T. Williams, X. K. Xin, J. Lin and A. K. Jen, Adv. Mater., 2014, 26, 3748–3754 CrossRef CAS PubMed.
- C. Zuo and L. Ding, Nanoscale, 2014, 6, 9935–9938 RSC.
- W. Ke, C. Xiao, C. Wang, B. Saparov, H. S. Duan, D. Zhao, Z. Xiao, P. Schulz, S. P. Harvey, W. Liao, W. Meng, Y. Yu, A. J. Cimaroli, C. S. Jiang, K. Zhu, M. Al-Jassim, G. Fang, D. B. Mitzi and Y. Yan, Adv. Mater., 2016, 28, 5214–5221 CrossRef CAS PubMed.
- Y. Wu, F. Xie, H. Chen, X. Yang, H. Su, M. Cai, Z. Zhou, T. Noda and L. Han, Adv. Mater., 2017, 29, 1701073 CrossRef PubMed.
- F. Wang, W. Geng, Y. Zhou, H. H. Fang, C. J. Tong, M. A. Loi, L. M. Liu and N. Zhao, Adv. Mater., 2016, 28, 9986–9992 CrossRef CAS PubMed.
- H. S. Kim, C. R. Lee, J. H. Im, K. B. Lee, T. Moehl, A. Marchioro, S. J. Moon, R. Humphry-Baker, J. H. Yum, J. E. Moser, M. Gratzel and N. G. Park, Sci. Rep., 2012, 2, 591 CrossRef PubMed.
- M. M. Lee, J. Teuscher, T. Miyasaka, T. N. Murakami and H. J. Snaith, Science, 2012, 338, 643–647 CrossRef CAS PubMed.
- R. Meng, X. Feng, Y. Yang, X. Lv, J. Cao and Y. Tang, ACS Appl. Mater. Interfaces, 2019, 11, 13273–13278 CrossRef CAS PubMed.
- A. K. Kadhim, M. R. Mohammad and A. I. Abd Ali, Chem. Phys. Lett., 2022, 786, 139189 CrossRef CAS.
- G. Liu, Z. Liu, L. Wang, K. Zhang and X. Xie, Chem. Phys. Lett., 2021, 771, 138496 CrossRef CAS.
- F. Wang, M. Yang, Y. Zhang, J. Du, S. Yang, L. Yang, L. Fan, Y. Sui, Y. Sun and J. Yang, Nano Res., 2021, 14, 2783–2789 CrossRef CAS.
- Q. Zhuang, H. Wang, C. Zhang, C. Gong, H. Li, J. Chen and Z. Zang, Nano Res., 2022 DOI:10.1007/s12274-022-4135-7.
- S. Wendt, P. T. Sprunger, E. Lira, G. K. Madsen, Z. Li, J. O. Hansen, J. Matthiesen, A. Blekinge-Rasmussen, E. Laegsgaard, B. Hammer and F. Besenbacher, Science, 2008, 320, 1755–1759 CrossRef CAS PubMed.
- D. Yang, R. Yang, K. Wang, C. Wu, X. Zhu, J. Feng, X. Ren, G. Fang, S. Priya and S. F. Liu, Nat. Commun., 2018, 9, 3239 CrossRef PubMed.
- T. Yang, X. Qin, H.-h. Wang, Q. Jia, R. Yu, B. Wang, J. Wang, K. Ibrahim, X. Jiang and Q. He, Thin Solid Films, 2010, 518, 5542–5545 CrossRef CAS.
- W. Ke, G. Fang, Q. Liu, L. Xiong, P. Qin, H. Tao, J. Wang, H. Lei, B. Li, J. Wan, G. Yang and Y. Yan, J. Am. Chem. Soc., 2015, 137, 6730–6733 CrossRef CAS PubMed.
- Y. Luan, X. Yi, P. Mao, Y. Wei, J. Zhuang, N. Chen, T. Lin, C. Li and J. Wang, iScience, 2019, 16, 433–441 CrossRef CAS PubMed.
- Y. Chen, X. Zuo, Y. He, F. Qian, S. Zuo, Y. Zhang, L. Liang, Z. Chen, K. Zhao, Z. Liu, J. Gou and S. F. Liu, Adv. Sci. (Weinh.), 2021, 8, 2001466 CAS.
- Z. Qian, L. Chen, J. Wang, L. Wang, Y. Xia, X. Ran, P. Li, Q. Zhong, L. Song, P. Müller-Buschbaum, Y. Chen and H. Zhang, Adv. Mater. Interfaces, 2021, 8, 2100128 CrossRef CAS.
- B. Y. Gao, Q. Cao, X. Y. Pu, J. B. Yang, J. Han, S. J. Wang, T. T. Li, Z. W. He and X. H. Li, Appl. Surf. Sci., 2021, 546, 148711 CrossRef CAS.
- L. Zhu, Q. Lu, C. Li, Y. Wang and Z. Deng, Chin. Chem. Lett., 2021, 32, 2259–2262 CrossRef CAS.
- D. Wang, C. Wu, X. Qi, W. Luo, Y. Zhang, Z. Zhang, X. Guo, B. Qu, L. Xiao and Z. Chen, ACS Appl. Energy Mater., 2019, 2, 5883–5888 CrossRef CAS.
- P. Wang, B. Chen, R. Li, S. Wang, N. Ren, Y. Li, S. Mazumdar, B. Shi, Y. Zhao and X. Zhang, ACS Energy Lett., 2021, 6, 2121–2128 CrossRef CAS.
- D. Wang, T. He, S. Li, Y. Jiang and M. Yuan, ACS Appl. Energy Mater., 2021 DOI:10.1021/acsaem.1c02666.
- J. Yan, Z. Lin, Q. Cai, X. Wen and C. Mu, ACS Appl. Energy Mater., 2020, 3, 3504–3511 CrossRef CAS.
- P. Caprioglio, M. Stolterfoht, C. M. Wolff, T. Unold, B. Rech, S. Albrecht and D. Neher, Adv. Energy Mater., 2019, 9, 1901631 CrossRef.
- X. Liu, Y. Zhang, L. Shi, Z. Liu, J. Huang, J. S. Yun, Y. Zeng, A. Pu, K. Sun, Z. Hameiri, J. A. Stride, J. Seidel, M. A. Green and X. Hao, Adv. Energy Mater., 2018, 8, 1800138 CrossRef.
- S. Sidhik, A. Cerdan Pasaran, D. Esparza, T. Lopez Luke, R. Carriles and E. De la Rosa, ACS Appl. Mater. Interfaces, 2018, 10, 3571–3580 CrossRef CAS PubMed.
- J. Cao, X. Lv, P. Zhang, T. T. Chuong, B. Wu, X. Feng, C. Shan, J. Liu and Y. Tang, Adv. Mater., 2018, 30, e1800568 CrossRef PubMed.
- M. Kim, G.-H. Kim, T. K. Lee, I. W. Choi, H. W. Choi, Y. Jo, Y. J. Yoon, J. W. Kim, J. Lee, D. Huh, H. Lee, S. K. Kwak, J. Y. Kim and D. S. Kim, Joule, 2019, 3, 2179–2192 CrossRef CAS.
- M. Sun, J. Shu, C. Zhao, J. Wu, H. Guo, Y. Guo, X. Yin, Y. Lin, Z. Tan, M. He and L. Wang, ACS Appl. Mater. Interfaces, 2022, 14, 13352–13360 CrossRef CAS PubMed.
- J. W. Jung, S. T. Williams and A. K. Y. Jen, RSC Adv., 2014, 4, 62971–62977 RSC.
- Y. Liu, Q. Chen, H.-S. Duan, H. Zhou, Y. Yang, H. Chen, S. Luo, T.-B. Song, L. Dou, Z. Hong and Y. Yang, J. Mater. Chem. A, 2015, 3, 11940–11947 RSC.
- K. Liu, S. Chen, J. Wu, H. Zhang, M. Qin, X. Lu, Y. Tu, Q. Meng and X. Zhan, Energy Environ. Sci., 2018, 11, 3463–3471 RSC.
- H. S. Kim, J. W. Lee, N. Yantara, P. P. Boix, S. A. Kulkarni, S. Mhaisalkar, M. Gratzel and N. G. Park, Nano Lett., 2013, 13, 2412–2417 CrossRef CAS PubMed.
- T. Zhu, Y. Yang, S. Zhou, X. Yao, L. Liu, W. Hu and X. Gong, Chin. Chem. Lett., 2020, 31, 2249–2253 CrossRef CAS.
- N. D. Pham, V. T. Tiong, P. Chen, L. Wang, G. J. Wilson, J. Bell and H. Wang, J. Mater. Chem. A, 2017, 5, 5195–5203 RSC.
|
This journal is © The Royal Society of Chemistry 2022 |
Click here to see how this site uses Cookies. View our privacy policy here.