DOI:
10.1039/D2RA03759A
(Paper)
RSC Adv., 2022,
12, 32898-32902
Facile synthesis of Cu N-lauroyl sarcosinate nanozymes with laccase-mimicking activity and identification of toxicity effects for C. elegans
Received
18th June 2022
, Accepted 8th November 2022
First published on 16th November 2022
Abstract
Nanozyme is a material with enzyme-like catalytic activity, which has been widely used in environmental, antibacterial, and other fields of research. However, there are few reports on the toxicity of nanozymes. In this work, nanozymes co-assembled from sodium N-lauroyl sarcosinate (Ls) and Cu ions possess a Cu(I)–Cu(II) electron transfer system similar to that of natural laccases. Reaction kinetic studies show that the catalyst follows a typical Michaelis–Menten model. Cu–N-lauroyl sarcosinate nanozyme (Cu-Ls NZ) possess excellent laccase-like activity to oxidize a variety of phenol-containing substrates, such as phenol, 4-iodophenol, and 2,4,5-trichlorophenol. To evaluate the toxicity of the material, the nematode C. elegans was exposed to various concentrations of Cu-Ls NZ. Effects on physiological levels were determined. The results showed that high doses of Cu-Ls NZ increased the amount of reactive oxygen species (ROS), decreased the locomotor activity of nematodes, and inhibited their larval growth.
1. Introduction
Phenols are widely used as starting materials in both the industrial and academic research environment.1,2 Phenol is a common chemical and constitutes a severe environmental hazard.3,4 With the continuous demand for chemical raw materials by industry, a large amount of high-salinity wastewater containing phenol is discharged into the aquatic environment. The flow of dangerous chemicals into the sea may lead to the phenol-polluted ocean.3 Currently, treatments of wastewater containing high concentrations of phenol consist mainly of physicochemical methods, such as adsorption and solvent extraction.5 Recently, considerable efforts have been dedicated to creating enzyme-mimicking catalysts to replace natural enzymes in practical applications.
As a common amide-containing surfactant, sodium N-lauroyl sarcosinate (Ls) can be found in detergents, primarily in shampoo, bubble-bath pastes, and cleaning cream.6 In addition, Ls is widely used in the construction industry as an excellent corrosion inhibitor.7 Ls interact with other ions, such as heavy metals, and is likely to concentrate such ions and potentially modulate their toxicity. Surfactants exhibit various self-assembled structures, some of which have potential applications in drug delivery and cosmetic and food formulations.8 Aqueous mixtures of Ls and the cationic surfactant N-dodecyl pyridinium chloride can produce small stable vesicles.9 Starting from these studies, when investigating laccase mimics, we observed that Ls formed complexes with Cu effectively catalyze the reaction of phenol derivatives with oxygen. Since laccases can catalyze the oxidation of various compounds containing phenolic structures,10 enzyme-mimicking catalysts have received a lot of attention as possible replacements of natural enzymes in the field of environmental science.11,12 In amide-containing surfactants, the amide group can act as a donor or an acceptor of H bonds and constitutes a readily accessible source of electrostatic, H-bonding, and hydrophobic elements present in proteins.7
To evaluate the toxicity of Cu-Ls NZ, the nematode Caenorhabditis elegans (C. elegans) was exposed to various concentrations of the material. In the natural environment, C. elegans inhabits the layer of water surrounding soil particles and mainly feeds on bacteria in compost, which renders the organism a suitable model for toxicity testing in soil and aquatic environments.13 C. elegans has also emerged as a powerful model for environmental toxicology, particularly for studying chemical genotoxicity.14,30
This study aimed to investigate the enzymatic reaction properties of Cu-Ls NZ and toxicity in the in vivo model organism.
2. Experimental section
2.1 Reagents
Copper(II) sulfate (CuSO4·5H2O, 99.9%), 4-amino antipyrine (4-AP, 98%), 2,4-dichlorophenol (2,4-DP, 98%), and 2-(N-morpholino) ethanesulfonic acid (MES, 99.5%) monohydrate were obtained from Aladdin Chemistry Co., Ltd (Shanghai, China). Sodium N-lauroylsarcosinate (98%) was obtained from Beijing Dingguo Changsheng Biotechnology Co., Ltd (Beijing, China). All other reagents were commercially available and used without further purification.
2.2 Synthesis of Cu-Ls NZ
The Cu-Ls NZ were prepared by first mixing Ls (25 mM, 500 μL) and CuSO4 (50 mM, 500 μL), dissolved in ultrapure water. Subsequently, the reaction product was collected by centrifugation (12
000 rpm, 5 min) and washed with deionized water three times to remove the excess reactant. Finally, the product was frozen-dried. Before the subsequent experiment, the aqueous solution of Cu-Ls NZ would be sonicated for 5 minutes to obtain a fine dispersion.
Other materials are synthesized using the same method, exchanging copper ions for other metal ions.
2.3 Catalytic activity assay
The catalytic activity of the Cu-Ls NZ was determined using 2,4-DP as the substrate and 4-AP as the chromogenic reagent.12,26 First, 100 μL of a 2,4-DP aqueous solution (1 mg mL−1) and 100 μL of a 4-AP aqueous solution (1 mg mL−1) were mixed with 700 μL of MES buffer (30 mmol L−1, pH 6.8). Then, 100 μL of the Cu-Ls NZ aqueous dispersion (1 mg mL−1) was added to the mixture. The reaction was maintained at 25 °C for 1 h. The reaction solution was monitored at 510 nm (UV-2501PC, Shimadzu, Japan), and the reaction rate, V, in mmol L−1 min−1 was defined as the production of chromogenic products (mmol L−1) per time unit (min). A molar absorption coefficient of 13.6 L mmol−1 cm−1 was used in the calculations.
After the reaction, the Cu-Ls NZ mixture was centrifuged, and the supernatant and precipitation was incubated with 800 μL MES buffer, 100 μL 2,4-DP and 100 μL 4-AP. The reaction was carried out at room temperature for 1 hour.
2.4 Analysis of stability
To study the effect of pH, Cu-Ls NZ was separately suspended at different pH (1–12) for 6 h before the activity assay. The relative activity was compared with that at pH 7. The effect of temperature was measured by storing Cu-Ls NZ dispersions at different temperatures (30–90 °C) for 30 min, and the activity at 30 °C was taken as a reference. The effect of ionic strength was measured by incubating Cu-Ls NZ to test solutions of different NaCl concentrations (0, 150, 300, and 500 mM). For these studies, the reaction was allowed to proceed for 1 h before the absorbance of the supernatant was measured at 510 nm. The long-term storage stability was established daily by determining the residual activity of Cu-Ls NZ dispersed in ultrapure water stored at room temperature.
2.5 Determination of enzyme kinetic parameters
The initial reaction rates for the reactions were obtained with different concentrations of 2,4-DP (10, 20, 40, 60, 80, and 100 μg mL−1). In all these reactions, the concentration of 4-AP was in excess at 1 mg mL−1. The Michaelis–Menten equation calculated the kinetic parameters (Km and Vmax).
2.6 Effect of metal ions and substrates on the laccase-mimicking activity
2.6.1 For metal ions. Materials formed from different metal ions and Ls were incubated with 700 μL MES buffer and 2,4-DP and 4-AP excess of 100 μg mL−1. The reaction was carried out at room temperature for 1 hour.
2.6.2 For substrates. Incubate different phenolic compounds with 700 μL MES buffer, 100 μL 4-AP and 100 μL 1 mg mL−1 Cu-Ls NZ. The reaction was carried out at room temperature for 1 hour. The absorbance at 510 nm was then recorded with UV-2550.
2.7 C. elegans strain and culture
Wild-type, Bristol strain N2 C. elegans was obtained from the Caenorhabditis Genetics Center (CGC, University of Minnesota, USA) and maintained at 20 °C. C. elegans species were grown in Petri dishes on nematode growth medium (NGM) and fed with E. coli OP50.
2.8 Acute toxicity of Cu-Ls NZ exposure in C. elegans
Synchronized L4 worms were exposed to various concentrations of Cu-Ls NZ for 24, 48 h in K-medium (3.1 g NaCl and 2.4 g KCl in 1000 mL deionized water).27 Twenty worms were added to each well of a 24-microtiter plate. Following exposure, the live and dead nematodes were scored. The experiment was repeated three times independently.
2.9 Measurement of reactive oxygen species
Endogenous reactive oxygen species (ROS) levels were measured using 2′,7′-dichlorofluorescein diacetate (H2DCF-DA).28 Worms were treated with 50 μg mL−1 Cu-Ls NZ or deionized water for 48 h and then collected. Then, the nematodes were incubated with 1 mM H2DCF-DA for 30 min. Fluorescence intensity was measured at excitation and emission wavelengths of 470 and 550 nm, respectively. The assay was performed in three independent trials. The software program ImageJ 15.2v was used to quantify the fluorescence intensity.29
2.10 Analysis of body length
L1 stage worms were treated with Cu-Ls NZ or deionized water for 24, 48, and 72 h. The worms were placed on 2% agarose pads and were recorded on a stereoscope model BK1201 (Chongqing COIC Industrial Co., Ltd, China). The body length of the worms was measured using Image J 15.2v software.29
2.11 Body bend assay
To measure body bending, worms treated 50 μg mL−1 Cu-Ls NZ or deionized water for 48 h were placed on M9 buffer without food, and the number of sinusoidal curves made during locomotion in 20 s was scored. This assay was repeated independently three times. Each group included at least 30 worms.
2.12 Reproduction assay
Synchronized L4 larvae (n = 3) were randomly transferred to fresh NGM plates treated with 50 μg mL−1 Cu-Ls NZ or deionized water. They were transferred onto a fresh NGM plate every 24 h. The eggs were then allowed to hatch and were counted at the L2 or L3 stage. The total number of progenies was referred to as the initial reproduction.
2.13 Pharyngeal pumping assay
For pharyngeal pumping experiments,24 worms (n = 10) were treated with 50 μg mL−1 Cu-Ls NZ or deionized water for 24 h on NGM plates. The pharyngeal pumping was recorded on the COIC stereoscope mentioned earlier and was counted for 10 s.
2.14 Statistical analysis
Statistical analysis was performed using Prism 7 software from GraphPad. Data was analyzed by Student's t-test, and values were presented as mean ± SD. Statistical differences were considered significant at p < 0.05 (*p < 0.05; **p < 0.01; ***p < 0.001, comparison to 0 μg mL−1, ns: no significance).
3. Results and discussion
3.1 Cu-Ls NZ with laccase-like activity
The laccase-like catalytic activity of the Cu-Ls NZ was measured via a redox reaction of 2,4-DP and 4-AP in the presence of oxygen.11,12 Each compound alone does not absorb light in the visible region. The chromogenic product can be easily detected by absorbance at 510 nm. As shown in Fig. 1A the colorless reaction solution immediately turned pink after the addition of Cu-Ls NZ, and the characteristic peak observed at 510 nm indicates the laccase-like catalytic ability of this material. The Cu-Ls NZ was centrifuged, and the centrifuged clear solution exhibited almost no activity in the catalytic test reaction, while the solid material was active, which indicated that the catalytic activity originated from Cu-Ls NZ instead of the free Cu2+ in the supernatant Fig. 1B. Kinetic measurements were conducted with natural laccase and Cu-Ls NZ at different substrate concentrations to obtain the enzyme kinetic parameters. The Km and Vmax values were calculated using the Michaelis–Menten model. The results listed in Table 1 suggest activity and properties of Cu-Ls NZ similar to the natural enzyme.
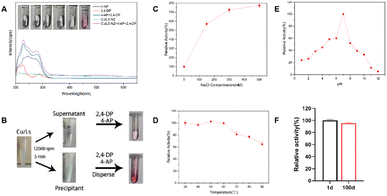 |
| Fig. 1 (A) UV-vis spectra of the three substrates and their reaction product (inset: 0.1 mg mL−1 of 2,4-DP and 4-AP in pH 7.0 MES buffer and oxidation with 0.1 mg mL−1 of Cu-Ls NZ). (B) Photographs of Cu-Ls NZ reacted with 2,4-DP in pH 7.0 MES buffer after centrifugation. Stability of the Cu-Ls NZ at different (C) NaCl concentrations, (D) temperature and (E) pH. (F) Long-term stability of Cu-Ls NZ. | |
Table 1 The enzyme kinetic parameters of laccase and Cu-Ls NZ
Catalysts |
Km (mM) |
Vmax (mM min−1) |
Cu-Ls NZ |
3.4 ± 0.89 |
0.0011 ± 0.00015 |
Laccase |
0.15 ± 0.021 |
0.012 ± 0.00053 |
The stability aspect of Cu-Ls NZ was systematically investigated under various harsh conditions. First, the effect of ionic strength was determined. Unexpectedly, the activity of Cu-Ls NZ increased by a factor of 8.5 at a NaCl concentration of 500 mM Fig. 1C. The high concentration of NaCl might have resulted in a decreased solubility of 2,4-DP and 4-AP via the salting-out effect.12 Subsequently, these compounds might have been preferentially adsorbed by the Cu-Ls NZ and then converted. In the following investigations on the effect of temperature, Cu-Ls NZ was exposed to temperatures from 30 to 90 °C for 30 min prior to the activity tests. As evident from Fig. 1D the Cu-Ls NZ activity was influenced only to a limited extent by the thermal treatment. Compared to the reaction after exposure to 30 °C, the activity decreased only by 36% after treatment at 90 °C. Next, the Cu-Ls NZ was exposed to a range of buffers from pH 1 to 12 at room temperature for 6 h Fig. 1E. In these experiments, the activity of Cu-Ls NZ was highest at pH 7. Concerning the catalyst's long-term stability, Cu-Ls NZ maintained an excellent catalytic activity with a retention of 95% after 100 days in a sterile water solution Fig. 1F.
Since sewage contains different metal ions,15 we investigated whether the combination of other metal ions with Ls exhibited a laccase-like activity. Although precipitation with Ls ligand was observed with all tested metal ions (Fig. 2A, top tubes), their activity was shallow except for Cu2+ (Fig. 2A, below tubes). Accordingly, the activity of Cu2+ was significant. It may be owing to the Cu-Ls NZ was the similar structure as the natural laccase.
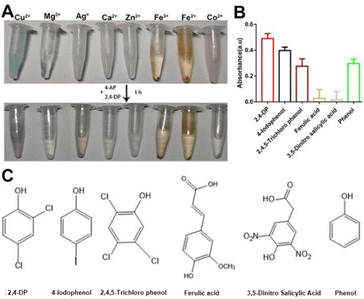 |
| Fig. 2 (A) The photographs of NPs by mixing Ls with various metal ions. The red color indicates laccase-like activity. (B) Catalytic efficacy of Cu-Ls NZ to oxidize substrates and (C) the chemical structures of different tested phenols. | |
To test the Cu-Ls NZ activity toward different substrates, 4-AP was mixed with selected phenolic compounds (Fig. 2B), of which the chemical structures are displayed in Fig. 2C. The results of these experiments demonstrate that Cu-Ls NZ possesses the capability to oxidize each of the phenolic compounds.
3.2 Structural characterization of Cu-Ls NZ
The co-assembly of Cu ions with Ls occurred when mixing CuSO4 and Ls at a molar ratio of 2
:
1. The structural morphology of Cu-Ls NZ was examined by SEM. The as-synthesized Cu-Ls NZ exhibited clear, ellipsoidal micelle-like structures of different sizes with a regular and smooth shape with approximately 50–500 nm (Fig. 3A and B).
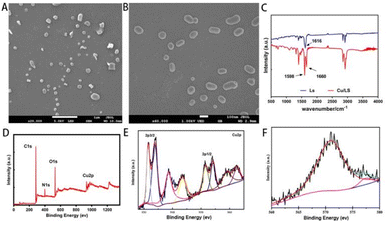 |
| Fig. 3 (A) and (B) SEM micrographs of Cu-Ls NZ. (C) FTIR spectra of Ls and Cu-Ls NZ. (D) XPS full scan spectrum of Cu-Ls NZ. (E) XPS spectrum of Cu 2p. (F) The Auger Cu LMM spectrum of Cu-Ls NZ. | |
The spectrum of the Ls featured a strong absorption band at 1616 cm−1, due to the asymmetric stretching vibration of the carboxylate moiety, along with symmetric stretching vibrations at 1402 cm−1.16 The FTIR spectrum of Cu-Ls NZ in the wavelength range of 4000–500 cm−1 confirmed that the Cu-Ls NZ was formed through the coordination of Ls with Cu ions (Fig. 3C). The splitting of the broad C
O asymmetric stretching vibration band at 1616 cm−1 reflected the formation of Cu–O coordination (1598 and 1660 cm−1).11,12
XPS was performed to determine the oxidation number of Cu ions in the Cu-Ls NZ. The peaks ascribed to Cu, O, and C were identified, suggesting that the Cu-Ls NZ were organic–inorganic hybrids (Fig. 3D). The spectra revealed a core region (Cu 2p3/2 and Cu 2p1/2) along with solid Cu(II) satellite peaks (Fig. 3E). The peaks with binding energies of 933.7 and 954.8 eV in the Cu 2p XPS spectrum were characteristic of Cu(II) 2p3/2 and Cu(II) 2p1/2. Two firm satellite peaks also verified that Cu(II) was in a paramagnetic chemical state.17 Peaks at lower binding energy at 931.5 and 951.4 eV indicated the presence of Cu0 or Cu+.17,18 In addition, the Auger Cu LMM spectrum revealed the detailed oxidation states of Cu in Cu-Ls NZ and supported the existence of Cu+ and Cu2+ assigned to 572.9 eV and 568.2 eV, respectively (Fig. 3F).
3.3 Toxicity assessment of Cu-Ls NZ in Caenorhabditis elegans
Nanoparticles usually exhibit biological toxicity.19 To assess the toxicity of Cu-Ls NZ we first treated N2 worms with Cu-Ls NZ at concentrations ranging from 0 to 200 μg mL−1 in 96-well plates at 20 °C. After 24 or 48 h exposure from L4 stage, there was no significant difference between the control group and the Cu-Ls NZ group. These results indicated that the high concentration of 50 μg mL−1 Cu-Ls NZ is safe, and the acute exposure can be considered as nonlethal in C. elegans (Fig. 4A). This concentration was considered for further toxicity studies. Some nanoparticles usually release Cu in the environment,20 resulting in Cu ion accumulation in organisms and the possible promotion of oxidative stress. Excess of ROS cause death21 and other adverse effects, such as growth and development22 and fat accumulation.23 The results of our investigations demonstrated that there is no significant change in ROS levels increased when exposing C. elegans to Cu-Ls NZ at concentrations of 50 μg mL−1 (Fig. 4B).
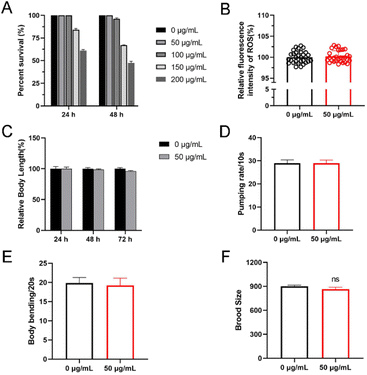 |
| Fig. 4 Effect of Cu-Ls NZ exposure in C. elegans. (A) Survival percent of N2 worms raised at 20 °C in 24-well plates containing 0, 50, 100, 150, or 200 μg mL−1 Cu-Ls NZ or control plates without Cu-Ls NZ after 24 and 48 h. (B) Effects of Cu-Ls NZ on ROS levels in N2 worms. The effect of Cu-Ls NZ on C. elegans physiological parameters: (C) body length (∼30); (D) body bending (∼70); and (E) reproduction (n = 3); (F) pumping rate (n = 10). | |
3.4 Inhibition on body length and locomotion behavior
The body length was used to evaluate the growth of C. elegans.24 The L1 stage nematodes were exposed to 100 μg mL−1 of Cu-Ls NZ for 24, 48, and 72 h in K-medium. After exposure to 50 μg mL−1 of Cu-Ls NZ, there was no significant change in body length compared with controls (Fig. 4C). Our results showed that 50 μg mL−1 Cu-Ls NZ had no significant effect on C. elegans development.
Body bends were used to evaluate the effects on locomotive behavior of C. elegans.25 At a 48 h exposure of C. elegans L4 stage to Cu-Ls NZ in K-medium, no significant reduction of the body bend was detected at the concentrations of 50 μg mL−1 (Fig. 4D). This result indicated that 50 μg mL−1 Cu-Ls NZ could had no significant effect on C. elegans exercise activity.
4. Conclusions
In summary, we documented the synthesis and functional characterization of Cu-Ls NZ and their effects on an animal model. Cu-Ls NZ was synthesized by a simple synthetic method and displayed laccase-like catalytic activity. According to the SEM and FTIR results, Cu-Ls NZ exhibited a stable micelle structure and stable binding. The characterization and catalytic results implied that a similar phenomenon might occur in discharged domestic sewage in nature with the possible capability to oxidize phenolic compounds. In addition, we demonstrated that Cu-Ls NZ do not exhibit any effects regarding acute toxicity or reproductive toxicity on C. elegans over a range of concentrations. Further evaluation of the toxicity of Cu-Ls NZ in cell models and mouse models is still required. In general, this work provided a novel nanozyme with low toxicity.
Author contributions
Fanwei Meng: conceptualization, data curation, investigation, methodology, resources, writing – original draft, visualization, formal analysis, writing – review & editing. Guofang Ma: data curation, software, investigation, Methodology., Resources. Jiahe Qiu: data curation. Zhendong Fu: data curation. Jiaqing Yan: conceptualization, supervision, resources, project administration, writing – review & editing. Liping Wang: conceptualization, supervision, resources, project administration, writing – review & editing.
Conflicts of interest
There are no conflicts to declare.
Acknowledgements
We thank the Education department of Jilin Province (No. JJKH20211211KJ). We thank the Caenorhabditis Genetics Centre (CGC) supported by the NIH Office of Research Infrastructure Programs (P40 OD010440) and the Caldwell Laboratory, University of Alabama, for the supply of the C. elegans strains. This work was supported by the Science and Technology Development Program of Jilin Province, China (No. 20200404114YY); the Department of Education Program of Jilin Province, China (No. JJKG20211211KJ).
References
- W. T. Wu, L. Zhang and S. L. You, Chem. Soc. Rev., 2016, 45, 1570–1580 RSC.
- H. Li, F. Meng, W. Duan, Y. Lin and Y. Zheng, Ecotoxicol. Environ. Saf., 2019, 184, 109658 CrossRef CAS PubMed.
- S. Duan, L. Wu, J. Li, Y. Huang, X. Tan, T. Wen, T. Hayat, A. Alsaedi and X. Wang, J. Hazard. Mater., 2019, 373, 580–590 CrossRef CAS PubMed.
- B. Marrot, A. Barrios-Martinez, P. Moulin and N. Roche, Biochem. Eng. J., 2006, 30, 174–183 CrossRef CAS.
- S. Ghosh and J. Dey, J. Phys. Chem. B, 2015, 119, 7804–7815 CrossRef CAS PubMed.
- D. R. Perinelli, M. Cespi, N. Lorusso, G. F. Palmieri, G. Bonacucina and P. Blasi, Langmuir, 2020, 36, 5745–5753 CrossRef CAS PubMed.
- B. Li, P. Somasundaran and P. Patra, Adv. Colloid Interface Sci., 2014, 210, 72–77 CrossRef CAS PubMed.
- S. Ghosh and J. Dey, J. Colloid Interface Sci., 2011, 358, 208–216 CrossRef CAS PubMed.
- J. Su, J. Fu, Q. Wang, C. Silva and A. Cavaco-Paulo, Crit. Rev. Biotechnol., 2018, 38, 294–307 CrossRef CAS PubMed.
- S. Shams, W. Ahmad, A. H. Memon, Y. Wei, Q. Yuan and H. Liang, RSC Adv., 2019, 9, 40845–40854 RSC.
- H. Liang, F. Lin, Z. Zhang, B. Liu, S. Jiang, Q. Yuan and J. Liu, ACS Appl. Mater. Interfaces, 2017, 9, 1352–1360 CrossRef CAS PubMed.
- Y. Nagar, R. S. Thakur, T. Parveen, D. K. Patel, K. R. Ram and A. Satish, Chemosphere, 2020, 246, 125730 CrossRef CAS PubMed.
- C. Weinhouse, L. Truong, J. N. Meyer and P. Allard, Environ. Mol. Mutagen., 2018, 59, 560–575 CrossRef CAS PubMed.
- M. Guan, M. Wang, W. Qi, R. Su and Z. He, Front. Chem. Sci. Eng., 2020, 15, 310–318 CrossRef.
- C. J. Munro, M. A. Nguyen, C. Falgons, S. Chaudhry, M. Olagunjo, A. Bode, C. Bobe, M. E. Portela, M. R. Knecht and K. M. Collins, Environ. Sci.: Nano, 2020, 7, 645–655 RSC.
- Z. Z. Yang, Y. T. Yu, H. R. Lin, D. C. Liao, X. H. Cui and H. B. Wang, Free Radical Biol. Med., 2018, 129, 310–322 CrossRef CAS PubMed.
- C. A. Schneider, W. S. Rasband and K. W. Eliceiri, Nat. Methods, 2012, 9, 671–675 CrossRef CAS PubMed.
- H. Li, X. Yu, C. Li, L. Ma, Z. Zhao, S. Guan and L. Wang, Food Funct., 2021, 12, 1219–1231 RSC.
- A. Gogoi, K. Taki and M. Kumar, Environ. Res., 2020, 183, 109265 CrossRef CAS PubMed.
- A. Li, X. Mu, T. Li, H. Wen, W. Li, Y. Li and B. Wang, Nanoscale, 2018, 10, 11948–11954 RSC.
- S. Ma, Z. Han, K. Leng, X. Liu, Y. Wang, Y. Qu and J. Bai, Small, 2020, 16, e2001384 CrossRef PubMed.
- W. Yan, J. Zhang, M. Abbas, Y. Li, S. Z. Hussain, S. Mumtaz, Z. Song, I. Hussain and B. Tan, Nanomaterials, 2020, 10, 1678 CrossRef CAS PubMed.
- P. Kumar, B. Anand, Y. F. Tsang, K. H. Kim, S. Khullar and B. Wang, Environ. Res., 2019, 176, 108488 CrossRef CAS PubMed.
- X. Guan, Q. Li, T. Maimaiti, S. Lan, P. Ouyang, B. Ouyang, X. Wu and S. T. Yang, J. Hazard. Mater., 2021, 409, 124521 CrossRef CAS PubMed.
- A. Vaccaro, Y. Kaplan Dor, K. Nambara, E. A. Pollina, C. Lin, M. E. Greenberg and D. Rogulja, Cell, 2020, 181, 1307–1328 CrossRef CAS PubMed.
- P. Palozza, N. Parrone, R. Simone and A. Catalano, Curr. Med. Chem., 2011, 18, 1846–1860 CrossRef CAS PubMed.
- K. Wang, S. Chen, C. Zhang, J. Huang, J. Wu, H. Zhou, L. Jin, X. Qian, J. Jin and J. Lyu, Exp. Gerontol., 2018, 112, 20–29 CrossRef CAS PubMed.
- X. Xiao, X. Zhang, C. Zhang, J. Li, Y. Zhao, Y. Zhu, J. Zhang and X. Zhou, Toxicol. Res., 2019, 8, 630–640 CrossRef CAS PubMed.
- F. Calahorro, F. Keefe, J. Dillon, L. Holden-Dye and V. O’Connor, J. Exp. Biol., 2019, 222(3), jeb189423 Search PubMed.
- L. Kong, X. Gao, J. Zhu, T. Zhang, Y. Xue and M. Tang, Reproductive toxicity induced by nickel nanoparticles in Caenorhabditis elegans, Environ. Toxicol., 2017, 32(5), 1530–1538 CrossRef CAS PubMed.
Footnote |
† Fanwei Meng and Guofang Ma are co-first authors. |
|
This journal is © The Royal Society of Chemistry 2022 |
Click here to see how this site uses Cookies. View our privacy policy here.