DOI:
10.1039/D2RA04640G
(Paper)
RSC Adv., 2022,
12, 24579-24588
Synthesis and evaluation of the antioxidant activity of 3-pyrroline-2-ones: experimental and theoretical insights†
Received
25th July 2022
, Accepted 22nd August 2022
First published on
30th August 2022
Abstract
The heterocyclic γ-lactam ring 2-pyrrolidinone has four carbon atoms and one nitrogen atom. Among the group of derivatives of 2-pyrrolidinones, 1,5-dihydro-2H-pyrrol-2-ones, also known as 3-pyrroline-2-ones, play a significant structural role in a variety of bioactive natural compounds. In this study, three-component reactions were used to successfully synthesize six polysubstituted 3-hydroxy-3-pyrroline-2-one derivatives. The antioxidant activity of the compounds was tested by the 2,2-diphenyl-1-picrylhydrazyl (DPPH) assay, identifying 4-ethoxycarbonyl-3-hydroxy-5-(4-methylphenyl)-1-phenyl-3-pyrroline-2-one (4b) as the most promising radical scavenger. Quantum chemistry calculations of the thermodynamics and kinetics of the radical scavenging activity also suggest that 4b is an effective HO˙ radical scavenger, with koverall values of 2.05 × 109 and 1.54 × 1010 M−1 s−1 in pentyl ethanoate and water, respectively. On the other hand, 4b could not scavenge hydroperoxyl radicals in either media. The ability of 4b to scavenge hydroxyl radicals in polar and non-polar environments is comparable to that of conventional antioxidants such as melatonin, gallic acid, indole-3-carbinol, ramalin, or Trolox. Thus 4b may be classed as a promising HO˙ radical scavenger in the physiological environment.
1. Introduction
2-Pyrrolidinone is a γ-lactam heterocyclic ring containing four carbon atoms and one nitrogen atom. Among the family of 2-pyrrolidinone derivatives, 1,5-dihydro-2H-pyrrol-2-ones (also named as 3-pyrroline-2-ones) are important structural subunits of numerous bioactive natural products (Fig. 1). For example, oteromycin that was isolated from fungus strains MF5810 and MF5811 is a HIV-1 integrase inhibitor,1 while the Fusarium pallidoroseum-originating equisetin is a natural antibiotic active against Staphylococcus aureus and Bacillus subtilis2 while it is also a potent inhibitor against HIV-integrase with IC50 values between 7 and 20 µM.1 In addition to natural products, more and more non-natural polysubstituted 1,5-dihydro-2H-pyrrol-2-ones have also been proven to possess a broad spectrum of pharmacological activities as e.g., anticancer,3–7 antibacterial,8–10 anti-HIV-1,11 as well as anti-inflammatory12,13 and antioxidant2,14–18 agents. Based on the promising data it was suggested that 1,5-dihydro-2H-pyrrol-2-one skeleton-containing heterocyclic compounds are potential drug candidates. Therefore, increasing attention has been directed at the synthesis of 1,5-dihydro-2H-pyrrol-2-ones, and especially 3-hydroxy-1,5-dihydro-2H-pyrrol-2-ones.19
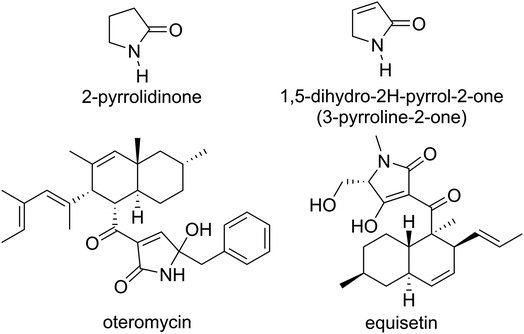 |
| Fig. 1 Natural products containing 3-pyrroline-2-one subunit. | |
Multi-component reactions (MCRs) offer an efficient synthetic pathway in organic chemistry to obtain complicated molecular structures.20 Recently, the synthesis of polysubstituted 3-hydroxy-1,5-dihydro-2H-pyrrol-2-ones has been established based on three-component reactions of aromatic aldehydes, arylamines and dialkyl acetylenedicarboxylate.21–27 The use of sodium diethyl oxalacetate instead of dialkyl acetylenedicarboxylate in the synthesis of polysubstituted 3-hydroxy-1,5-dihydro-2H-pyrrol-2-ones via three-component reactions has also been reported; however, there are still some disadvantages regarding to the use of sodium diethyl oxalacetate that need to be improved.28–30 Therefore, optimizing reaction conditions to synthesize polysubstituted 3-hydroxy-3-pyrroline-2-ones via MCRs involving sodium diethyl oxalacetate is still in demand to the synthetic organic chemist community.
A key bioactivity described above is the potential antioxidant activity of this family of compounds.2,14–18,31 The imbalance between the production and consumption of oxidants in biological systems leads to oxidative stress, that is, chemical breakdown/damage of various biologically important molecules.32 Although there are natural oxidants of various chemical characteristics, free radicals are the main cause of oxidative stress. They are highly reactive and capable of initiating chain reactions, hence propagating molecular damage. A representative reactive oxygen species is the hydroxyl radical.33 It is so reactive that it would effectively assault nearly every organic molecule around the place of its production. This radical is held accountable for the majority of ionizing radiation-induced tissue damage34 and is the most significant cause of oxidative DNA damage.35–37 Inhibiting OH˙ generation would therefore be an effective means of reducing oxidative stress. However, studies of antioxidant activity of 3-hydroxy-3-pyrroline-2-ones are thus far limited: there is no information on the mechanism and kinetics of the antiradical activity, particularly in physiological environments.
The use of computational approaches to investigate the structure–activity relationship and to direct the design of novel medications with enhanced activity is well established,38–42 making it possible to perform the evaluation of the radical (i.e., HO˙ and HOO˙) scavenging activity in silico, benchmarked against experimental data.
Thus, in this study, three-component reactions of aromatic aldehydes, amines and sodium diethyl oxalacetate will be optimized in order to shorten reaction time, increase the yields and eliminate the need for elevated temperature. Consecutively, the 1,1-diphenyl-1-picrylhydrazyl (DPPH) free radical scavenging capacity of six polysubstituted 3-hydroxy-3-pyrroline-2-ones will be measured. Finally, the interaction of the best performing 3-hydroxy-3-pyrroline-2-one with HO˙ and HOO˙ radicals will be evaluated using well-established model chemistry based on the quantum mechanics-based test for the overall free radical scavenging activity (QM-ORSA) protocol.40,43
2. Experimental and computational methods
2.1. Experimental
2.1.1. Chemicals and experimental methods.
All chemicals were purchased from the main chemical suppliers: Merck, Acros, or Sigma Aldrich. For column chromatography, 70–230 mesh silica 60 (E. M. Merck) was used as the stationary phase. Melting points (not corrected) were determined using a Büchi Melting Point B-545 apparatus. NMR spectra were acquired on Bruker Avance II+ 500 MHz or Bruker Avance II+ 600 MHz instruments and chemical shifts (δ) are reported in parts per million (ppm) referenced to tetramethylsilane (TMS) or the internal (NMR) solvent signals. Exact mass measurements were acquired on a quadrupole orthogonal acceleration time-of-flight mass spectrometer (Synapt G2 HDMS, Waters, Milford, MA). Samples were infused at 3 µL min−1 and spectra were obtained in positive (or negative) ionization mode with a resolution of 15
000 (FWHM) using leucine enkephalin as lock mass. High-resolution mass measurements were recorded on a SCIEX X500 QTOF with an electrospray ionization source in a positive ion mode. The temperatures of the source were set at 300 °C. Curtain gas chambers were filled with high-purity nitrogen (25 psi). The capillary voltage was constantly kept at 5500 V. The collision energy was set at 10 V and zero collision energy spread. IDA mode was used to find mass in the range (100 to 1000).
2.1.2. General procedure for the synthesis of 1,5-disubstituted-4-ethoxycarbonyl-3-hydroxy-3-pyrroline-2-one.
Aromatic aldehyde (1 equiv.), amine (1 equiv.), citric acid (2 equiv.) and absolute ethanol (1.0 mL) were mixed in a round bottom flask. The mixture was magnetically stirred at room temperature under an argon atmosphere for 1 hour. Subsequently, sodium diethyl oxalacetate (2 equiv.) was added and the mixture was stirred vigorously at room temperature under Ar atmosphere for 8 hours and the formation of 1,5-disubstituted-4-ethoxycarbonyl-3-hydroxy-3-pyrroline-2-one was followed by TLC (hexane/EtOAc = 5
:
1 and hexane/EtOAc = 5
:
3.5). Then, CH2Cl2 and HCl (5%) were added and the resulting mixture was stirred vigorously for 15 minutes. The organic layer was separated, then washed three times with distilled water and dried over MgSO4. The crude product was purified via recrystallization in the solvent mixture of CH2Cl2 and absolute ethanol or CH2Cl2 and ethylacetate to obtain a pure product.
2.1.3. Spectra data of products.
2.1.3.1. 1,5-Diphenyl-4-ethoxycarbonyl-3-hydroxy-3-pyrroline-2-one (4a).
M.p. 171–173 °C. 1H NMR (500 MHz, CDCl3) δ 9.14 (sbr, 1H; –OH), 7.49 (d, 3J(H,H) = 7.80 Hz, 2H; Ar–H), 7.29–7.23 (m, 7H; Ar–H), 7.10 (t, 3J(H,H) = 7.47 Hz, 1H; Ar–H), 5.75 (s, 1H), 4.20 (m, 2H; OCH2), 1.18 ppm (t, 3J(H,H) = 7.13 Hz, 3H; CH3). 13C NMR (125 MHz, CDCl3) δ 165.21, 162.99, 156.55, 136.36, 135.18, 129.05, 128.68, 128.61, 127.63, 125.93, 122.38, 113.29, 61.68, 61.35, 14.03 ppm. HRMS (ESI-TOF MS/MS) m/z [M + H]+ calcd for C19H17NO4: 324.1236; found: 324.1223.
2.1.3.2. 4-Ethoxycarbonyl-3-hydroxy-5-(4-methylphenyl)-1-phenyl-3-pyrroline-2-one (4b).
M.p. 206–207 °C. 1H NMR (500 MHz, CDCl3) δ 9.09 (sbr, 1H; –OH), 7.49 (dd, 3J(H,H) = 8.64 Hz, 4J(H,H) = 1.08 Hz, 2H; Ar–H), 7.27 (m, 2H; Ar–H), 7.11 (m, 3H; Ar–H), 7.05 (d, 3J(H,H) = 7.86 Hz, 2H; Ar–H), 5.72 (s, 1H), 4.19 (m, 2H; –OCH2–), 2.26 (s, 3H; CH3), 1.20 ppm (t, 3J(H,H) = 7.14 Hz, 3H; CH3). 13C NMR (125 MHz, CDCl3) δ 165.21, 193.01, 156.37, 138.36, 136.45, 132.04, 129.39, 129.03, 127.48, 125.85, 122.37, 113.37, 61.45, 61.34, 21.24, 14.07 ppm. HRMS (ESI-TOF MS/MS) m/z [M + H]+ calcd for C20H19NO4: 338.1392; found: 338.1383.
2.1.3.3. 4-Ethoxycarbonyl-3-hydroxy-5-(4-nitrophenyl)-1-phenyl-3-pyrroline-2-one (4c).
M.p. 173–175 °C. 1H NMR (500 MHz, CDCl3) δ 9.05 (sbr, 1H; –OH), 8.12 (d, 3J(H,H) = 8.40 Hz, 2H; Ar–H), 7.43 (m, 4H; Ar–H), 7.28 (t, 3J(H,H) = 7.71 Hz, 2H; Ar–H), 7.13 (t, 3J(H,H) = 7.43 Hz, 1H; Ar–H), 5.85 (s, 1H), 4.21 (m, 2H; OCH2), 1.21 ppm (t, 3J(H,H) = 7.15 Hz, 3H; CH3). 13C NMR (125 MHz, CDCl3) δ 164.66, 162.65, 156.98, 148.16, 142.94, 135.84, 129.44, 128.69, 126.53, 125.07, 122.27, 112.33, 61.78, 60.79, 14.17 ppm. HRMS (ESI-TOF MS/MS) m/z [M + H]+ calcd for C19H16N2O6: 369.1087; found: 369.1081.
2.1.3.4. 1-Benzyl-4-ethoxycarbonyl-3-hydroxy-5-(4-methylphenyl)-3-pyrroline-2-one (4d).
M.p. 184–186 °C. 1H NMR (500 MHz, CDCl3) δ 9.05 (sbr, 1H; –OH), 7.30 (m, 3H; Ar–H), 7.16 (d, 3J(H,H) = 7.80 Hz, 2H; Ar–H), 7.12 (dd, 3J(H,H) = 7.94 Hz, 4J(H,H) = 2.02 Hz, 2H; Ar–H), 6.98 (d, 3J(H,H) = 8.09 Hz, 2H; Ar–H), 5.18 (d, 2J(H,H) = 14.79 Hz, 1H; –CH2N), 4.84 (s, 1H), 4.08 (m, 2H; OCH2), 3.54 (d, 2J(H,H) = 14.83 Hz, 1H; –CH2N), 2.36 (s, 3H; CH3), 1.08 ppm (t, 3J(H,H) = 7.16 Hz, 3H; CH3). 13C NMR (125 MHz, CDCl3) δ 165.58, 163.59, 157.75, 138.79, 136.55, 131.53, 129.67, 128.94, 128.69, 127.97, 127.86, 113.43, 61.13, 59.54, 44.01, 21.37, 14.02 ppm. HRMS (ESI-quadrupole) m/z [M + H]+ calcd for C21H21NO4: 352.1549; found: 352.1535.
2.1.3.5. 1-Benzyl-4-ethoxycarbonyl-3-hydroxy-5-(4-nitrophenyl)-3-pyrroline-2-one (4e).
M.p. 224–227 °C. 1H NMR (500 MHz, DMSO-d6) δ 12.01 (sbr, 1H; –OH), 8.14 (d, 3J(H,H) = 8.65 Hz, 2H; Ar–H), 7.40 (d, 3J(H,H) = 8.57 Hz, 2H; Ar–H), 7.25 (m, 3H; Ar–H), 7.05 (dd, 3J(H,H) = 7.72 Hz, 4J(H,H) = 1.88 Hz, 2H; Ar–H), 5.16 (s, 1H), 4.78 (d, 2J(H,H) = 15.35 Hz, 1H; –CH2N), 3.98 (dq, 3J(H,H) = 7.03 Hz, 2J(H,H) = 14.09 Hz, 1H; –OCH2–), 3.92 (dq, 3J(H,H) = 6.96 Hz, 2J(H,H) = 14.00 Hz, 1H; –OCH2–), 3.80 (d, 2J(H,H) = 15.37 Hz, 1H; –CH2N), 1.01 ppm (t, 3J(H,H) = 7.10 Hz, 3H; CH3). 13C NMR (125 MHz, CDCl3) δ 164.88, 161.69, 154.09, 147.32, 144.04, 136.20, 129.29, 128.54, 127.77, 127.41, 123.61, 110.97, 59.64, 59.57, 44.18, 13.92 ppm. HRMS (ESI-quadrupole) m/z [M + H]+ calcd for C20H18N2O6: 383.1243; found: 383.1231.
2.1.3.6. 4-Ethoxycarbonyl-3-hydroxy-5-(4-methylphenyl)-1-(3-nitrophenyl)-3-pyrroline-2-one (4f).
M.p. 179–181 °C. 1H NMR (500 MHz, CDCl3) δ 9.08 (sbr, 1H; –OH), 8.30 (t, 4J(H,H) = 2.17 Hz, 1H; Ar–H), 8.09 (dd, 3J(H,H) = 8.35 Hz, 4J(H,H) = 2.29 Hz, 1H; Ar–H), 7.93 (dd, 3J(H,H) = 8.25 Hz, 4J(H,H) = 2.14 Hz, 1H; Ar–H), 7.45 (t, 3J(H,H) = 8.22 Hz, 1H; Ar–H), 7.13 (d, 3J(H,H) = 8.18 Hz, 2H; Ar–H), 7.08 (d, 3J(H,H) = 7.93 Hz, 2H; Ar–H), 5.76 (s, 1H), 4.20 (m, 2H; –OCH2), 2.67 (s, 3H; CH3), 1.20 ppm (t, 3J(H,H) = 7.11 Hz, 3H; CH3). 13C NMR (125 MHz, CDCl3) δ 165.24, 163.12, 156.11, 148.55, 139.05, 137.75, 131.16, 130.00, 129.81, 127.43, 127.34, 120.08, 116.06, 114.01, 61.70, 61.21, 21.29, 14.07 ppm. HRMS (ESI-quadrupole) m/z [M + H]+ calcd for C20H18N2O6: 383.1243; found: 383.1232.
2.1.4. DPPH assay.
Radical-scavenging properties of polysubstituted 3-hydroxy-3-pyrroline-2-ones were evaluated against 1,1-diphenyl-2-picrylhydrazyl (DPPH) radical.44–48 DPPH solution (1 mM) was prepared in methanol and solutions of each polysubstituted 3-hydroxy-3-pyrroline-2-one were prepared in DMSO at various concentrations (128, 32 and 8 µg mL−1). Then, 200 µL DPPH solution was added to 1.28 µL samples at each tested concentration and the free radical scavenging reactions were carried out on a 96-well plate at 37 °C for 30 minutes. The absorbance was measured at 517 nm wavelength by a BioTek Epoch 2 Microplate Spectrophotometer. The percentage of free radical scavenging was calculated as SP(%) = [(OD0 − OD1)/OD0] × 100, where OD0 was defined as the final absorbance of the control reaction with quercetin as the reference antioxidant, and OD1 stands for the absorbance in the presence of the sample. Each experiment was repeated three times and quercetin was used as the positive control.
2.2. Computational details
All DFT calculations were carried out with the Gaussian 09 suite of programs.49 M06-2X functional50 and 6-311++G(d,p) basis set were used for all calculations. The M06-2X functional offers one of the most reliable methods to study the thermodynamics and kinetics of radical reactions.40,50–55 The kinetic calculations were performed following the QM-ORSA protocol,40,43 following the literature.54–61 This method has been repeatedly benchmarked against experimental data, delivering results with low errors (kcalc/kexp ratio = 1–2.9), particularly in lipid and aqueous solutions.40,43,52,62 As a reference all details of the calculations are shown in Table S1, ESI.†
3. Results and discussion
3.1. Synthesis of polysubstituted 3-pyrroline-2-ones
The reaction between benzaldehyde (1a), aniline (2a) and sodium diethyl oxalacetate (3) in the presence of citric acid (2 equiv.) as the catalyst was used as the starting point to optimize the reaction conditions. Equimolar amounts of 1a, 2a and 3 at 0.5 M concentrations in absolute ethanol at room temperature turned over to 1,5-diphenyl-4-ethoxycarbonyl-3-hydroxy-3-pyrroline-2-one (4a) with 42% yield. This result is consistent with prior reports.29,30
There was a slight decrease in the yield of 4a (35%) when the concentration of starting materials in solvent was reduced to 0.34 M. The yield was only 37–38% when the concentration of 1a or 2a was increased to 0.75 M in absolute ethanol. However, there was a dramatic increase in the yield of 4a to 86% when 2 equiv of sodium diethyl oxalacetate (3) was used (Table 1). Therefore, the ratio 1
:
1
:
2 of reactants aromatic aldehyde, amine and sodium diethyl oxalacetate, respectively, was used to synthesize other polysubstituted 3-hydroxy-1,5-dihydro-2H-pyrrol-2-ones in absolute ethanol (1 mL) (Table 2).
Table 1 Starting materials 1a
:
2a
:
3 optimization for the synthesis of 4a
Entry |
Solvent volume (mL) |
Ratio 1a : 2a : 3 (equiv.) |
Concentration 1a : 2a : 3 (mmol mL−1) |
Yield (%) |
1 |
1.0 |
1 : 1 : 1 |
0.5 : 0.5 : 0.5 |
42 |
2 |
1.5 |
1 : 1 : 1 |
0.34 : 0.34 : 0.34 |
35 |
3 |
1.0 |
1.5 : 1 : 1 |
0.75 : 0.5 : 0.5 |
37 |
4 |
1.0 |
1 : 1.5 : 1 |
0.5 : 0.75 : 0.5 |
38 |
5 |
1.0 |
1 : 1 : 1.5 |
0.5 : 0.5 : 0.75 |
68 |
6 |
1.0 |
1 : 1 : 2 |
0.5 : 0.5 : 1.0 |
86 |
Table 2 Synthesis of polysubstituted 3-hydroxy-1,5-dihydro-2H-pyrrol-2-ones
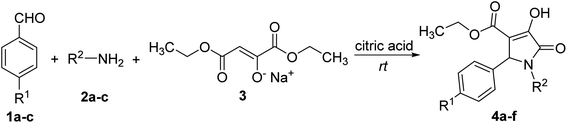
|
Entry |
R1 |
R2 |
Product |
Yield (%) |
1 |
H |
C6H5 |
4a
|
86 |
2 |
CH3 |
C6H5 |
4b
|
72 |
3 |
NO2 |
C6H5 |
4c
|
91 |
4 |
CH3 |
C6H5CH2 |
4d
|
55 |
5 |
NO2 |
C6H5CH2 |
4e
|
79 |
6 |
CH3 |
3-NO2C6H4 |
4f
|
70 |
The initial step in the multi-component reaction to synthesize polysubstituted 3-hydroxy-1,5-dihydro-2H-pyrrol-2-ones proceeds via acid-catalyzed condensation of aromatic aldehyde (1a–c) and amine (2a–c) in the presence of citric acid as the catalyst to yield imine (5) which will be then protonated to iminium (6).21,24 In the next step, sodium diethyl oxalacetate (3) affords the enol derivative (7) in an acidic environment, and subsequently, enol (7) will react with iminium species 6 to obtain intermediate 8 containing protonated ketone functional group. Then, the deprotonation of 8 will result in intermediate 9 containing enol moiety. The intramolecular nucleophilic attack of the secondary amino group present in intermediate 9 to the carbonyl carbon of a carboxylate moiety results in the formation of polysubstituted 3-hydroxy-1,5-dihydro-2H-pyrrol-2-ones (4a–f) (Scheme 1).19,21,24
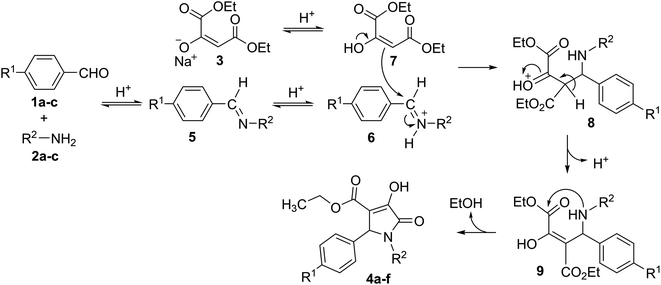 |
| Scheme 1 Mechanism for the synthesis of polysubstituted 3-hydroxy-1,5-dihydro-2H-pyrrol-2-ones.19 | |
The presence of methyl group in 4-methylbenzaldehyde (1b) will reduce the electrophilicity of carbonyl carbon via a positive inductive effect as compared to benzaldehyde. In contrast, the electron-withdrawing property of the nitro group in 4-nitrobenzaldehyde (1c) results in an increase in the electrophilicity of the carbonyl carbon.63 Consequently, the rate of imine formation reaction between 4-nitrobenzaldehyde (1c) and aniline (2a) is faster than that between 4-methylbenzaldehyde (1b) and aniline (2a).64 The easier the imine formation, the higher the yield of desired product polysubstituted 3-hydroxy-1,5-dihydro-2H-pyrrol-2-one. Moreover, the formation of the –CH
N– double bond will decrease when using aliphatic amines as compared to aromatic amines.65,66 Therefore, the yields of polysubstituted 3-hydroxy-1,5-dihydro-2H-pyrrol-2-ones 4b, 4c are higher than that of 4d, 4e, respectively.
In the structure of polysubstituted 3-hydroxy-1,5-dihydro-2H-pyrrol-2-ones, the –C(O)OCH2CH3 group is almost coplanar with the plane of the 3-pyrroline-2-one.21,22 As a consequence, two protons of methylene group are diastereotopic and they will show geminal coupling in 1H NMR. In addition, methylene protons (CH2) will also couple with protons of methyl group (CH3) separated by three sigma bonds. Therefore, two protons of methylene group should be represented by two doublet of quartet (dq) instead of a simple quartet. For instance, 1H NMR of 1-benzyl-4-ethoxycarbonyl-3-hydroxy-5-(4-nitrophenyl)-3-pyrroline-2-one (4e) showed two doublet of quartet at the chemical shift of 3.92 and 3.98 ppm representing for two methylene protons of –C(O)OCH2CH3 group. In addition, two protons of methylene group attached to nitrogen at 1-position are also diastereotopic and thus, represented by two doublets at the chemical shifts of 4.78 and 3.80 ppm.
3.2. Radical scavenging activity of 3-pyrroline-2-ones
3.2.1. DPPH antioxidant assay.
In the initial evaluation of the antioxidant activity of the synthetic 3-pyrroline-2-ones, the DPPH assay was used following the literature44,47,48 using quercetin as the reference antioxidant (Table 3). Under the tested conditions, the studied compound exhibited lower DPPH scavenging activity (EC50 > 128 µg mL−1, 0.33–0.39 mM) than quercetin (9.97 ± 0.25 µg mL−1, 0.033 mM). Among the synthetic compounds, the best DPPH antiradical activity was observed for 4b (40% DPPH at 128 µg mL−1), which was more than five times higher than that of 4d (7% DPPH) or 4f (6% DPPH). Activities of 4a, 4c and 4e were intermediate at 14, 32 and 26% DPPH, respectively. However, previous studies showed that the 3-pyrroline-2-one derivatives could exhibit potent radical scavenging activity in more biologically relevant settings (i.e., against HO˙ or lipid peroxidation)2,15–18 thus the HO˙ and HOO˙ radical scavenging activity of the most active compound (i.e., 4b) in physiological environments has been investigated further using computational chemistry.
Table 3 DPPH radical scavenging activity
Compound |
DPPH (%) |
EC50 (µg mL−1) |
128 (µg mL−1) |
32 (µg mL−1) |
8 (µg mL−1) |
4a
|
14 |
0 |
0 |
>128 |
4b
|
40 |
12 |
0 |
>128 |
4c
|
32 |
11 |
0 |
>128 |
4d
|
7 |
0 |
0 |
>128 |
4e
|
26 |
5 |
0 |
>128 |
4f
|
6 |
1 |
0 |
>128 |
Quercetin |
100 |
45.5 |
0 |
9.97 ± 0.25 |
3.2.2. Calculations of the HOO˙ and HO˙ radical scavenging activity of the most active compound (4b).
3.2.2.1 The antiradical activity in the gas phase.
The antioxidant properties of 4b were initially tested in the gas phase in order to identify the predominant antioxidant mechanism(s) for the more complex calculations in physiological environments. As previously demonstrated, this approach decreases computation time while giving accurate and reliable data.55 In the first step, the principal thermodynamic properties (proton affinity (PA), ionization energy (IE) and bond dissociation enthalpy (BDE)) that define the radical scavenging activity mechanisms (sequential proton loss electron transfer (SPLET), single electron transfer proton transfer (SETPT) and formal hydrogen transfer (FHT)),67,68 of 4b were computed (Table 4).
Table 4 The calculated BDEs, PAs and IEs (in kcal mol−1)a
Positions |
BDE |
PA |
IE |
4b numbering is shown in Fig. 3.
|
O3–H |
93.2 |
326.6 |
186.4 |
C5–H |
76.0 |
|
|
O7–H |
96.1 |
|
|
C8–H |
101.5 |
|
|
O21–H |
89.7 |
|
|
The lowest calculated BDE was observed at the C5–H bond at 76.0 kcal mol−1, whereas those of the other C(O)–H bonds were higher by about 13.7–25.5 kcal mol−1. Thus, data implies that the C5–H bond dominates the antioxidant activity of 4b following the FHT pathway. On the other hand, the radical scavenging activity of 4b according to either the SPLET or SETPT would be challenging due to the high PA and IE values (PA = 326.6 kcal mol−1 and IE = 186.4 kcal mol−1) in comparison to the BDEs.
The radical adduct formation (RAF) process also plays a key role in the radical scavenging of compounds with double bonds, particularly in the HO˙ antiradical action, as demonstrated by prior research62,69–71 and hence this pathway should be also examined. Gibbs free energy changes (ΔG°) for the HO˙ and HOO˙ radical scavenging reactions of 4b via the FHT, SET and RAF mechanisms were calculated in the gas phase and is presented in Table 5. With the exception of the SET reaction (ΔG° = 158.8 kcal mol−1), it was found that the HO˙ radical scavenging reactions are spontaneous (ΔG° < 0) for all positions in 4b; however, the HOO˙ radical scavenging is spontaneous only at the C5–H bond (ΔG° = −10.2 kcal mol−1) according to the FHT mechanism. Consistently all positions (ΔG° < 0, Table 5) were evaluated kinetically for the radical scavenging of 4b against the HO˙ radical in a vacuum, while only the H-abstraction of the C5–H bond was estimated for the HOO˙ radical. The results are presented in Table 6 and Fig. 2.
Table 5 The calculated ΔG° values (in kcal mol−1) of the reactions of 4b with HO˙ and HOO˙ following the FHT, SET and RAF mechanisms
Mechanisms |
Positions |
ΔG° |
HO˙ |
HOO˙ |
FHT |
O3–H |
−22.8 |
8.5 |
C5–H |
−41.5 |
−10.2 |
C7–H |
−21.0 |
10.2 |
C8–H |
−15.8 |
15.4 |
C21–H |
−25.8 |
5.4 |
RAF |
C3 |
−24.8 |
6.3 |
C4 |
−25.0 |
3.2 |
C9 |
−6.7 |
|
C10 |
−11.5 |
|
C11 |
−4.5 |
|
C12 |
−7.4 |
|
C13 |
−4.4 |
|
C14 |
−8.4 |
|
C15 |
−8.6 |
|
C16 |
−6.2 |
|
C17 |
−6.3 |
|
C18 |
−9.3 |
|
C19 |
−7.1 |
|
C20 |
−5.1 |
|
SET |
|
158.8 |
163.5 |
Table 6 Calculated activation energies (ΔG≠ (kcal mol−1)), tunneling corrections (κ) and kEck, koverall (M−1 s−1) and branching ratios (Γ, %) at 298.15 K for the HO˙ and HOO˙ scavenging of 4b
Mechanisms |
Positions |
HO˙ |
HOO˙ |
ΔG≠ |
κ
|
k
Eck
|
Γ
|
ΔG≠ |
κ
|
k
Eck
|
FHT |
O3–H |
10.7 |
21.3 |
1.93 × 106 |
0.0 |
|
|
|
C5–H |
3.4 |
1.1 |
2.19 × 1010 |
37.9 |
18.0 |
43.8 |
5.48 × 101 |
C7–H |
5.0 |
1.0 |
1.39 × 109 |
2.4 |
|
|
|
C8–H |
9.4 |
2.7 |
2.11 × 106 |
0.0 |
|
|
|
C21–H |
6.8 |
1.1 |
7.23 × 107 |
0.1 |
|
|
|
RAF |
C3 |
4.8 |
1.0 |
1.93 × 109 |
3.3 |
|
|
|
C4 |
3.2 |
1.1 |
3.18 × 1010 |
55.1 |
|
|
|
C9 |
10.2 |
1.3 |
2.89 × 105 |
0.0 |
|
|
|
C10 |
5.5 |
1.1 |
6.43 × 108 |
1.1 |
|
|
|
C11 |
9.2 |
1.3 |
1.51 × 106 |
0.0 |
|
|
|
C12 |
7.9 |
1.2 |
1.26 × 107 |
0.0 |
|
|
|
C13 |
9.8 |
1.3 |
5.36 × 105 |
0.0 |
|
|
|
C14 |
7.9 |
1.1 |
1.20 × 107 |
0.0 |
|
|
|
C15 |
9.7 |
1.1 |
5.06 × 105 |
0.0 |
|
|
|
C16 |
7.4 |
1.1 |
5.06 × 105 |
0.0 |
|
|
|
C17 |
7.6 |
1.2 |
5.06 × 105 |
0.0 |
|
|
|
C18 |
8.0 |
1.0 |
5.06 × 105 |
0.0 |
|
|
|
C19 |
6.8 |
1.1 |
5.06 × 105 |
0.0 |
|
|
|
C20 |
7.4 |
1.2 |
5.06 × 105 |
0.0 |
|
|
|
k
overall
|
5.77 × 10
10
|
|
|
|
5.48 × 10
1
|
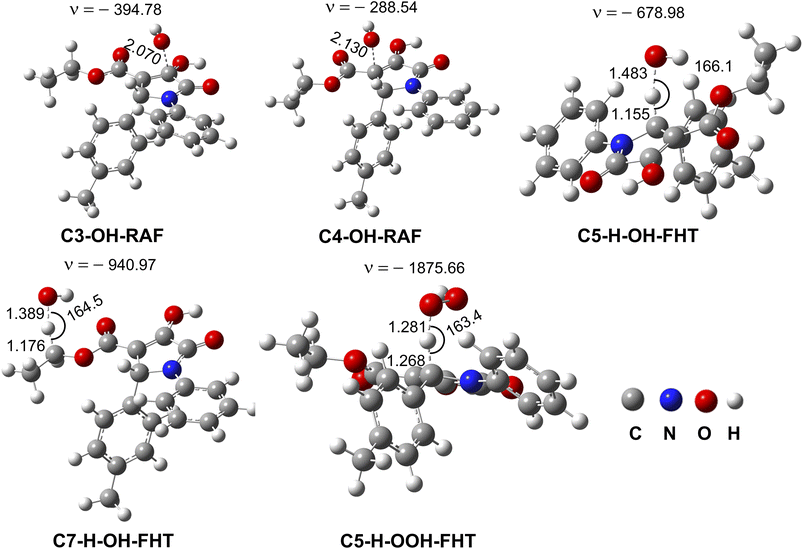 |
| Fig. 2 Optimized geometries of the main transition states between 4b and HO˙/HOO˙ radicals according to FHT and RAF processes. | |
The overall rate constant (koverall) for HO˙ radical scavenging in the gas phase was 5.77 × 1010 M−1 s−1, however only 5.48 × 101 M−1 s−1 for HOO˙ antiradical activity (Table 6). The hydroxyl antiradical activity was defined by a combination of the RAF mechanism (at the C3 (Γ = 3.3%) and C4 (Γ = 55.1%)) and the FHT mechanism (at the C5–H (Γ = 37.9%) and C7–H (Γ = 2.4%)). The H-abstraction of the C5–H bond determined the HOO˙ antiradical activity while contributing 37.9% to the total HO˙ radical scavenging. Thus these reactions should be used for further kinetic evaluation in the physiological environments.
3.2.2.2. The antiradical activity in the physiological environments.
3.2.2.2.1. Acid base equilibrium.
In order to account for the influence of physiological settings, the radical scavenging of 4b against HO˙ and HOO˙ radicals was simulated in water at pH = 7.4 for the aqueous solution and in pentyl ethanoate for lipid medium.40,55 Following the literature,72,73 the acid-base equilibria of 4b at the O3–H bond were computed to estimate the state of 4b in aqueous solution at pH = 7.4 (Fig. 3). The pKa value was 5.40. Fig. 3 shows that 4b consistently exists both in the neutral state (HA, 1.0%) and the monoanion state (A−, 99.0%) at physiological pH (7.40). These states are employed for further investigation in the polar medium.
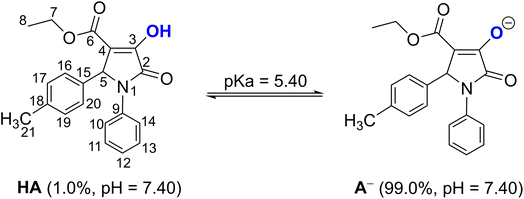 |
| Fig. 3 The deprotonation of 4b at pH = 7.40. | |
The QM-ORSA protocol was used to evaluate the kinetics of the HO˙ and HOO˙ scavenging reactions in physiological environments.40 The results are shown in Table 7. The results showed that in pentyl ethanoate and water solvents, the koverall values for the 4b + HO˙ + reaction were 2.05 × 109 and 1.54 × 1010 M−1 s−1, respectively, while those were 6.90 × 10−1 and 1.82 × 103 M−1 s−1 for the 4b + HOO˙ reaction. The HO˙ antiradical activity in lipid medium was defined by the RAF mechanism at the C4 position (Γ = 57.1%) and the FHT pathway at C5–H bond (Γ = 30.6%), whereas that for the aqueous solution was characterized by the SET reaction of the anion state (Γ = 56.7%). The RAF and FHT reactions of the anion state contributed more than 40% in the hydroxyl antiradical activity in water at pH = 7.40, however these reactions of the neutral state had no contribution to the activity. At the same time, the FHT (C5–H, Γ = 59.7%) and RAF (C4, Γ = 40.2%) reactions of the anion state played a decisive role in the HOO˙ antiradical activity of 4b in water at pH = 7.40. The ability of 4b to scavenge hydroxyl radical in polar and non-polar environments is comparable to that of common antioxidants such melatonin,74 gallic acid,75 indole-3-carbinol,71 ramalin,70 and Trolox.40 However, 4b exhibited low hydroperoxyl radical scavenging activity in both the lipid and polar media. Thus 4b is a good but not exceptional hydroxyl radical scavenger in the physiological environment with targeted activities that may invite further studies into its activity against specific compounds.
Table 7 Gibbs free energies of activation (ΔG≠, kcal mol−1), rate constants (kapp, kf, M−1 s−1) and branching ratios (Γ, %) at 298.15 K, in the NPY oxidation by HO˙/HOO˙ radicals in the studied environments
States |
Mechanisms |
Pentyl ethanoate |
Water |
ΔG≠ |
k
app
|
Γ
|
ΔG≠ |
k
app
|
f
|
k
f
|
Γ
|
HO˙ |
HA |
SET |
|
|
|
22.8 |
1.20 × 10−4 |
0.01 |
1.20 × 10−6 |
0.0 |
|
|
FHT |
C5–H |
5.5 |
6.27 × 108 |
30.6 |
5.6 |
6.76 × 108 |
0.01 |
6.76 × 106 |
0.0 |
|
|
|
C7–H |
6.6 |
1.19 × 108 |
5.8 |
8.2 |
8.10 × 106 |
0.01 |
8.10 × 104 |
0.0 |
|
|
RAF |
C3 |
6.5 |
1.32 × 108 |
6.4 |
6.3 |
1.95 × 108 |
0.01 |
1.95 × 106 |
0.0 |
|
|
|
C4 |
5.2 |
1.17 × 109 |
57.1 |
4.7 |
3.08 × 109 |
0.01 |
3.08 × 107 |
0.2 |
|
A− |
SET |
|
|
|
|
0.7 |
8.80 × 109 |
0.99 |
8.71 × 109 |
56.7 |
|
|
FHT |
C5–H |
|
|
|
3.8 |
2.38 × 109 |
0.99 |
2.35 × 109 |
15.3 |
|
|
|
C7–H |
|
|
|
6.1 |
2.13 × 108 |
0.99 |
2.11 × 108 |
1.4 |
|
|
RAF |
C3 |
|
|
|
∼0 |
2.10 × 109 |
0.99 |
2.08 × 109 |
13.5 |
|
|
|
C4 |
|
|
|
∼0 |
2.00 × 109 |
0.99 |
1.98 × 109 |
12.9 |
|
k
overall
|
|
2.05 × 10
9
|
|
|
1.54 × 1010 |
|
HOO˙ |
HA |
FHT |
C5–H |
20.6 |
6.90 × 10−1 |
100.0 |
19.9 |
3.10 |
0.01 |
3.10 × 10−2 |
0.0 |
|
A− |
SET |
|
|
|
|
19.3 |
4.80 × 10−2 |
0.99 |
4.75 × 10−2 |
0.0 |
|
|
FHT |
C5–H |
|
|
|
15.0 |
1.10 × 103 |
0.99 |
1.09 × 103 |
59.7 |
|
|
RAF |
C3 |
|
|
|
17.5 |
1.10 |
0.99 |
1.09 |
0.1 |
|
|
|
C4 |
|
|
|
13.6 |
7.40 × 102 |
0.99 |
7.33 × 102 |
40.2 |
|
k
overall
|
6.90 × 10
−1
|
|
|
1.82 × 103 |
|
4. Conclusion
Six polysubstituted 3-hydroxy-3-pyrroline-2-ones were successfully synthesized by using three-component reactions at room temperature. The DPPH assay indicated that 4b exhibits the highest DPPH radical scavenging activity. The thermodynamic and kinetic calculations also showed that 4b is a potent HO˙ radical scavenger with koverall = 2.05 × 109 and 1.54 × 1010 M−1 s−1 in pentyl ethanoate and water, respectively. However, 4b exhibited only low hydroperoxyl radical scavenging activity in either of the lipid and polar media. Compared to typical antioxidants such as melatonin, gallic acid, indole-3-carbinol, ramalin, and Trolox, the ability of 4b to scavenge hydroxyl radicals in polar and non-polar environments is similar to that of these compounds. Thus 4b is a promising HO˙ radical scavenger in physiological environments.
Conflicts of interest
There are no conflicts to declare.
Acknowledgements
This research is funded by the Vietnamese Ministry of Education and Training under project number B2021-DNA-17 (N.T.N.).
References
- S. B. Singh, D. L. Zink, M. A. Goetz, A. W. Dombrowski, J. D. Polishook and D. J. Hazuda, Tetrahedron Lett., 1998, 39, 2243–2246 CrossRef CAS.
- J. Whitt, S. M. Shipley, D. J. Newman and K. M. Zuck, J. Nat. Prod., 2014, 77, 173–177 CrossRef CAS PubMed.
- X. Del Corte, A. López-Francés, A. Maestro, I. Villate-Beitia, M. Sainz-Ramos, E. Martínez de Marigorta, J. L. Pedraz, F. Palacios and J. Vicario, Pharmaceuticals, 2021, 14, 782 CrossRef CAS PubMed.
- E. Surmiak, A. Twarda-Clapa, K. M. Zak, B. Musielak, M. D. Tomala, K. Kubica, P. Grudnik, M. Madej, M. Jablonski and J. Potempa, ACS Chem. Biol., 2016, 11, 3310–3318 CrossRef CAS PubMed.
- N. Joksimović, J. Petronijević, N. Janković, D. Baskić, S. Popović, D. Todorović, S. Matić, G. A. Bogdanović, M. Vraneš and A. Tot, Bioorg. Chem., 2019, 88, 102954 CrossRef PubMed.
- J. Kuznecovs, M. Vorona, I. Domraceva, I. Kanepe-Lapsa, M. Petrova, E. Liepins, S. Belyakov, A. Leonchiks and G. Veinberg, Chem. Heterocycl. Compd., 2018, 54, 514–519 CrossRef CAS.
- J. Li, Y. Wu, Z. Guo, C. Zhuang, J. Yao, G. Dong, Z. Yu, X. Min, S. Wang and Y. Liu, Bioorg. Med. Chem. Lett., 2014, 24, 2648–2650 CrossRef CAS PubMed.
- V. Gein, V. Mihalev, N. Kasimova, E. Voronina, M. Vakhrin and E. Babushkina, Pharm. Chem. J., 2007, 41, 208–210 CrossRef CAS.
- A. Q. Cusumano and J. G. Pierce, Bioorg. Med. Chem. Lett., 2018, 28, 2732–2735 CrossRef CAS PubMed.
- A. López-Pérez, S. Freischem, I. Grimm, O. Weiergräber, A. J. Dingley, M. P. López-Alberca, H. Waldmann, W. Vollmer, K. Kumar and C. Vuong, Antibiotics, 2021, 10, 529 CrossRef PubMed.
- K. Ma, P. Wang, W. Fu, X. Wan, L. Zhou, Y. Chu and D. Ye, Bioorg. Med. Chem. Lett., 2011, 21, 6724–6727 CrossRef CAS PubMed.
- V. Gein, V. Yushkov, N. Kasimova, N. Shuklina, M. Y. Vasil'eva and M. Gubanova, Pharm. Chem. J., 2005, 39, 484–487 CrossRef CAS.
- N. V. Ortiz Zacarías, J. P. van Veldhoven, L. Portner, E. van Spronsen, S. Ullo, M. Veenhuizen, W. J. van der Velden, A. J. Zweemer, R. M. Kreekel and K. Oenema, J. Med. Chem., 2018, 61, 9146–9161 CrossRef PubMed.
- S. Zykova, M. Danchuk, V. Talismanov, N. Tokareva, N. Igidov, I. Rodin, A. Koshchaev, N. Gugushvili and O. Karmanova, J. Pharm. Sci. Res., 2018, 10, 164–166 CAS.
- X. Liu, L. Zang, C. J. Van der Schyf, K. Igarashi, K. Castagnoli and N. Castagnoli, Chem. Res. Toxicol., 1999, 12, 508–512 Search PubMed.
- W.-H. Zhang, J. Liu, G. Xu, Q. Yuan and L. M. Sayre, Chem. Res. Toxicol., 2003, 16, 512–523 Search PubMed.
- K. Itakura, T. Osawa and K. Uchida, J. Org. Chem., 1998, 63, 185–187 CrossRef CAS PubMed.
- L. Tsai, P. A. Szweda, O. Vinogradova and L. I. Szweda, Proc. Natl. Acad. Sci. U. S. A., 1998, 95, 7975–7980 CrossRef CAS PubMed.
- E. M. de Marigorta, M. Jesús, A. M. O. de Retana, J. Vicario and F. Palacios, Synthesis, 2018, 50, 4539–4554 CrossRef.
-
J. Zhu, Q. Wang and M. Wang, Multicomponent reactions in organic synthesis, John Wiley & Sons, 2014 Search PubMed.
- H. Ahankar, A. Ramazani, K. Ślepokura, T. Lis and S. W. Joo, Green Chem., 2016, 18, 3582–3593 RSC.
- Z. Hosseinzadeh, A. Ramazani, H. Ahankar, K. Ślepokura and T. Lis, Silicon, 2019, 11, 2933–2943 CrossRef CAS.
- R. Ghorbani‐Vaghei, N. Sarmast and J. Mahmoodi, Appl. Organomet. Chem., 2017, 31, e3681 CrossRef.
- M. Saha and A. R. Das, ChemistrySelect, 2017, 2, 10249–10260 CrossRef CAS.
- R. Sarkar and C. Mukhopadhyay, Tetrahedron Lett., 2013, 54, 3706–3711 CrossRef CAS.
- S. Esmaeilzadeh and D. Setamdideh, J. Serbian Chem. Soc., 2021, 59 Search PubMed.
- A. Dutta, M. A. Rohman, R. Nongrum, A. Thongni, S. Mitra and R. Nongkhlaw, New J. Chem., 2021, 45, 8136–8148 RSC.
- B. Metten, M. Kostermans, G. Van Baelen, M. Smet and W. Dehaen, Tetrahedron, 2006, 62, 6018–6028 CrossRef CAS.
- M. Mohammat, Z. Shaameri and A. Hamzah, Molecules, 2009, 14, 250–256 CrossRef CAS PubMed.
- S. Manta, D.-N. Gkaragkouni, E. Kaffesaki, P. Gkizis, D. Hadjipavlou-Litina, E. Pontiki, J. Balzarini, W. Dehaen and D. Komiotis, Tetrahedron Lett., 2014, 55, 1873–1876 CrossRef CAS.
-
A. V. Dolzhenko and A. V. Dolzhenko, in Green synthetic approaches for biologically relevant heterocycles, Elsevier, 2015, pp. 101–139 Search PubMed.
- L. M. Sayre, G. Perry and M. A. Smith, Chem. Res. Toxicol., 2008, 21, 172–188 Search PubMed.
- W. A. Pryor, Free Radicals Biol. Med., 1988, 4, 219–223 CrossRef CAS PubMed.
- R. J. Reiter, D.-X. Tan, T. S. Herman and C. R. Thomas Jr, Int. J. Radiat. Oncol., Biol., Phys., 2004, 59, 639–653 CrossRef PubMed.
- L. P. Candeias and S. Steenken, Chem. Eur. J., 2000, 6, 475–484 CrossRef CAS PubMed.
- C. Chatgilialoglu, M. D’Angelantonio, M. Guerra, P. Kaloudis and Q. G. Mulazzani, Angew. Chem., Int. Ed. Engl., 2009, 48, 2214–2217 CrossRef CAS PubMed.
- A. Galano and J. R. Alvarez-Idaboy, Org. Lett., 2009, 11, 5114–5117 CrossRef CAS PubMed.
- M. Leopoldini, N. Russo and M. Toscano, Food Chem., 2011, 125, 288–306 CrossRef CAS.
- J. S. Wright, E. R. Johnson and G. A. DiLabio, J. Am. Chem. Soc., 2001, 123, 1173–1183 CrossRef CAS PubMed.
- A. Galano and J. R. Alvarez‐Idaboy, J. Comput. Chem., 2013, 34, 2430–2445 CrossRef CAS PubMed.
- A. Galano, G. Mazzone, R. Alvarez-Diduk, T. Marino, J. R. Alvarez-Idaboy and N. Russo, Annu. Rev. Food Sci. Technol., 2016, 7, 335–352 CrossRef CAS PubMed.
- M. Leopoldini, N. Russo, S. Chiodo and M. Toscano, J. Agric. Food Chem., 2006, 54, 6343–6351 CrossRef CAS PubMed.
- A. Galano and J. Raúl Alvarez-Idaboy, Int. J. Quantum Chem., 2019, 119, e25665 CrossRef.
- M. Cuendet, K. Hostettmann, O. Potterat and W. Dyatmiko, Helv. Chim. Acta, 1997, 80, 1144–1152 CrossRef CAS.
- A. I. Elshamy, T. Yoneyama, N. Van Trang, N. T. Son, Y. Okamoto, S. Ban, M. Noji and A. Umeyama, J. Mol. Struct., 2020, 1200, 127061 CrossRef CAS.
- L. T. T. Anh, N. Van Tuyen, P. T. Thuy, P. M. Quan, N. T. T. Ha and N. T. Tra, Mol. Divers., 2022, 26, 229–243 CrossRef CAS PubMed.
- K. Marxen, K. H. Vanselow, S. Lippemeier, R. Hintze, A. Ruser and U.-P. Hansen, Sensors, 2007, 7, 2080–2095 CrossRef CAS PubMed.
- M. Burits and F. Bucar, Phytother. Res., 2000, 14, 323–328 CrossRef CAS PubMed.
-
M. J. Frisch, G. W. Trucks, H. B. Schlegel, G. E. Scuseria, M. A. Robb, J. R. Cheeseman, G. Scalmani, V. Barone, B. Mennucci, G. A. Petersson, H. Nakatsuji, M. Caricato, X. Li, A. F. I. H. P. Hratchian, J. Bloino, G. Zheng, M. H. J. L. Sonnenberg, M. Ehara, K. Toyota, J. H. R. Fukuda, M. Ishida, T. Nakajima, Y. Honda, H. N. O. Kitao, T. Vreven, J. A. Montgomery Jr, F. O. J. E. Peralta, M. J. Bearpark, J. Heyd, K. N. K. E. N. Brothers, V. N. Staroverov, R. Kobayashi, K. R. J. Normand, A. P. Rendell, J. C. Burant, J. T. S. S. Iyengar, M. Cossi, N. Rega, N. J. Millam, J. E. K. M. Klene, J. B. Cross, V. Bakken, C. Adamo, R. G. J. Jaramillo, R. E. Stratmann, O. Yazyev, R. C. A. J. Austin, C. Pomelli, J. W. Ochterski, K. M. R. L. Martin, V. G. Zakrzewski, G. A. Voth, J. J. D. P. Salvador, S. Dapprich, A. D. Daniels, J. B. F. Ö. Farkas, J. V. Ortiz, J. Cioslowski, and D. J. Fox, Gaussian 09, Gaussian, Inc., Wallingford CT, 2009 Search PubMed.
- Y. Zhao and D. G. Truhlar, J. Phys. Chem. A, 2008, 112, 1095–1099 CrossRef CAS PubMed.
- A. Galano and J. R. Alvarez-Idaboy, J. Comput. Chem., 2014, 35, 2019–2026 CrossRef CAS PubMed.
- J. R. l. Alvarez-Idaboy and A. Galano, J. Phys. Chem. B, 2012, 116, 9316–9325 CrossRef CAS PubMed.
- M. E. Alberto, N. Russo, A. Grand and A. Galano, Phys. Chem. Chem. Phys., 2013, 15, 4642–4650 RSC.
- E. Dzib, J. L. Cabellos, F. Ortíz‐Chi, S. Pan, A. Galano and G. Merino, Int. J. Quantum Chem., 2019, 119, e25686 CrossRef.
- Q. V. Vo, M. V. Bay, P. C. Nam, D. T. Quang, M. Flavel, N. T. Hoa and A. Mechler, J. Org. Chem., 2020, 85, 15514–15520 CrossRef CAS PubMed.
- M. G. Evans and M. Polanyi, Trans. Faraday Soc., 1935, 31, 875–894 RSC.
- H. Eyring, J. Chem. Phys., 1935, 3, 107–115 CrossRef.
- D. G. Truhlar, W. L. Hase and J. T. Hynes, J. Phys. Chem., 1983, 87, 2664–2682 CrossRef CAS.
- T. Furuncuoglu, I. Ugur, I. Degirmenci and V. Aviyente, Macromolecules, 2010, 43, 1823–1835 CrossRef CAS.
- E. Vélez, J. Quijano, R. Notario, E. Pabón, J. Murillo, J. Leal, E. Zapata and G. Alarcón, J. Phys. Org. Chem., 2009, 22, 971–977 CrossRef.
-
E. Dzib, J. L. Cabellos, F. Ortiz-Chi, S. Pan, A. Galano and G. Merino, Eyringpy 1.0.2, Cinvestav Mérida, Yucatán, 2018 Search PubMed.
- Q. V. Vo, M. V. Bay, P. C. Nam and A. Mechler, J. Phys. Chem. B, 2019, 123, 7777–7784 CrossRef CAS PubMed.
- A. K. Chakraborti, S. Bhagat and S. Rudrawar, Tetrahedron Lett., 2004, 45, 7641–7644 CrossRef CAS.
- B. Mostafa, S. M. Habibi‐Khorassani and M. Shahraki, J. Phys. Org. Chem., 2017, 30, e3616 CrossRef.
- A. Simion, C. Simion, T. Kanda, S. Nagashima, Y. Mitoma, T. Yamada, K. Mimura and M. Tashiro, J. Chem. Soc., Perkin Trans. 1, 2001, 2071–2078 RSC.
-
J. Clayden, N. Greeves and S. Warren, Organic chemistry, Oxford university press, 2012 Search PubMed.
- A. Galano, J. Mex. Chem. Soc., 2015, 59, 231–262 Search PubMed.
- Y.-Z. Zheng, G. Deng, Q. Liang, D.-F. Chen, R. Guo and R.-C. Lai, Sci. Rep., 2017, 7, 1–11 CrossRef PubMed.
- C. Iuga, J. R. Alvarez-Idaboy and A. Vivier-Bunge, J. Phys. Chem. B, 2011, 115, 12234–12246 CrossRef CAS PubMed.
- Q. V. Vo, N. M. Tam, M. Van Bay and A. Mechler, Chem. Phys. Lett., 2020, 739, 137004 CrossRef CAS.
- Q. V. Vo, M. Van Bay, P. C. Nam and A. Mechler, ACS Omega, 2019, 4, 19375–19381 CrossRef CAS PubMed.
- A. Galano, A. Pérez-González, R. Castañeda-Arriaga, L. Muñoz-Rugeles, G. Mendoza-Sarmiento, A. Romero-Silva, A. Ibarra-Escutia, A. M. Rebollar-Zepeda, J. R. León-Carmona and M. A. Hernández-Olivares, J. Chem. Inf. Model., 2016, 56, 1714–1724 CrossRef CAS PubMed.
- Q. V. Vo, N. M. Tam, M. Van Bay, N. M. Thong, T. Le Huyen, N. T. Hoa and A. Mechler, RSC Adv., 2020, 10, 14937–14943 RSC.
- A. Galano, Phys. Chem. Chem. Phys., 2011, 13, 7178–7188 RSC.
- T. Marino, A. Galano and N. Russo, J. Phys. Chem. B, 2014, 118, 10380–10389 CrossRef CAS PubMed.
|
This journal is © The Royal Society of Chemistry 2022 |
Click here to see how this site uses Cookies. View our privacy policy here.