DOI:
10.1039/D2RA04977E
(Paper)
RSC Adv., 2022,
12, 26782-26788
Nano/micrometer porous conductive network structure Li4Ti5O12@C/CNT microspheres with enhanced sodium-storage capability as an anode material†
Received
9th August 2022
, Accepted 5th September 2022
First published on 21st September 2022
Abstract
Li4Ti5O12@C/CNT microspheres, wherein CNTs were firmly anchored to Li4Ti5O12@C nanoparticles, were prepared via a facile spray drying method and subsequently annealed in an argon atmosphere, exhibiting long cycling stability (charge/discharge capacities of 85.45/86.18 mA h g−1 after 500 cycles at 500 mA g−1) and excellent rate capability (charge capacity of 61.16 mA h g−1 after 10 cycles at 1000 mA g−1). The special spherical structure design is not only beneficial to improving the structural stability and reaction kinetics of the electrode materials during the long-term extraction–insertion of sodium ions but also supplies numerous interfacial sites to store more sodium ions.
1. Introduction
Li4Ti5O12, with a spinel structure, is a promising anode material of lithium-ion batteries because of its excellent cycling stability, stable discharge voltage platform, and high lithium-ion diffusion coefficient.1,2 However, its further development is severely restricted by its low theoretical specific capacity and poor electronic conductivity.3–5 In addition, flatulence occurs due to the side reactions between electrolyte and Li4Ti5O12, leading to rapid capacity fading and serious security threats.6,7 Research shows that the capacity can be improved by reducing the particle size,8 and a high rate capability can be realized by coating with a carbon layer9 or compositing with CNTs10,11 with good conductivity. Carbon layers coated on the surface of Li4Ti5O12 particles can prevent direct contact between the electrolyte and Li4Ti5O12, preventing the appearance of flatulence. Although the reversible capacity and conductivity of Li4Ti5O12 have been greatly improved, the healthy development of lithium-ion batteries is strictly limited by the lack of lithium resources.
Sodium-ion batteries have been regarded as an ideal alternative to lithium-ion batteries due to the abundance of sodium resources and their low cost, and have been widely studied in recent years.12–18 Similar to lithium-ion batteries, CNTs and carbon layers can be used to enhance the sodium-storage capability of Li4Ti5O12. A free-standing LTO-C/RGO electrode was prepared by modified vacuum filtration, which exhibited superior rate capability (98.7 mA h g−1 at 5C) and long cycling performance (114 mA h g−1 at 2C after 600 cycles).19 Wang et al. reported free-standing CNT/Li4Ti5O12/C composite nanofibers, achieving a high reversible capacity (119 mA h g−1 after 100 cycles at 100 mA g−1) and excellent rate capability (77 mA h g−1 at 50 0 mA h g−1).20 Although the rate capability and cycling stability have been greatly improved via various effective methods, the yield of the product is too low to satisfy the practical application of sodium-ion batteries. Thus, it is particularly important to find a method for large-scale production. Spray drying is a method for large-scale production that is widely used in the field of lithium-ion batteries.21–28 However, its application in the field of sodium-ion batteries is rarely reported. A Li4Ti5O12@C/rGO electrode was fabricated via the spray drying method, which shows superior sodium storage performance. A capacity retention of 95% is maintained after 1000 cycles at 5C at room temperature. Even when the ambient temperature increases to 60 °C, the capacity retention is still as high as 95% after 500 cycles at 10C.29
Hence, nano/micrometer porous structure Li4Ti5O12@C/CNT microspheres were prepared via a facile spray drying method and subsequently annealed in an argon atmosphere in this work. As a comparison, Li4Ti5O12 hollow nanospheres were synthesized by the solvothermal method to identify the superiority of the Li4Ti5O12@C/CNT microsphere structure. The results of electrochemical performance indicate that the special nano/micrometer porous structure of the Li4Ti5O12@C/CNT microspheres is crucial to improving their sodium-storage capability. Compared with the Li4Ti5O12 hollow nanospheres, the Li4Ti5O12@C/CNT microspheres show superior rate capability and longer cycling life. Thus, the Li4Ti5O12@C/CNT microspheres are a promising anode material for sodium-ion batteries.
2. Experimental section
2.1 Chemicals
Tetrabutyl titanate (C16H36O4Ti), lithium acetate (CH3COOLi), titanium dioxide (TiO2), absolute ethanol, and sucrose (C12H22O11) were all purchased from Sinopharm Chemical Reagent Co., Ltd. Carbon nanotubes (CNTs, 95%) were purchased from Chengdu Organic Chemicals Co., Ltd. All the chemical reagents were used without further purification. Deionized water from a Milli-Q system (Millipore, Bedford, MA) was used in all the experiments.
2.2 Fabrication of the Li4Ti5O12@C/CNT microspheres
The synthetic process of the Li4Ti5O12@C/CNT microspheres is shown in Fig. 1. Firstly, CNTs and deionized water were mixed and stirred for 2 h to obtain a well dispersed CNT dispersion, and sucrose was dissolved in deionized water to prepare a sucrose solution. CH3COOLi, TiO2, sucrose solution, and the CNT dispersion were mixed in a suspension. Then, the suspension was sprayed onto a Li4Ti5O12@C/CNT microsphere precursor using a spray dryer. Finally, the Li4Ti5O12@C/CNT microsphere precursor was annealed under an argon atmosphere at 800 °C for 10 h to synthesize the Li4Ti5O12@C/CNT microspheres.
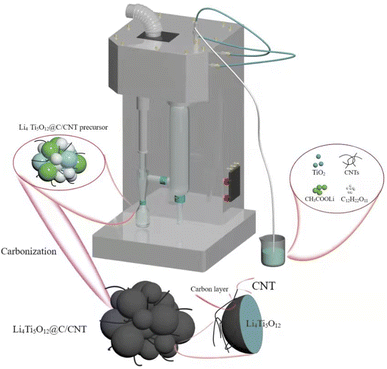 |
| Fig. 1 The synthetic process of the Li4Ti5O12@C/CNT microspheres. | |
2.3 Fabrication of the Li4Ti5O12 hollow nanospheres
The synthetic process of the Li4Ti5O12 hollow nanospheres is shown in Fig. S1,† which is mainly composed of SiO2 nanospheres, the SiO2@Li4Ti5O12 nanosphere precursor, and Li4Ti5O12 hollow nanospheres.
2.3.1 Fabrication of the SiO2 nanospheres. Firstly, 32 mL absolute ethanol and 1.5 mL deionized water were mixed and stirred for 30 min to obtain a well dispersed ethanol solution. Then, 35 mL concentrated ammonia was mixed with ethanol solution and stirred for 1 h to prepare a mixed solution. Finally, 3 mL tetraethyl orthosilicate was added to the mixed solution and stirred for 5 h. SiO2 nanospheres were synthesized via centrifugation and dried at 80 °C for 10 h.
2.3.2 Fabrication of the SiO2@Li4Ti5O12 nanosphere precursor. Firstly, 0.6 g SiO2 nanospheres were dispersed in 100 mL absolute ethanol and stirred for 2 h to prepare a homogeneous suspension. Secondly, a 20 mL mixed solution containing 0.132 g lithium acetate and 0.850 g tetrabutyl titanate was poured into the suspension and stirred for 2 h at room temperature to obtain a mixed solution. Thirdly, the mixed solution was poured into a hydrothermal autoclave and maintained at 200 °C for 36 h. Finally, the SiO2@Li4Ti5O12 nanosphere precursor powder was fabricated via centrifugation and subsequently dried at 80 °C for 12 h.
2.3.3 Fabrication of the Li4Ti5O12 hollow nanospheres. Firstly, the synthesized SiO2@Li4Ti5O12 nanosphere precursor powder was dispersed in a 100 mL 0.5 M KOH solution and stirred for 12 h in a water bath at 60 °C. Then, the product was washed by ultrasonication 3 times, and subsequently dried in a vacuum at 80 °C for 10 h. Finally, the dried powder was annealed under an air atmosphere at 800 °C for 12 h to prepare the Li4Ti5O12 hollow nanospheres.
2.4 Material characterization
The crystal structures were examined by X-ray diffraction (XRD, Bruker D8 Advance). The microstructures of the materials were analyzed by scanning electron microscopy (S-3400N) and transmission electron microscopy (JEM2100). The Raman spectra were recorded on a Raman spectrometer (LabRAM HR). The weight loss of the materials was detected by thermogravimetric analysis (Diamond TG/DTA). The N2 adsorption–desorption isotherms were plotted to analyze the specific surface area (BELSORP-miniII).
2.5 Sodium-ion battery electrochemical measurements
Firstly, the prepared powder, Super P, and sodium carboxymethyl cellulose (weight ratio: 7
:
1
:
2) were mixed to prepare a slurry. Then, the slurry was uniformly coated on copper foil and dried under vacuum at 80 °C for 12 h. Thirdly, coin cells (CR2016) were assembled in a glovebox filled with argon. Sodium metal foil and glass fiber were used as the counter electrode and separator, respectively. NaClO4 was mixed with ethylene carbonate (EC) and diethyl carbonate (DEC) (1
:
1 v/v) to prepare the electrolyte. The charge/discharge, rate capability, and cycling performance were tested using a Land Battery instrument. Cyclic voltammetry and electrochemical impedance spectroscopy were performed on a electrochemical workstation (CHI604D).
3. Results and discussion
The phases of the Li4Ti5O12 nanospheres and Li4Ti5O12@C/CNT microspheres are analyzed by X-ray diffraction to estimate the purity of the phase (Fig. 2). The diffraction peaks of the two materials are essentially similar, exhibiting that the crystal structure of Li4Ti5O12 has not been changed by the coated carbon layers and composite CNTs. The pure Li4Ti5O12 phases are detected and other phases are not found, showing that the Li4Ti5O12 nanospheres and Li4Ti5O12@C/CNT microspheres are synthesized successfully.
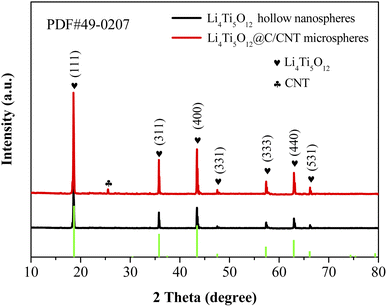 |
| Fig. 2 XRD patterns of the Li4Ti5O12@C/CNT microspheres and Li4Ti5O12 hollow nanospheres. | |
Fig. 3 shows the microstructures of the Li4Ti5O12 hollow nanospheres and Li4Ti5O12@C/CNT microspheres. The SiO2 nanospheres (Fig. S2a†) with a diameter of about 200 nm show good dispersion, which is conducive to the coating of the Li4Ti5O12 shell. Li4Ti5O12 was successfully coated on the surface of the SiO2 nanospheres, and SiO2@Li4Ti5O12 core–shell nanospheres were successfully prepared (Fig. S2b†). The size of the Li4Ti5O12 hollow nanospheres ranges from 50 nm to 300 nm (Fig. 3a), which is beneficial to the size effect. The microspheres with a diameter of 1–10 μm exhibit good dispersion. The outer surface structure of the microspheres consists of Li4Ti5O12 nanoparticles and CNTs with a diameter of 50 nm (Fig. S3a†), and a network structure was formed by interweaving between the Li4Ti5O12 nanoparticles and CNTs (Fig. 3b and S3b†). In addition, numerous nanometer and micrometer-scale pores were produced due to the interweaving action.
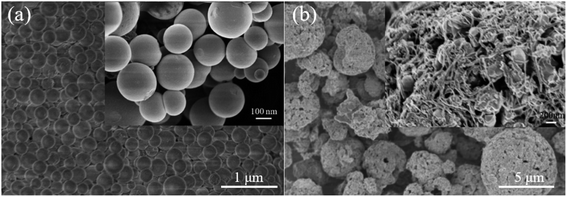 |
| Fig. 3 SEM images of (a) the Li4Ti5O12 hollow nanospheres and (b) Li4Ti5O12@C/CNT microspheres; inset represents the outer surface structure of a microsphere. | |
TEM and HRTEM images of the Li4Ti5O12 hollow nanospheres and Li4Ti5O12@C/CNT microspheres are shown in Fig. 4. The shell thickness of the Li4Ti5O12 hollow nanospheres is about 10 nm (Fig. 4a), which is too thin to resist the stress from the long charge and discharge processes. The lattice spacing of 0.486 nm corresponds to the (111) crystal facet of the Li4Ti5O12@C/CNT microspheres. Meanwhile, the carbon layers coated on the surface of the Li4Ti5O12 nanoparticles measure about 1 nm. The coated carbon layers can not only improve the electroconductibility but also prevent direct contract between the electrolyte and Li4Ti5O12 to reduce side effects.
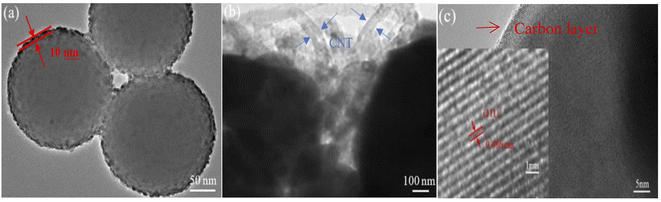 |
| Fig. 4 TEM images of the (a) Li4Ti5O12 hollow nanospheres and (b) Li4Ti5O12@C/CNT microspheres. (c) HRTEM image of the Li4Ti5O12@C/CNT microspheres. | |
The Raman test results of the Li4Ti5O12@C/CNT microspheres are shown in Fig. S4.† The presence of carbon is identified by the D and G peaks of the Li4Ti5O12@C/CNT microspheres. An ID/IG ratio greater than 1 indicates that the coated carbon layers are highly graphitized. The disordered D-band peak located at 1340 cm−1 and graphitic G-band peak located at 1591 cm−1 represent the crystal defects of carbon atoms and sp3 hybridization, respectively. Abundant defects are conducive to the diffusion of sodium ions. In addition, the defects accelerate the transmission of electrons to improve conductibility. Fig. S5† presents the thermogravimetric curve of the Li4Ti5O12@C/CNT microspheres. Three stages of weight loss were found. The 0.27 wt%, 7.38 wt% and 3.74 wt% weight losses are attributed to the loss of adsorbed water, coated carbon layers, and CNTs, respectively, indicating that the carbon layers were successfully coated on the surface of the Li4Ti5O12 nanoparticles and the CNTs were successfully composited with the Li4Ti5O12 nanoparticles, which is consistent with the results in Fig. 3b and 4b. The N2 adsorption/desorption isotherms of the Li4Ti5O12@C/CNT microspheres and Li4Ti5O12 hollow nanospheres were recorded to test the specific surface area. The specific surface area of the Li4Ti5O12 hollow nanospheres is slightly lower than that of the Li4Ti5O12@C/CNT microspheres (Fig. S6†), which is the result of the special porous spherical structure. The abundant nanometer and micrometer-scale pores, distributed on the surface of the Li4Ti5O12@C/CNT microspheres, are conducive to increasing the contact area between the electrode and electrolyte and increasing the specific surface area.
Fig. 5a exhibits the cycling stability curves of the Li4Ti5O12@C/CNT microspheres and Li4Ti5O12 hollow nanospheres at a current density of 100 mA g−1 for 150 cycles. The obvious capacity attenuation phenomenon appeared in the first two cycles, which is the result of the decomposition of the electrolyte. The charge/discharge capacities of the Li4Ti5O12@C/CNT microspheres are both significantly higher than those of the Li4Ti5O12 hollow nanospheres at different cycles. Moreover, the Li4Ti5O12@C/CNT microspheres show superior cycling stability. The charge/discharge capacities of the Li4Ti5O12@C/CNT microspheres decreased from 172.1/174.9 mA h g−1 to 122.8/124.1 mA h g−1 after 150 cycles. By contrast, the Li4Ti5O12 hollow nanospheres exhibit poorer cycling stability; the charge/discharge capacities decay from 171.4/174.3 mA h g−1 to 82.2/84.6 mA h g−1 after 150 cycles. The longer cycling stability of the Li4Ti5O12@C/CNT microspheres is attributed to their special structural design. Moreover, the abundant nanometer and micrometer-scale pores distributed on the surface of the microspheres can effectively relieve the huge volume change from the repeated extraction–insertion of sodium ions. In addition, the CNTs play a key role in enhancing the structural stability of the microspheres. The Li4Ti5O12 nanoparticles are firmly anchored by the CNTs, forming microspheres with an extremely stable structure. The cycling stability test results of the Li4Ti5O12@C/CNT microspheres and Li4Ti5O12 hollow nanospheres at a current density of 500 mA g−1 for 500 cycles are shown in Fig. S7† to further confirm the superior cycling stability of the Li4Ti5O12@C/CNT microspheres. The charge/discharge capacities (85.45/86.18 mA h g−1) of the Li4Ti5O12@C/CNT microspheres are higher than those of the Li4Ti5O12 hollow nanospheres (13.52/14.07 mA h g−1) after 500 cycles, and the capacity attenuation of the Li4Ti5O12@C/CNT microspheres is much lower than that of the Li4Ti5O12 hollow nanospheres, indicating the superior cycling stability of the Li4Ti5O12@C/CNT microspheres. The rate performances of the Li4Ti5O12@C/CNT microspheres and Li4Ti5O12 hollow nanospheres were tested to identify the superior conductibility of the Li4Ti5O12@C/CNT microspheres (Fig. 5b). The capacity decay rate of the Li4Ti5O12 hollow nanospheres is significantly faster than that of the Li4Ti5O12@C/CNT microspheres with the increase in current density. A charge capacity of 61.16 mA h g−1 is maintained at 1000 mA g−1, and a 138.81 mA h g−1 charge capacity is retained when the current density recovers to 50 mA g−1 (Li4Ti5O12@C/CNT microspheres). As a comparison, the charge capacity of the Li4Ti5O12 hollow nanospheres decays from 132.94 mA h g−1 (50 mA g−1) to 18.32 mA h g−1 (1000 mA g−1), and the charge capacity recovers to 119.38 mA h g−1 when the current density decreases to 50 mA g−1. The excellent rate capability of the Li4Ti5O12@C/CNT microspheres can be attributed to the coated carbon layers and CNTs. The carbon layers coated on the surface of the Li4Ti5O12 nanoparticles are beneficial to improving the electronic conductivity, promoting the transfer of electrons, and enhancing the rate capability of Li4Ti5O12. A spherical conductive network structure was formed due to the synergetic effect of the CNTs and carbon layers, which provides more transmission paths for electrons.
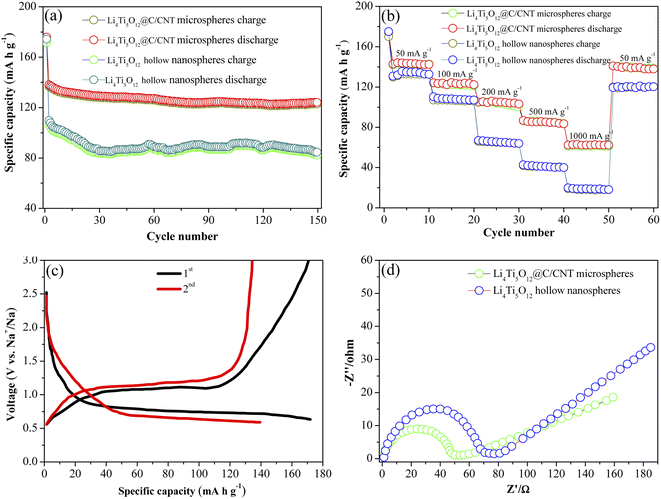 |
| Fig. 5 (a) Cycling curves of the Li4Ti5O12@C/CNT microspheres and Li4Ti5O12 hollow nanospheres at a current density of 100 mA g−1, (b) rate performance of the Li4Ti5O12@C/CNT microspheres and Li4Ti5O12 hollow nanospheres, (c) charge and discharge profiles of the Li4Ti5O12@C/CNT microspheres, and (d) Nyquist plots of Li4Ti5O12@C/CNT microspheres and Li4Ti5O12 hollow spheres. | |
The charge/discharge curves of the Li4Ti5O12@C/CNT microspheres are shown in Fig. 5c. The stable voltage platforms located at 1.07 V and 0.78 V correspond to the charge voltage platform and discharge voltage platform, respectively, implying that the structure of the Li4Ti5O12@C/CNT microspheres is extremely stable. On the contrary, the Li4Ti5O12 hollow nanospheres display a higher charge voltage platform (1.21 V) and lower discharge voltage platform (0.65 V) due to polarization (Fig. S8†). The polarization of the Li4Ti5O12@C/CNT microspheres is effectively alleviated by the coated carbon layers and composited CNTs, which greatly improve the electroconductivity and promote the kinetic properties.
The first three cycles of cyclic voltammetry were performed to analyze the electrochemical reaction process of the Li4Ti5O12@C/CNT microspheres during the process of Na-ion insertion–extraction (Fig. S9†). The reduction/oxidation peaks located at 0.72/1.12 V are attributed to the sodium-ion insertion–extraction of the Li4Ti5O12 nanoparticles in the first cycle. Compared with the first cycle, the reduction peaks of the last two cycles have been changed significantly, which is due to the irreversible sodium insertion process. The cyclic voltammetry curves of the first three cycles almost overlap, indicating the excellent cycling stability of the Li4Ti5O12@C/CNT microspheres.
Electrochemical impedance spectroscopy of the Li4Ti5O12@C/CNT microspheres and Li4Ti5O12 hollow nanospheres was performed to identify the superiority of the spherical conductive network structure (Fig. 5d). The charge transfer resistance of the Li4Ti5O12@C/CNT microspheres is lower than that of the Li4Ti5O12 hollow nanospheres, which is attributed to the superior conductivity of the Li4Ti5O12@C/CNT microspheres. Compared with the Li4Ti5O12 hollow nanospheres, the Li4Ti5O12@C/CNT microspheres are composed of carbon layers and CNTs, and a conductive network structure is formed due to the synergy of the carbon layers and CNTs. The spherical structure of the Li4Ti5O12@C/CNT microspheres is essentially maintained at 500 mA g−1 for 500 cycles (Fig. S10†), which further indicates the stable spherical structure of the Li4Ti5O12@C/CNT microspheres.
4. Conclusions
Herein, Li4Ti5O12@C/CNT microspheres with numerous nanometer and micrometer-scale pores were synthesized, which show excellent sodium-storage capability. The charge/discharge capacities of 85.45/86.18 mA h g−1 are maintained after 500 cycles at 500 mA g−1, exhibiting long cycling stability. The CNTs tightly anchored to the Li4Ti5O12@C nanoparticles, and the various nanometer/micrometer-scale pores distributed on the surface of the microspheres play a vital role in improving the cycling stability. The CNTs ensure the stability of the spherical structure during the long-term extraction–insertion of sodium ions. The abundant pores are able to effectively relieve the volume expansion from the charge/discharge processes. In addition, a charge capacity of 61.16 mA h g−1 is kept at a high current density of 1000 mA g−1, showing high rate capability, which is the result of the coated carbon layers and CNTs. The carbon layers coated on the Li4Ti5O12 nanoparticles greatly improve the electroconductivity of the Li4Ti5O12 nanoparticles. Meanwhile, a 3D continuous conductive network is formed via the tandem action of the CNTs. There are correlations between the Li4Ti5O12@C nanoparticles. The prepared Li4Ti5O12@C/CNT microspheres indicate that the design of the nanometer/micrometer porous conductive network structure is an effective method to enhance the sodium-storage capability, which provides a new idea for the fabrication of anode materials for high-performance sodium-ion batteries.
Conflicts of interest
The authors declare no competing financial interest.
Acknowledgements
This work was supported by the National Natural Science Foundation of China (51725101, 11727807, 51672050, 61790581), the Ministry of Science and Technology of China (973 Project No. 2018YFA0209102) and the Science and Technology research project of Jiangxi Provincial Department of Education (GJJ200338).
References
- X. L. Jia, Y. F. Kan and X. Zhu, et al., Building flexible Li4Ti5O12/CNT lithium-ion battery anodes with superior rate performance and ultralong cycling stability, Nano energy, 2014, 10, 344–352 CrossRef CAS.
- J. H. Park, S. W. Kang and T. S. Kwon, et al., Spray-drying assisted synthesis of a Li4Ti5O12/C composite for high rate performance lithium ion batteries, Ceram. Int., 2018, 44(3), 2683–2690 CrossRef CAS.
- F. Zhang, F. Y. Yi and T. Meng, et al., In Situ Supramolecular Self-Assembly Assisted Synthesis of Li4Ti5O12-Carbon-Reduced Graphene Oxide Microspheres for Lithium-Ion Batteries, ACS Sustainable Chem. Eng., 2019, 7(1), 916–924 CrossRef CAS.
- W. B. Zhu, Z. Y. Zhuang and Y. M. Yang, et al., Synthesis and electrochemical performance of hole-rich Li4Ti5O12 anode material for lithium-ion secondary batteries, J. Phys. Chem. Solids, 2016, 93, 52–58 CrossRef CAS.
- H. G. Jung, J. Kim and B. Scrosati, et al., Micron-sized, carbon-coated Li4Ti5O12 as high power anode material for advanced lithium batteries, J. Power Sources, 2011, 196(18), 7763–7766 CrossRef CAS.
- I. Belharouak, G. M. Koening and T. Tan, et al., Performance Degradation and Gassing of Li4Ti5O12/LiMn2O4 Lithium-Ion Cells, J. Electrochem. Soc., 2012, 159, A1165–A1170 CrossRef CAS.
- J. Li, S. Huang and S. F. Li, et al., Synthesis and electrochemical performance of Li4Ti5O12/Ag composite prepared by electroless plating, Ceram. Int., 2017, 43(2), 1650–1656 CrossRef CAS.
- Z. X. Sun, L. J. Sun and S. W. Koh, et al., Photovoltaic-powered supercapacitors for driving overall water splitting: A dual-modulated 3D architecture, Carbon Energy, 2022, 1–12 Search PubMed.
- G. N. Zhu, H. J. Liu and J. H. Zhuang, et al., Carbon-coated nano-sized Li4Ti5O12 nanoporous micro-sphere as anode material for high-rate lithium-ion batteries, Energy Environ. Sci., 2011, 4(10), 4016–4022 RSC.
- B. Q. Hu, X. S. Zhou and J. Xu, et al., Excellent Rate and Low Temperature Performance of Lithium-Ion Batteries based on Binder-Free Li4Ti5O12Electrode, Chemelectrochem, 2020, 7, 3, DOI:10.1002/celc.201901914.
- L. Deng, W. H. Yang and S. X. Zhou, et al., Effect of carbon nanotubes addition on electrochemical performance and thermal stability of Li4Ti5O12 anode in commercial LiMn2O4/Li4Ti5O12 full-cell, Chin. Chem. Lett., 2015, 26(12), 1529–1534 CrossRef CAS.
- G. Hasegawa, K. Kanamori and T. Kiyomura, et al., Hierarchically Porous Li4Ti5O12 Anode Materials for Li- and Na-Ion Batteries: Effects of Nanoarchitectural Design and Temperature Dependence of the Rate Capability, Adv. Energy Mater., 2015, 5(1), 1400730 CrossRef.
- S. Ghosh, S. Mitra and P. Barpanda, et al., Sonochemical Synthesis of Nanostructured Spinel Li4Ti5O12 Negative Insertion Material for Li-ion and Na-ion Batteries, Electrochim. Acta, 2021, 392, 139026 CrossRef.
- S. H. Gong, J. H. Lee and D. W. Chun, et al., Effects of Cr doping on structure and electrochemical properties of Li4Ti5O12 nanostructure for sodium-ion battery anode, J. Energy Chem., 2021, 59, 465–472 CrossRef.
- M. Kitta, T. Kojima and R. Kataoka, et al., Realizing the Single-Phase Spinel-Type Sodium Titanium Oxide with the Li4Ti5O12-like Structure for Building Stable Sodium-Ion Batteries, ACS Appl. Mater. Interfaces, 2020, 12(8), 9322–9331 CrossRef CAS.
- G. B. Xu, L. W. Yang and X. L. Wei, et al., MoS2-Quantum-Dot-Interspersed Li4Ti5O12 Nanosheets with Enhanced Performance for Li- and Na-Ion Batteries, Adv. Funct. Mater., 2016, 26(19), 3349–3358 CrossRef CAS.
- C. J. Chen, H. H. Xu and T. F. Zhou, et al., Integrated Intercalation-Based and Interfacial Sodium Storage in Graphene-Wrapped Porous Li4Ti5O12 Nanofibers Composite Aerogel, Adv. Energy Mater., 2016, 6(13), 1600322 CrossRef.
- M. Kitta, R. Kataoka and S. Tanaka, et al., Spinel-Type Sodium Titanium Oxide: A Promising Sodium-Insertion Material of Sodium-Ion Batteries, ACS
Appl. Mater. Interfaces, 2019, 2(6), 4345–4353 CAS.
- G. B. Xu, Y. Tian and X. L. Wei, et al., Free-standing electrodes Composed of carbon-coated Li4Ti5O12 nanosheets and reduced graphene oxide for advanced sodium ion batteries, J. Power Sources, 2017, 337, 180–188 CrossRef CAS.
- J. Q. Wang, W. H. Li and Z. Z. Yang, et al., Free-standing and binder-free sodium-ion electrodes based on carbon-nanotube Li4Ti5O12 nanoparticles embedded in carbon nanofibers, RSC Adv., 2014, 4(48), 25220–25226 RSC.
- T. Lan, Q. Qiao and F. X. Hu, et al., Electrochemical Oscillation during the Galvanostatic Charging of Li4Ti5O12 in Li-Ion Batteries, J. Phys. Chem. C, 2021, 125(27), 14549–14558 CrossRef CAS.
- J. Xu, D. M. Zhang and Z. P. Zhang, et al., A high performance all-vanadate-based Li-ion full cell, J, Mater. Chem. A, 2021, 9(6), 10345–10353 RSC.
- C. W. Chang-Jian, B. C. Ho and C. K. Chung, et al., Doping and surface modification enhance the applicability of Li4Ti5O12 microspheres as high-rate anode materials for lithium ion batteries, Ceram. Int., 2018, 44(18), 23063–23072 CrossRef CAS.
- W. C. Chien, Z. H. Wu and Y. C. Hsieh, et al., Electrochemical performance of Li4Ti5O12 anode materials synthesized using a spray-drying method, Ceram. Int., 2020, 46(17), 26923–26935 CrossRef CAS.
- T. Lan, Q. Qiao and F. X. Hu, et al., Electrochemical Oscillation during the Galvanostatic Charging of Li4Ti5O12 in Li-Ion Batteries, J. Phys. Chem. C, 2021, 125(27), 14549–14558 CrossRef CAS.
- J. H. Park, S. W. Kang and T. S. Kwon, et al., Spray-drying assisted synthesis of a Li4Ti5O12/C composite for high rate performance lithium ion batteries, Ceram. Int., 2018, 44(3), 2683–2690 CrossRef CAS.
- E. C. Cho, C. W. Chang-Jian and J. A. Chou, et al., MWCNT-embedded Li4Ti5O12 microspheres interfacially modified with polyaniline as ternary composites for high-performance lithium ion battery anodes, Ceram. Int., 2020, 46(5), 6801–6810 CrossRef CAS.
- D. B. Ruan, M. S. Kim and B. Yang, et al., 700 F hybrid capacitors cells composed of activated carbon and Li4Ti5O12 microspheres with ultra-long cycle life, J. Power Sources, 2017, 366, 200–206 CrossRef CAS.
- H. K. Roh, G. W. Lee and S. Haghighat, Polyol-mediated carbon-coated Li4Ti5O12 nanoparticle/graphene composites with long-term cycling stability for lithium and sodium ion storages, Chem. Eng. J., 2020, 385, 123984 CrossRef.
|
This journal is © The Royal Society of Chemistry 2022 |
Click here to see how this site uses Cookies. View our privacy policy here.