DOI:
10.1039/D2RA05050A
(Paper)
RSC Adv., 2022,
12, 27546-27549
Copper-catalyzed [3+3] annulation of ketones with oxime acetates for the synthesis of pyridines†
Received
12th August 2022
, Accepted 19th September 2022
First published on 28th September 2022
Abstract
A novel and efficient copper-catalyzed strategy for the synthesis of diverse pyridines through the [3+3] annulation of ketones with oxime acetates has been reported. It is very convenient to obtain various unsymmetrical 2,6-diarylpyridines by changing different substrates. The rare copper-catalyzed direct difunctionalization of saturated ketones for the synthesis of nitrogen heterocycles is developed. This protocol has excellent functional group tolerance, readily available raw materials, high chemoselectivity and broad substrate scope.
Introduction
Pyridine skeletons have attracted extensive attention because they are not only core structural elements in synthetic pharmaceuticals and natural products (such as common vitamin B6, nicotine and clivimine) but also play pivotal roles in materials science, coordination chemistry, and small-molecule catalysis.1 Therefore, the development of approaches for the convenient and efficient synthesis of substituted pyridines has been an important work in heterocyclic chemistry.2 In addition to the traditional methods based on the condensation of aldehyde, β-keto ester and amine (such as the Hantzsch dihydropyridine synthesis), many alternative methods have been actively explored to date, especially those catalyzed by transition metals.3 In recent years, the [3+3]-type annulation has been shown to be an effective strategy for the transition-metal-catalyzed synthesis of pyridine derivatives.4 In the above context, oxime derivatives as the C–C–N building blocks and the internal oxidants have been widely used to the synthesis of structurally different pyridine scaffolds. For example, in 2013, Yoshikai's group disclosed a copper-catalyzed [3+3]-type annulation of α,β-unsaturated aldehydes with oxime acetates for the synthesis of polysubstituted pyridines (Scheme 1a).5 Subsequently, they further extended this work and synthesized highly substituted pyridines (Scheme 1b).6 In 2018, Li's group reported the synthesis of fluoroalkylated pyridines by Cu-catalyzed [3+3] annulation of β-trifluoromethylated ketenes and oxime acetates (Scheme 1c).7
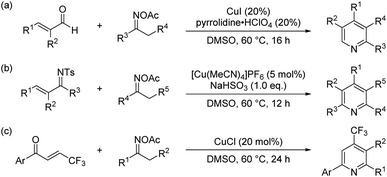 |
| Scheme 1 Methods for pyridine scaffolds synthesis via [3+3] annulation. | |
Ketones constitute a powerful class of useful synthons which are versatile for various organic synthesis transformations, importantly the synthesis of heterocyclic derivatives. In recent years, the β-functionalization of saturated ketones has received much attention, and various methods to construct β-functionalized ketones have been developed.8 For example, in 2016, Su's group reported a copper-catalyzed protocol for the direct β-functionalization of saturated ketones with a diversity of nitrogen, oxygen and carbon nucleophiles (Scheme 2a).9 Subsequently, Pan's group developed a copper-catalyzed N–H olefination of sulfonamides for the synthesis of enaminones using saturated ketones as an olefin source (Scheme 2b).10 In 2019, Li's group also reported similar work (Scheme 2c).11 These elegant works provide a practical approach for the direct β-functionalization of saturated ketones. However, both the α or β functionalization of saturated ketones are single-site reactions, and their bifunctionalization reactions are rarely reported.
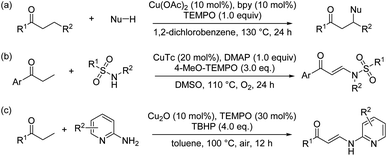 |
| Scheme 2 Methods for β-functionalization of ketones. | |
We have always been interested in the synthesis and modification of nitrogen heterocycles.12 Inspired by those pioneering works,5–7,9–11 we consider oxime esters as substrates to achieve α,β-bifunctionalization of saturated ketones. The specific strategy is as follows: TEMPO-assisted copper(II)-catalyzed in situ generation of enones from saturated ketones 1. Oxime esters 2 can be successfully converted to imines or enamines by copper(I) catalysis. The formed imines or enamines then undergo [3+3]-type annulation with the in situ generated α,β-unsaturated ketones to afford dihydropyridines, which is further oxidized to give polysubstituted pyridines 3 or 3′(Scheme 3). It directly realizes the α,β-bifunctionalization of unsaturated ketones to synthesize pyridine derivatives without using α,β-unsaturated aldehydes or α,β-unsaturated ketimines through multi-step synthesis. However, there are two challenges to implementing this process: firstly, incompatibility issues could arise if the multiple dehydrogenation of saturated ketones would lead to excessive oxidation of the coupling partners; secondly, there is a problem of chemoselectivity during annulation, and two products may be obtained. We hereby report a copper-catalyzed [3+3]-type reactions of saturated ketones with oxime acetates for the synthesis of polysubstituted pyridines.
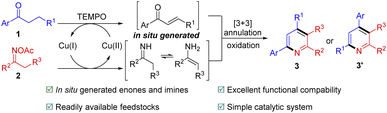 |
| Scheme 3 Our strategy for pyridine scaffolds synthesis via [3+3] annulation. | |
Results and discussion
We commenced our study by choosing commercially available propiophenone 1a and O-acetyl ketoxime 2a as model substrates for the optimization reaction conditions. Upon screening a variety of copper catalysts, ligands, oxidants, additives, solvents and temperature, optimized reaction conditions are determined as follows: 1a (0.5 mmol), 2a (0.2 mmol), CuBr (10 mol%), 1,10-phenanthroline (10 mol%), NaIO4 (0.2 mmol), NaOAc (0.1 mmol), TEMPO (0.04 mmol); DMSO is used as reaction solvent; and the reaction is carried out under an N2 atmosphere at 120 °C for 24 h (Table, entry 1). The reaction cannot proceed without a copper catalyst (entry 2). Absent the addition of ligands, the efficiency of the reaction will drop significantly (entry 3). TEMPO is essential for the smooth realization of the reaction (entry 4). Change various copper catalysts, the reaction effect is obviously different, CuBr showed the best effect to the reaction (entries 5–8). Compared with other ligand, 1,10-phenanthroline promotes the reaction better (entry 9). Although 4-OH-TEMPO can also promote the reaction, the effect of the reaction is not as good as that of TEMPO (entry 10). By increasing the amount of TEMPO and avoiding the addition of oxidants, the efficiency of obtaining the product is significantly reduced (entries 11 and 12). In addition, changing the type of oxidants or increasing the dosage, the reaction effect is not so good as the standard conditions (entries 13–16). Similarly, with other types of bases or solvents, there was no further improvement in the effect of the reaction.
Based on the above optimization conditions, the synthetic scope and generality of this reaction is probed next. Variations of ketones are first tested (Scheme 4). In general, a range of electronically different functionalities on the phenyl ring of propiophenones are well compatible to give desired pyridines in moderate yields (3a–3j). Especially, aromatic ketones with weak electron-donating groups and weak electron-withdrawing groups, such as aliphatic groups (3a–3d) and halogen groups (3e–3g), performed much better in the [3+3]-type reaction. In addition, the steric effect has a great influence on the transformation (3d vs. 3j). Notably, electron-donating heteroaromatic ketone (2-propionylthiophene) smoothly underwent the reaction without by-product at their reactive C-3 position of heteroaromatic ring, while electron-withdrawing heterocyclic ketone (3-propionylpyridine) cannot obtain product 3k. Although in low yield, 1-(p-tolyl)butan-1-one can also obtain 2,4,6-trisubstituted pyridine 3m. Aliphatic ketones failed to afford the corresponding products (3n and 3p). The overall yield of the reaction is relatively low, there may be two reasons: (1) the efficiency of TEMPO-assisted copper-catalyzed conversion of unsaturated ketones to enones is very low, and a large number of unsaturated ketones can be recovered; (2) on the other hand, oxime acetates are easily decomposed under these conditions and cannot rapidly capture the enone produced in situ, resulting in a low reaction yield.
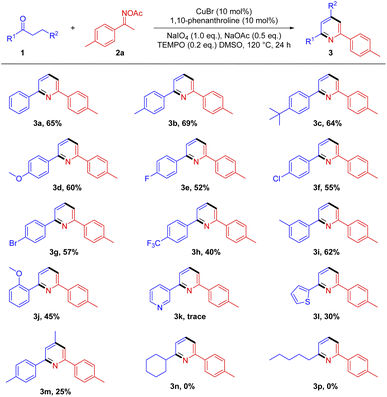 |
| Scheme 4 Substrate scope of ketones. | |
To show the generality of the copper-catalyzed [3+3] annulation, a range of substituted oxime acetates is evaluated in Scheme 5. Acetophenone oximes bearing a variety of functionalities with diverse substitution patterns on the benzene ring afforded the target products in moderate yields (4a–4l). Both electron-donating groups and weak electron-withdrawing groups functionalities are well tolerated. In addition, oxime esters with multiple substituents can also smoothly obtain the target products (4f, 4g and 4i). Among them, the steric hindrance effect has a certain influence on the reaction (4d and 4g). The oxime acetates from naphthalenyl ketone give moderate yield of the product 4l. Unfortunately, oximes from aliphatic ketones such as hexan-2-one afforded very low yield of product.
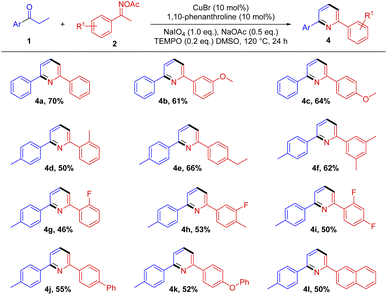 |
| Scheme 5 Substrate scope of ketones and oxime ester compounds. | |
To achieve insights into the mechanism of this reaction, several control experiments are conducted in Scheme 6. When the reaction is in the absence of TEMPO, the reaction cannot proceed (Table 1, entry 4). During the reaction, we monitored the formation of α,β-unsaturated ketone (Scheme 6a). The reaction of an α,β-unsaturated ketone 1a′ with O-acetyl ketoxime 2a is performed under the standard conditions, afforded pyridine 3a in 80% yield (Scheme 6b). Therefore, it shows that α,β-unsaturated ketone might be an intermediate of the reaction. Finally, we also use oxime 2a′ instead of oxime ester 2a, the reaction cannot happen (Scheme 6c).
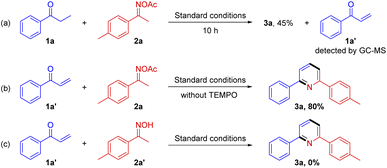 |
| Scheme 6 Mechanistic study. | |
Table 1 Optimization of reaction conditionsa

|
Entry |
Variations from standard conditions |
Isolated yield of 3a (%) |
Standard conditions: 1a (0.5 mmol), 2a (0.2 mmol), CuBr (10 mol%), 1,10-phenanthroline (10 mol%), NaIO4 (0.2 mmol), NaOAc (0.1 mmol), TEMPO (0.04 mmol), DMSO (1.0 mL), 120 °C, 24 h, N2 atmosphere. |
1 |
None |
65 |
2 |
No CuBr |
0 |
3 |
No 1,10-phenanthroline |
32 |
4 |
No TEMPO |
0 |
5 |
CuCl instead of CuBr |
50 |
6 |
CuI instead of CuBr |
55 |
7 |
Cu(OAc)2 instead of CuBr |
38 |
8 |
Cu(OTf)2 instead of CuBr |
18 |
9 |
bpy instead of 1,10-phenanthroline |
60 |
10 |
4-OH-TEMPO instead of TEMPO |
58 |
11 |
TEMPO (1.0 eq.), no NaIO4 |
42 |
12 |
TEMPO (2.0 eq.), no NaIO4 |
39 |
13 |
O2 instead of NaIO4 |
Trace |
14 |
TBHP instead of NaIO4 |
45 |
15 |
K2S2O8 instead of NaIO4 |
38 |
16 |
NaIO4 (1.5 eq.) |
63 |
17 |
Cs2CO3 instead of NaOAc |
54 |
18 |
t-BuONa instead of NaOAc |
42 |
19 |
Toluene instead of DMSO |
49 |
20 |
PhCl instead of DMSO |
55 |
Based on the above control experiments and literature,5–7,9–11,13 a plausible mechanism for the copper-catalyzed [3+3]-type reaction is proposed in Scheme 7. Firstly, Cu(II) as Lewis acid reacts with propiophenone 1a to form metalenolate complex A. Then, homolysis of the A generates Cu(I) species and aromatic ketone radical intermediate B is captured by TEMPO to form α-TEMPO-substituted ketone C that undergoes fast TEMPOH-elimination to form enones 1a′. On the other hand, Cu(I)-catalyzed reduction of the N–O bond of ketoxime acetate 2a via a sequential single electron transfer process forms the imine E or enamine F intermediate. Next, nucleophilic addition of enamine F to enones intermediate 1a′ produces the enamine intermediate G. Then, the dihydropyridine H can be obtained by an intramolecular condensation reaction. Finally, oxidative aromatization of intermediate H yields pyridine 3a.
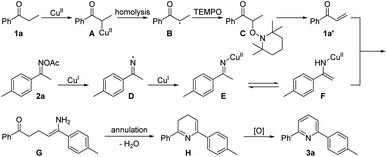 |
| Scheme 7 Proposed mechanism. | |
Conclusions
In conclusion, we have successfully developed a practical Cu-catalyzed formal [3+3] annulation of ketones with oxime acetates, providing efficient approach to valuable polysubstituted pyridines in moderate yields. In this process, saturated ketones and oxime acetates are converted to the corresponding enones and imines, respectively, which then undergo cascade annulation and oxidative aromatization to yield pyridine derivatives. It is a copper-catalyzed bifunctionalization of saturated ketones for the synthesis of nitrogen heterocycles, but also has the advantages of excellent functional group tolerance, ready availability of the raw materials, high chemoselectivity, broad substrate scope, and cheap catalysts.
Conflicts of interest
There are no conflicts to declare.
Acknowledgements
We appreciate the Project funded by China Postdoctoral Science Foundation (No. 2022M711481), the Scientific Research Fund of Jiangxi Provincial Education Department (No. GJJ201504), the Scientific Research Project of Gannan Medical University (No. YB201903) for financial support.
Notes and references
-
(a) C. Allais, J. M. Grassot, J. Rodriguez and T. Constantieux, Chem. Rev., 2014, 114, 10829 CrossRef CAS PubMed;
(b) D. M. Peloquin and T. A. Schmedake, Coord. Chem. Rev., 2016, 323, 107 CrossRef CAS;
(c) S. X. Lin, M. A. Curtis and J. Sperry, Bioorg. Med. Chem., 2020, 28, 115820 CrossRef CAS PubMed;
(d) D. H. Ma, B. S. Martin, K. S. Gallagher, T. Saito and M. J. Dai, J. Am. Chem. Soc., 2021, 143(40), 16383 CrossRef CAS PubMed;
(e) M. Sheokand, Y. Rout and R. Misra, J. Mater. Chem. C, 2022, 10, 6992 RSC;
(f) F. Wang, L. Q. Zhang, W. G. Han, Z. Y. Bin and J. S. You, Angew. Chem., Int. Ed., 2022, 61, e202205380 CAS.
-
(a) A. V. Gulevich, A. S. Dudnik, N. Chernyak and V. Gevorgyan, Chem. Rev., 2014, 114, 10829 CrossRef PubMed;
(b) Z. D. Song, X. Huang, W. B. Yi and W. Zhang, Org. Lett., 2016, 18, 5640 CrossRef CAS PubMed;
(c) B. Stanovnik, Eur. J. Org. Chem., 2019, 5120 CrossRef CAS;
(d) D. A. Shabalin, Org. Biomol. Chem., 2021, 19, 8184 RSC;
(e) Q. Chen, S. H. Chen, H. Q. Wu, X. Q. Zeng, W. Q. Chen, G. X. Sun and Z. Y. Wang, Chinese J. Org. Chem., 2021, 41, 3482 CrossRef;
(f) X. H. Li, W. Cai and Y. Huang, Adv. Synth. Catal., 2022, 364, 1879 CrossRef CAS.
-
(a) S. Liu and L. S. Liebeskind, J. Am. Chem. Soc., 2008, 130, 6918 CrossRef CAS PubMed;
(b) Z. H. Ren, Z. Y. Zhang, B. Q. Yang, Y. Y. Wang and Z. H. Guan, Org. Lett., 2011, 13, 5394 CrossRef CAS PubMed;
(c) J. C. Wu, W. B. Xu, Z. X. Yu and J. Wang, J. Am. Chem. Soc., 2015, 137, 9489 CrossRef CAS PubMed;
(d) M. N. Zhao, Z. H. Ren, L. Yu, Y. Y. Wang and Z. H. Guan, Org. Lett., 2016, 18, 1194 CrossRef CAS PubMed;
(e) Y. J. Fu, P. P. Wang, X. Guo, P. Wu, X. Meng and B. H. Chen, J. Org. Chem., 2016, 81, 11671 CrossRef CAS PubMed;
(f) C. S. Wang, Q. Sun, F. Garcia, C. Wang and N. Yoshikai, Angew. Chem., Int. Ed., 2021, 60, 9627 CrossRef CAS PubMed;
(g) X. L. Li, X. Q. Zhang, F. K. Zhang, X. Z. Luo and H. Q. Luo, Adv. Synth. Catal., 2022, 364, 1683 CrossRef CAS.
-
(a) D. C. Bai, X. L. Wang, G. F. Zheng and X. W. Li, Angew. Chem., Int. Ed., 2018, 57, 6633 CrossRef CAS;
(b) J. L. Zhan, M. W. Wu, D. Wei, B. Y. Wei, Y. Jiang, W. Yu and B. Han, ACS Catal., 2019, 9, 4179 CrossRef CAS.
- Y. Wei and N. Yoshikai, J. Am. Chem. Soc., 2013, 135, 3756 CrossRef CAS PubMed.
- H. W. Huang, J. H. Cai, L. C. Tang, Z. L. Wang, F. F. Li and G. J. Deng, J. Org. Chem., 2016, 81, 1499 CrossRef CAS PubMed.
- W. W. Tan, Y. J. Ong and N. Yoshikai, Angew. Chem., Int. Ed., 2017, 56, 8240 CrossRef CAS PubMed.
-
(a) Z. X. Huang, H. N. Lim, F. Y. Mo, M. C. Young and G. B. Dong, Chem. Soc. Rev., 2015, 44, 7764 RSC;
(b) Z. X. Huang and G. B. Dong, J. Am. Chem. Soc., 2013, 135, 17747 CrossRef CAS PubMed;
(c) Y. P. Shang, X. M. Jie, J. Zhou, P. Hu, S. J. Huang and W. P. Su, Angew. Chem., Int. Ed., 2013, 52, 1299 CrossRef CAS PubMed.
- X. M. Jie, Y. P. Shang, X. F. Zhang and W. P. Su, J. Am. Chem. Soc., 2016, 138, 5623 CrossRef CAS PubMed.
- X. Liang, X. Huang, M. T. Xiong, K. X. Shen and Y. J. Pan, Chem. Commun., 2018, 54, 8403 RSC.
- T. Z. Wang, G. W. Chen, Y. J. Lu, Q. Chen, Y. P. Huo and X. W. Li, Adv. Synth. Catal., 2019, 361, 3886 CrossRef CAS.
-
(a) L. Cao, H. Zhao, Z. D. Tan, R. Q. Guan, H. F. Jiang and M. Zhang, Org. Lett., 2020, 22, 4781 CrossRef CAS PubMed;
(b) L. Cao, H. Zhao, R. Q. Guan, H. F. Jiang, P. H. Dixneuf and M. Zhang, Nat. Commun., 2021, 12, 4206 CrossRef CAS PubMed;
(c) K. Yang, S. H. Luo, S. H. Chen, X. Y. Cao, Y. J. Zhou, Y. L. Lin, Y. P. Huo and Z. Y. Wang, Org. Biomol. Chem., 2021, 19, 8133 RSC;
(d) K. Yang, Z. X. Chen, Y. J. Zhou, Q. Chen, S. W. Yu, S. H. Luo and Z. Y. Wang, Org. Chem. Front., 2022, 9, 1127 RSC.
-
(a) H. W. Huang, J. H. Cai, H. Xie, J. Tan, F. F. Li and G. J. Deng, Org. Lett., 2017, 19, 3743 CrossRef CAS PubMed;
(b) Q. H. Gao, H. J. Yan, M. M. Wu, J. J. Sun, X. Q. Yan and A. X. Wu, Org. Biomol. Chem., 2018, 16, 2342 RSC;
(c) Q. H. Gao, Y. K. Wang, Q. Q. Wang, Y. P. Zhu, Z. M. Liu and J. X. Zhang, Org. Biomol. Chem., 2018, 16, 9030 RSC;
(d) J. D. Duan, L. Zhang, G. C. Xu, H. M. Chen, X. J. Ding, Y. Y. Mao, B. S. Rong, N. Zhu and K. Guo, J. Org. Chem., 2020, 85, 8157 CrossRef CAS PubMed.
|
This journal is © The Royal Society of Chemistry 2022 |
Click here to see how this site uses Cookies. View our privacy policy here.