DOI:
10.1039/D2RA05283K
(Paper)
RSC Adv., 2022,
12, 26776-26780
Silver-catalyzed cascade cyclization and functionalization of N-aryl-4-pentenamides: an efficient route to γ-lactam-substituted quinone derivatives†
Received
23rd August 2022
, Accepted 9th September 2022
First published on 21st September 2022
Abstract
The synthesis of γ-lactam-substituted quinone derivatives through a Ag2O-catalyzed cascade cyclization and functionalization of N-aryl-4-pentenamides has been developed. Related 2-oxazolidinone substituted quinone products can be also obtained with N-aryl allyl carbamates. The reactions proceed through an amidyl radical-initiated 5-exo-trig cyclization and followed radical addition to quinones. They provide an efficient route to various γ-lactam-substituted quinone derivatives with a wide range of substrate scope.
Introduction
Quinone-based organic molecules are present in various natural products and have been widely used as building blocks in total synthesis because of their broad spectrum of biological activities, including antibacterial, antitumor, antimalarial and anti-inflammatory.1 Notably, alkyl 1,4-naphthoquinones such as menadione (vitamin K3), plumbagin, plasmodione and phytomenadione (vitamin K1) have attracted a great deal of attention due to their relevant biological activity (Fig. 1).2 Consequently, the development of general and efficient methodologies to incorporate functional groups into 1,4-naphthoquinones is of great importance.3 However, common strategies that would allow access to such compounds suffer from several drawbacks. The prevailing transition-metal-catalyzed method usually works sluggishly due to the ability of quinone substrates to act as ligands and oxidants,4 even workable examples of these underwent a Kochi–Anderson type reaction,5 despite a few exceptions.6 Alternative methods involving the prefunctionalization of substrates followed by deprotection have been proved time-consuming and labor-intensive.7 Therefore, developing more general and environmentally benign methods for the functionalization of 1,4-naphthoquinone derivatives is in great demand.8
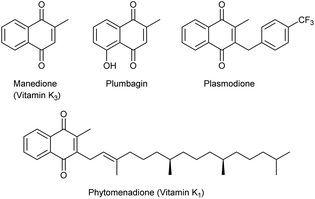 |
| Fig. 1 Examples of 1,4-naphthoquinone derivatives with biological functions. | |
Amidyl radicals recently have attracted tremendous attentions as a class of highly reactive intermediates in organic synthesis.9 In recent years, cascade cyclization and functionalization of enamides through amidyl radicals emerged as an efficient method for construction of lactam-containing molecules. Great contributions have been made by Nicolaou, Studer, Knowles, Xu, Li and others to generate amidyl radicals from N-aryl-4-pentenamides under various conditions.10–15 The generated amidyl radicals immediately initiate 5-exo-trig cyclization to form the C-centered radical, which can be applied to diverse functionalizations (Scheme 1a). Inspired by these achievements, we envisaged that the generated radicals would be trapped by electron-deficient double bond of 1,4-naphthoquinone to directly form valuable lactam-substituted quinone derivatives. Recently we disclosure a silver-mediated tandem trifluoromethylthiolation and cyclization of N-aryl-3-butenamides.16 As the extension of our current research on difunctionalization of alkenes, herein we report a silver-catalyzed cascade cyclization and functionalization of N-aryl-4-pentenamides with quinone, which provide an efficient route to γ-lactam-substituted quinone derivatives (Scheme 1b). Even though quinone and γ-lactam are both common and important organic motifs, to the best of our knowledge, the combination of two structures in a single molecule has never been reported.
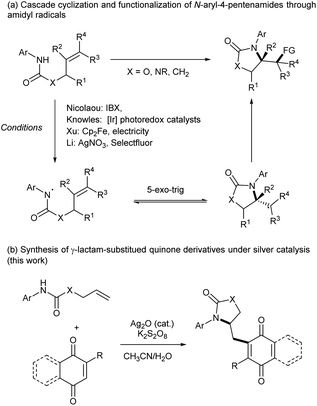 |
| Scheme 1 Cascade cyclization and functionalization of N-aryl-4-pentenamides through amidyl radicals. | |
Results and discussion
Initially, we started to explore the reaction conditions with N-phenyl-4-pentenamide (1a) and menadione (2a) as the model substrates (Table 1). To our delight, the desired product (3aa) was isolated in 33% yield in the presence of 20 mol% Ag2O with 1.5 equiv. K2S2O8 in DCM at 100 °C for 10 hours (entry 1). Then we set out to increase reaction yield by screening solvents. As shown in entries 2–5, although the reaction proceeded in DCE, DMSO and CH3CN, the highest isolated yield was afforded by CH3CN. We subsequently examined the mixed solvents (entries 6–8). The isolated yield was greatly improved from 54% to 80% in a mixture of CH3CN/H2O (1
:
1). Other silver salts were used, including AgNO3, AgOAc, AgOTf, AgF and AgO. All of these silver salts were effective to this reaction and generated similar results (entries 9–13). Given the cost and availability, Ag2O was chosen as the catalyst. Then a series of oxidants was examined. Other persulfate salts such as Na2S2O8 and (NH4)2S2O8 showed similar reactivity as K2S2O8, while oxone was much less efficient (entries 14–16). Organic peroxides were ineffective in this reaction (entries 17 and 18). Reaction temperature was also screened but the product yield decreased under either higher or lower temperature (entries 19 and 20). Finally, the attempts of reducing the substrate amount or catalyst loading all resulted in decreased yields (entries 21 and 22). Therefore, we decided to use the conditions in entry 8 as the optimized reaction conditions.
Table 1 Optimization of the reaction conditionsa
With the optimized conditions in hand, we first investigated the substrate scope of N-aryl-4-pentenamides (1) as shown in Scheme 2. When methyl groups substituted at the α position of amide, reaction still worked efficiently to generate the desired products (3ba–3ca). However, no significant Thorpe–Ingold effect was observed. For the substrates with different N-aryl moiety, the reaction proceeded smoothly to produce a wide variety of γ-lactam-substituted menadiones in good to excellent yields (3da–3ka). Either electron-donating or electron-withdrawing groups at the para, meta or ortho positions of aryl ring were tolerated without significant electronic effects. Moreover, a series of di-substituted or tri-substituted N-arylamides were also tolerated to afford the products in good to excellent yields, which shows the steric hindrance of the aryl group did not affect the efficiency of this transformation (3la–3qa). Furthermore, the reaction could be easily scaled up to gram level (for details, see the ESI†).
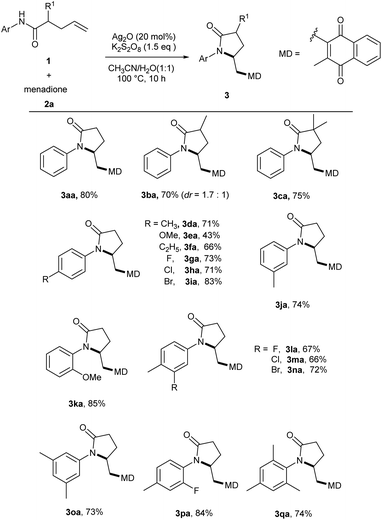 |
| Scheme 2 Substrate scope of N-aryl-4-pentenamides (1).a,b aReaction conditions: 1 (0.2 mmol), 2a (0.6 mmol), Ag2O (20 mol%), K2S2O8 (1.5 equiv.) in CH3CN/H2O (1 : 1, 2.0 mL) for 10 h. bIsolated yields. | |
Next, the substrate scope for quinones was examined as illustrated in Scheme 3. Unsubstituted 1,4-naphthoquinone generated the mono-substituted product in moderate yield without the formation of di-substituted product (3ab). Higher reactivity was observed with 2-chloro- and bromo-naphthoquinones (3ac, 3ad). The absolute structure of 3ac was unambiguously determined by the X-ray diffraction analysis and other products were assigned by analogy.17 A number of benzoquinones including alkyl, halogen, and alkoxy substituents also underwent this reaction (3ae–3ai). Relatively poor yields of 3ae and 3ah were obtained due to low conversions of their substrates.
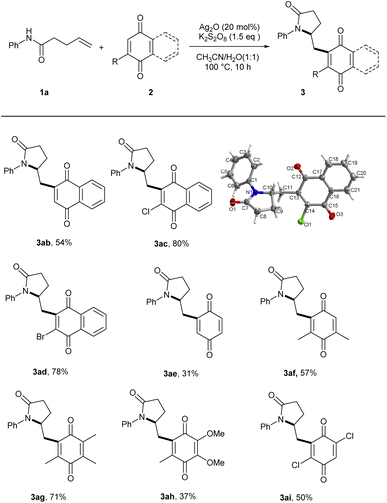 |
| Scheme 3 Substrate scope of quinones (2).a,b aReaction conditions: 1a (0.2 mmol), 2 (0.6 mmol), Ag2O (20 mol%), K2S2O8 (1.5 equiv.) in CH3CN/H2O (1 : 1, 2.0 mL) for 10 h. bIsolated yields. | |
In an effort to increase the molecular diversity, a serious of N-aryl allyl carbamates (4) was investigated as well. To our delight, these substrates were also applicable in this method and afforded the corresponding 2-oxazolidinone products (Scheme 4). The para-substituent effect was first evaluated. Various functionalities such as alkyl, halogen and trifluoromethyl were tolerated at para, meta or ortho positions of aryl ring without distinct electronic effect (5aa–5ja). Di-substituted N-arylamides could also participate in this reaction, giving the desired products in 56–73% yields (5la–5ma).
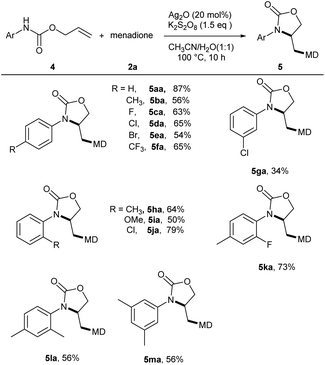 |
| Scheme 4 Substrate scope of N-aryl allyl carbamates (4).a,b aReaction conditions: 4 (0.2 mmol), 2a (0.6 mmol), Ag2O (20 mol%), K2S2O8 (1.5 equiv.) in CH3CN/H2O (1 : 1, 2.0 mL) for 10 h. bIsolated yields. | |
To gain deeper insight into the reaction mechanism, a series of experiments were conducted (Scheme 5). First, the standard reaction in the absence of silver was conducted. Instead of the difunctionalization product 3aa, only a cyclization product 6 was formed with very low conversion. It indicated the critical role of silver in this transformation. Next, the reaction of N-benzyl-4-pentenamide (7) under standard conditions was examined. It failed to generate desired product, which further proved the reaction underwent a radical mechanism since aryl group is essential to the initial amidyl radical formation.10b Finally, radical inhibition reactions were carried out with the addition of radical scavengers 2,2,6,6-tetramethyl-1-piperidinyloxy (TEMPO) or butylated hydroxytoluene (BHT). The results showed that both regents completely inhibited the transformation, although no TEMPO or BHT intermediates were detected.
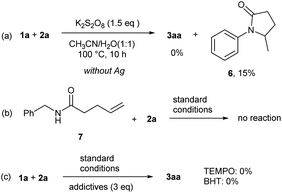 |
| Scheme 5 Control experiments. | |
Therefore, based on the experimental results and reported literature, a plausible mechanism was proposed in Scheme 6. Initially, SO4˙− radical was generated from persulfate with the assistance of Ag(I).14,18 It allows hydrogen atom abstraction (HAT) of the substrate 1a to form an amidyl radical A. Radical A readily initiates subsequent intramolecular 5-exo-trig cyclization to afford a carbon-centered radical B. The resulting radical B then undergoes a silver-promoted conjugated addition to the menadione to form the intermediate C. Finally, intermediate C undergoes HAT to regenerate Ag(I) and the desired products 3aa. The role of Ag(I) in this reaction is probably to promote the generation of SO4˙− radical and co-ordinate with the intermediate O-radical.19
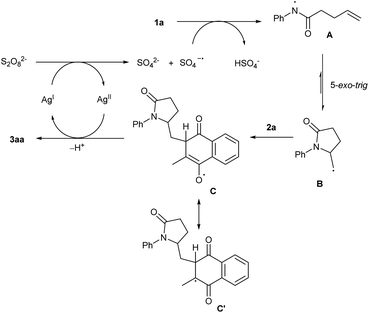 |
| Scheme 6 Proposed mechanism. | |
Conclusions
In summary, we have developed a cascade cyclization and functionalization of N-aryl-4-pentenamides with quinones. This method allows efficient access to various γ-lactam-substituted quinone derivatives with a wide range of functional group tolerance. Relative 2-oxazolidinone substituted quinone products can be also obtained with N-aryl allyl carbamates. Preliminary mechanistic studies suggested that an amidyl radical is involved in this reaction. We demonstrated the utility of the amidyl radicals for the direct construction of γ-lactam and consequent installation of quinone groups, which might be potentially used to construct more complex compounds. Relevant researches are ongoing in our laboratory and will be reported in due course.
Conflicts of interest
There are no conflicts to declare.
Acknowledgements
We are grateful to the Scientific Research Project of Hubei Education Department (T2020023 and Q20211402) and the open project of Hubei Provincial Key Laboratory of Green Materials for Light Industry (No. 202107B06) for financial support.
Notes and references
-
(a) C. Asche, Mini-Rev. Med. Chem., 2005, 5, 449–467 CrossRef CAS PubMed;
(b) V. P. Papageorgiou, A. N. Assimopoulou, E. A. Couladouros, D. Hepworth and K. C. Nicolaou, Angew. Chem., Int. Ed., 1999, 38, 270–301 CrossRef;
(c) C. Commandeur, C. Chalumeau, J. Dessolin and M. Laguerre, Eur. J. Org. Chem., 2007, 2007, 3045–3052 CrossRef;
(d) O. P. S. Patel, R. M. Beteck and L. J. Legoabe, Eur. J. Med. Chem., 2021, 210, 113084 CrossRef CAS PubMed;
(e) Y. Kumagai, Y. Shinkai, T. Miura and A. K. Cho, Annu. Rev. Pharmacol. Toxicol., 2012, 52, 221–247 CrossRef CAS PubMed.
-
(a) J.-J. Lu, J.-L. Bao, G.-S. Wu, W.-S. Xu, M.-Q. Huang, X.-P. Chen and Y.-T. Wang, Anti-Cancer Agents Med. Chem., 2013, 13, 456–463 CAS;
(b) C. V. Prasad, V. L. Nayak, S. Ramakrishna and U. V. Mallavadhani, Chem. Biol. Drug Des., 2018, 91, 220–233 CrossRef CAS PubMed;
(c) L. Salmon-Chemin, E. Buisine, V. Yardley, S. Kohler, M.-A. Debreu, V. Landry, C. Sergheraert, S. L. Croft, R. L. Krauth-Siegel and E. Davioud-Charvet, J. Med. Chem., 2001, 44, 548–565 CrossRef CAS PubMed;
(d) J. Demma, K. Hallberg and B. Hellman, Toxicol. In Vitro, 2009, 23, 266–271 CrossRef CAS PubMed;
(e) P. Mounkoro, T. Michel, S. Blandin, M.-P. Golinelli-Cohen, E. Davioud-Charvet and B. Meunier, Free Radicals Biol. Med., 2019, 141, 269–278 CrossRef CAS;
(f) M. A. Pasha, K. Anebouselvy and D. B. Ramachary, Tetrahedron, 2022, 117–118, 132793 CrossRef CAS.
- J. M. Wood, R. L. de Carvalho and E. N. da Silva Júnior, Chem. Rec., 2021, 21, 2604–2637 CrossRef CAS PubMed.
-
(a) G. A. M. Jardim, E. N. da Silva Júnior and J. F. Bower, Chem. Sci., 2016, 7, 3780–3784 RSC;
(b) Q. Wang, B. Wang, H. Deng, Y. Shangguan, Y. Lin, Y. Zhang, Z. Zhang, Y. Xiao, H. Guo and C. Zhang, J. Org. Chem., 2019, 84, 1006–1014 CrossRef CAS.
-
(a) G. Naturale, M. Lamblin, C. Commandeur, F.-X. Felpin and J. Dessolin, Eur. J. Org. Chem., 2012, 2012, 5774–5788 CrossRef CAS;
(b) Q. Gong, F. Yang, J. Hu, T. Li, P. Wang, X. Li and X. Zhang, Eur. J. Med. Chem., 2021, 224, 113707 CrossRef CAS;
(c) K. L. Woolley, M. Nadikudi, M. N. Koupaei, M. Corban, P. McCartney, A. C. Bissember, T. W. Lewis, N. Gueven and J. A. Smith, Med. Chem. Commun., 2019, 10, 399–412 RSC;
(d) M. Donzel, D. Karabiyikli, L. Cotos, M. Elhabiri and E. Davioud-Charvet, Eur. J. Org. Chem., 2021, 2021, 3622–3633 CrossRef CAS.
-
(a) J. Liu, D. Yu, Y. Yang, H. You, M. Sun, Y. Wang, X. Shen and Z.-Q. Liu, Org. Lett., 2020, 22, 4844–4847 CrossRef CAS PubMed;
(b) Z. Xu, Y. Gao, S. Wang, Q. Zhang, L. Zhang and L. Shen, J. Org. Chem., 2022, 87, 3461–3467 CrossRef;
(c) Á. Gutiérrez-Bonet, C. Remeur, J. K. Matsui and G. A. Molander, J. Am. Chem. Soc., 2017, 139, 12251–12258 CrossRef;
(d) D. Li and X. Shen, Org. Biomol. Chem., 2020, 18, 750–754 RSC.
- Y.-S. Jung, B.-Y. Joe, S. J. Cho and Y. Konishi, Bioorg. Med. Chem. Lett., 2005, 15, 1125–1129 CrossRef CAS PubMed.
- Y. Fujiwara, V. Domingo, I. B. Seiple, R. Gianatassio, M. Del Bel and P. S. Baran, J. Am. Chem. Soc., 2011, 133, 3292–3295 CrossRef CAS PubMed.
-
(a) S. Z. Zard, Chem. Soc. Rev., 2008, 37, 1603–1618 RSC;
(b) J.-R. Chen, X.-Q. Hu, L.-Q. Lu and W.-J. Xiao, Chem. Soc. Rev., 2016, 45, 2044–2056 RSC;
(c) T. Xiong and Q. Zhang, Chem. Soc. Rev., 2016, 45, 3069–3087 RSC.
-
(a) K. C. Nicolaou, Y. Zhong and P. S. Baran, Angew. Chem., Int. Ed., 2000, 39, 625–628 CrossRef CAS;
(b) K. C. Nicolaou, P. S. Baran, Y.-L. Zhong, S. Barluenga, K. W. Hunt, R. Kranich and J. A. Vega, J. Am. Chem. Soc., 2002, 124, 2233–2244 CrossRef CAS PubMed.
-
(a) J. Kemper and A. Studer, Angew. Chem., Int. Ed., 2005, 44, 4914–4917 CrossRef CAS PubMed;
(b) J. Guin, R. Fröhlich and A. Studer, Angew. Chem., Int. Ed., 2008, 47, 779–782 CrossRef CAS.
-
(a) G. J. Choi and R. R. Knowles, J. Am. Chem. Soc., 2015, 137, 9226–9229 CrossRef CAS;
(b) D. C. Miller, G. J. Choi, H. S. Orbe and R. R. Knowles, J. Am. Chem. Soc., 2015, 137, 13492–13495 CrossRef CAS.
- L. Zhu, P. Xiong, Z.-Y. Mao, Y.-H. Wang, X. Yan, X. Lu and H.-C. Xu, Angew. Chem., Int. Ed., 2016, 55, 2226–2229 CrossRef CAS PubMed.
- Z. Li, L. Song and C. Li, J. Am. Chem. Soc., 2013, 135, 4640–4643 CrossRef CAS PubMed.
-
(a) J. Jia, Y. A. Ho, R. F. Bülow and M. Rueping, Chem.–Eur. J., 2018, 24, 14054–14058 CrossRef CAS PubMed;
(b) J. Davies, T. D. Svejstrup, D. F. Reina, N. S. Sheikh and D. Leonori, J. Am. Chem. Soc., 2016, 138, 8092–8095 CrossRef CAS;
(c) S. Zheng, Á. Gutiérrez-Bonet and G. A. Molander, Chem, 2019, 5, 339–352 CrossRef CAS PubMed;
(d) J. Shi, L.-Y. Guo, Q.-P. Hu, Y.-T. Liu, Q. Li and F. Pan, Org. Lett., 2021, 23, 8822–8827 CrossRef CAS;
(e) J.-L. Tu, J.-W. Yang, W. Xu, M. Su and F. Liu, Org. Chem. Front., 2021, 8, 6405–6410 RSC.
- L. Wang, L. Xie, Z. Fang, Q. Zhang and D. Li, Org. Chem. Front., 2022, 9, 3061–3067 RSC.
- CCDC-2170961 (3ac).†.
-
(a) N. Jacobsen and K. Torssell, Acta Chem. Scand., 1973, 27, 3211–3216 CrossRef CAS;
(b) J. Goldman, N. Jacobsen and K. Torssell, Acta Chem. Scand., 1974, 28, 492–500 CrossRef CAS;
(c) X.-L. Zhu, Y. Huang, X.-H. Xu and F.-L. Qing, Org. Lett., 2020, 22, 5451–5455 CrossRef CAS PubMed.
-
(a) N. R. Patel and R. A. Flowers, J. Am. Chem. Soc., 2013, 135, 4672–4675 CrossRef CAS PubMed;
(b) Z. Huang, L. Jin, Y. Feng, P. Peng, H. Yi and A. Lei, Angew. Chem., Int. Ed., 2013, 52, 7151–7155 CrossRef CAS PubMed;
(c) S. Pandaram, A. Krishna T. P. and A. Ilangovan, Org. Biomol. Chem., 2020, 18, 3027–3031 RSC.
Footnote |
† Electronic supplementary information (ESI) available: For detailed experimental procedures, analytical data and copies of NMR spectra. CCDC 2170961. For ESI and crystallographic data in CIF or other electronic format see https://doi.org/10.1039/d2ra05283k |
|
This journal is © The Royal Society of Chemistry 2022 |
Click here to see how this site uses Cookies. View our privacy policy here.