DOI:
10.1039/D2RA05552J
(Paper)
RSC Adv., 2022,
12, 31923-31934
Enhanced pseudocapacitive energy storage and thermal stability of Sn2+ ion-intercalated molybdenum titanium carbide (Mo2TiC2) MXene†
Received
3rd September 2022
, Accepted 24th October 2022
First published on 8th November 2022
Abstract
Electrochemical energy-storage (EES) devices are a major part of energy-storage systems for industrial and domestic applications. Herein, a two-dimensional (2D) transition metal carbide MXene, namely Mo2TiC2, was intercalated with Sn ions to study the structural, morphological, optical, and electrochemical energy-storage effects. The Sn2+-intercalated modified layered structure, prepared via a facile liquid-phase pre-intercalated cetyltrimethylammonium bromide (CTAB) method, showed a higher surface area of 30 m2 g−1, low band gap of 1.3 eV, and large interlayer spacing of 1.47 nm, as compared to the pristine Mo2TiC2. The Sn@Mo2TiC2 electrode showed a high specific capacitance of 670 F g−1, representing a large diffusion control value compared to pure Mo2TiC2 (212 F g−1) at a scan rate of 2 mV s−1. The modified electrode also presented long-term cyclic performance, high-capacity retention and coulombic efficiency measured over 10
000 cycles. The Sn@Mo2TiC2 electrode showed much improved electrocatalytic efficiency, which may open up ways to employ double-transition 2D MXenes in energy-storage devices.
Introduction
The rising demands for energy across the globe, and the reducing resources of fossil fuels and their harmful impact on the environment have urged engineers, scientists, and researchers to investigate new renewable sources of energy generation. Solar and wind power systems are two major sources of renewable energy, which are efficient, clean, and environmentally friendly with great potential to fulfill future energy requirements. A key hurdle in their way, however, is how to manufacture some efficient energy-storage devices at the domestic as well as an industrial level.1 For this purpose, lithium-ion batteries are playing a key role at a commercial scale, but like fossil fuels, the sources of lithium metal are limited, and they also suffer from low energy density and high cost.2–6 So, keeping in mind the present energy usage scenario, scientists are engaged in pushing the research in two major directions: to improve the already built energy-storage systems and develop new efficient high energy-density systems.7
Electrochemical energy-storage (EES) devices are attracting the attention of researchers due to their high energy-storage capacity, cyclic stability, and fast kinetics, which have made it possible to use these systems in all kinds of electronic gadgets, hybrid electric vehicles, and grid-storage applications.8–10 Two-dimensional (2D) materials are the most suitable candidates for EES devices due to their improved physical and chemical properties. A wide range of 2D materials has been reported, including graphene, metal dichalcogenides, oxides, sulfides, and nitrides.11–18
One of the most recent advancements in the field of 2D materials is related to transition metal carbides or nitrides, also known as MXenes, which were reported for the first time in 2011.19 These MXenes are directly synthesized from the MAX phase by the selective etching of the A atomic layer in HF etching solution for the optimum time, wherein the general formula describes the composition of MAX, which is written as Mn+1AXn, where M is an early transition metal, A belongs to group IIIA or IVA elements, and X is a carbon or nitrogen element, and n = 1, 2, or 3.20 The exfoliated MXenes have the general formula Mn+1 Xn Tx, where Tx defines the surface terminations (OH, F, and O) associated with MXenes and x denotes the number of termination groups. MXenes have lot of applications in the field of energy storage,21–39 biosensing,40,41 electromagnetic interference shielding,42,43 water purification,44 ion sieving,45 and anti-bacterial activities.46,47 MXenes show pseudocapacitive behavior when used in supercapacitor applications.
In the presence of termination groups (F and OH) on the surface of MXenes, their surface behaves as a negatively charged MXene.48 Therefore, due to the negatively charged surface of MXenes, some metal cations are easily intercalated between the MXene layers due to electrostatic interactions.49 This pillared process helps to rectify the restacking issue among the MXene layers, which restricts the cyclic stability of MXenes. Pillared intercalated clays (PILCs) are the most common and important examples of the pillaring effects in nanostructures due to their applications in separation and catalysis.50,51 PILCs show a large interlayer space due to the stable pillaring effect among the layers of clays.52
Luo et al. prepared hybrid electrodes by using Ti3C2 MXenes for high-performance lithium-ion capacitors and a sodium metal anode, whereby they obtained better results compared to pure Ti3C2 MXene.53,54 Maughan et al. also synthesized the silica-pillared Mo2TiC2 MXene, which shows better performance for Li- and Na-ion batteries, and also synthesized in situ pillared MXene for zinc-ion hybrid capacitor applications.55,56
Inspired by the above-mentioned idea, we prepared the Sn-intercalated nanostructure (Sn@Mo2TiC2) with improved electronic conductivity, large surface area, and good ion-diffusion properties. The CTAB surfactant was used for pre-intercalation because Mo2TiC2 does not form a homogeneous solution in deionized water due to its high surface resistance, while the pre-intercalated long-tailed CTA+ cation provides an easy way to intercalate Sn2+ among the layers of Mo2TiC2 MXenes and in the presence of surfactant, and the absorbed Sn2+ spread uniformly among the layers of Mo2TiC2 nanocomplex, whereas without the surfactant it was difficult to intercalate Sn2+ on MXene nanosheets.57 The Sn2+-intercalated Mo2TiC2 offers more surface area due to the large interlayer space that helps to store a larger number of charges among the 2D gallery heights of the MXenes. Meanwhile, in the presence of Sn2+-intercalated Mo2TiC2 hybrid (Sn@Mo2TiC2), the surface and layered structure of the MXene became more conductive. Another important advantage of Sn2+ pillaring among the layers of MXenes was the volume expansion, which further enhances the electrochemical stability by rectifying the restacking issue among the sheets and provides more active sites for the storage of charges even at high scan rates.53 The prepared electrode for supercapacitor applications has a high cyclic stability and specific capacitance value as compared to pure Mo2TiC2 MXene.
Experimentation
The whole process used for the synthesis of the Sn@Mo2TiC2 nanostructure was like that reported by Luo et al.53,54 with some modifications, as described in Fig. 1.
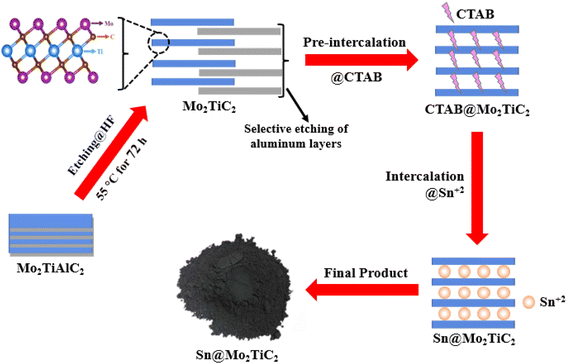 |
| Fig. 1 Schematic diagram for the synthesis of the Sn@Mo2TiC2 nanohybrid. | |
Synthesis of Mo2TiC2 MXene
MAX phase Mo2TiAlC2 (200 mesh) powder was purchased from Yiyi Technology (Jilin Province Yiyi Technology Co., Ltd, Jilin, China) and directly used for the synthesis of Mo2TiC2 MXene through a conventional method. For this purpose, 1 g of MAX powder was added into 10 ml 50% concentrated solution of hydrofluoric acid (HF) and the mixture was continuously stirred in a Teflon beaker at 250 rpm for 72 h at 55 °C. The Mo2TiC2 obtained through this process was centrifuged and washed with deionized water several times at 3500 rpm until the pH reached 6, and finally, the powder was collected by vacuum filtration and dried under vacuum at 60 °C for 24 h.
Synthesis of the Sn@Mo2TiC2 hybrid
For the preparation of the Sn@Mo2TiC2 nanostructure, 200 mg Mo2TiC2 was stirred in 40 ml CTAB (0.150 wt%) solution for 24 h at room temperature. Then SnCl2–2H2O (Sinopharm Chemical Reagent Co. Ltd.) was added (13.536 mg) to prepare 1.5 mM solution and again stirred for 12 h under the same conditions. Finally, the product collected was rinsed with water and dried under a vacuum at 60 °C for another 24 h.
Material characterization
To study the structural properties of the nanohybrid, an X-ray diffractometry system (XRD, Bruker, D8 Advance) with a monochromatic Cu-Kα source and wavelength of 1.54 Å was used. The thermal stability of the nanohybrid was determined by thermogravimetric analysis (TGA, SDT650) and an ultraviolet–visible (UV–vis, Lambda 365 PerkinElmer) absorption test was performed to estimate the band gap of the nanostructure. To study the surface area and average pore volume of the hybrid material, gas adsorption–desorption analysis based on the Brunauer–Emmett–Teller (BET, Quantachrome NovaWin) method was performed. The morphological properties of the materials were analyzed by scanning electron microscopy (SEM; Hitachi s-4800 FEG), while energy dispersive X-ray spectroscopy (EDX) was used to measure the elemental composition of the material. Fourier transform infrared (FTIR, Bruker Alpha) spectrometry was used to study the chemical structure of the samples. Raman spectrometry (Thermo Renishaw, He–Ne laser, λ = 532 nm) was employed to study the chemical bond structure of the nanohybrid.
Electrochemical measurements
Electrochemical measurements were determined by using a potentiostat electrochemical workstation (Gamry 1010B) three-electrode system, in the presence of 1 M KOH electrolyte solution. The counter and reference electrodes consisted of platinum wire and Ag/AgCl, respectively. The working electrode was prepared by the following steps. To study the electrochemical properties of our hybrid electrode, we used Ni foam as a current collector. For that purpose, we made a slurry of Sn@Mo2TiC2 nanohybrid by using 75% active material, 15% carbon black, 10% polyvinylidene fluoride (PVDF), and N-methylpyrrolidone (NMP) used as a solvent. The as-prepared slurry was coated on the Ni foam of a 1 cm × 1 cm current collector, which was prewashed sequentially with hydrochloric acid, ethanol, and deionized water by using a sonicator. Finally, the Ni foam electrode was dried in a vacuum oven overnight and pressed under 5 Mpa pressure for 10 s. Cyclic voltammetry (CV) tests were performed at different scan rates to study the electrochemical behaviors of the samples and the gravimetric capacitance. The long-term cyclic performances of the electrodes were studied by galvanostatic charge–discharge (GCD) tests at a current density of 10 A g−1 for 10
000 cycles. To study the charge-transfer resistance and diffusion coefficient of the samples, electrochemical impedance spectroscopy (EIS) measurements were performed in the frequency range of 20 kHz to 10 mHz at open circuit voltage (OCV), with a sinusoidal signal of 10 mV.
Results and discussion
The X-ray diffraction (XRD) patterns of the MAX phase, MXene, and Sn-intercalated MXene are presented in Fig. 2a. The results obtained from the XRD data were in good agreement with the earlier reports, which evidenced the successful synthesis of Mo2TiC2 MXene from the Mo2TiAlC2 MAX phase.58,59 The peak values of the planes (002), (004), and (006) in Mo2TiC2 were shifted toward lower diffraction angles of 6.8°, 13.6°, and 18.9° compared to the parent MAX phase (9.4°, 19°, and 28.6°), which signified an increase in the c-lattice parameter (c-LP) due to the surface functional groups and adsorption of water molecules between the Mo2TiC2 layers.60
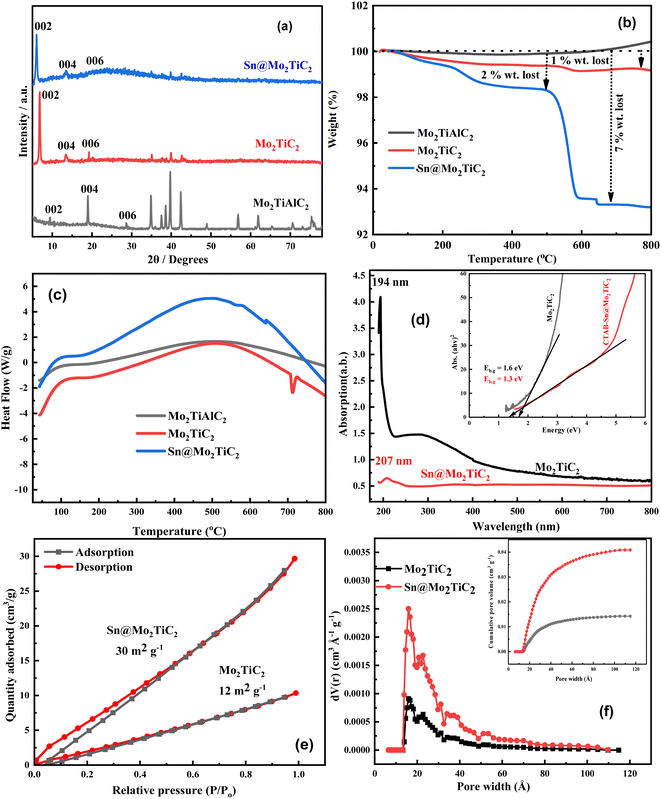 |
| Fig. 2 (a) XRD plots for Mo2TiAlC2, Mo2TiC2, and the Sn@Mo2TiC2 nanohybrid, (b) TGA plots for Mo2TiAlC2, Mo2TiC2, and Sn@Mo2TiC2, (c) DSC curves for Mo2TiAlC2, Mo2TiC2, and Sn@Mo2TiC2, (d) UV-visible absorption spectra and inset Tauc plots for both Mo2TiC2 and Sn@Mo2TiC2, (e) N2 adsorption–desorption plots at 77 k for Mo2TiC2 and the Sn@Mo2TiC2 nanohybrid, (f) pore-size distribution, (inset cumulative pore volume graph past) vs. the pore width calculated through the NL DFT method for both the samples Mo2TiC2 and Sn@Mo2TiC2. | |
The main peak of the 002 plane was further shifted to a lower angle from 6.8° to 6.0° in the case of Mo2TiC2 MXene to Sn@Mo2TiC2, which presented that Sn2+ intercalation enhanced the c-LP from 25.8 to 29.3 Å and d-spacing from 1.29 nm to 1.47 nm. The increase in the c-LP and d value of the 002 plane for Sn@Mo2TiC2 confirmed the successful Sn2+ intercalation among the layers of Mo2TiC2 MXene nanosheets. The value of the peak intensity was also observed to decrease for the (002) plane in the case of the Sn@Mo2TiC2 hybrid compared to pure Mo2TiC2 and the 006 peaks also vanished, which also suggested the amorphous nanocomplex formed on Mo2TiC2. The interaction between Mo2TiC2 was further confirmed from the peak split in the FTIR spectra of pure Mo2TiC2 and Sn@Mo2TiC2, as shown in Fig. 4a.
Thermogravimetric (TGA) analysis was performed to investigate the thermal properties of the materials to estimate the thermal conductivity, thermal stability, and thermal expansion. TGA measurements for Mo2TiAlC2, Mo2TiC2, and Sn@Mo2TiC2 were carried out in the range from room temperature (RT) to 800 °C in the presence of N2 gas, as shown in Fig. 2b. The MAX phase was thermally stable throughout the temperature range, except for a little weight gain in the range of 600 °C to 800 °C, which may have been due to the selective oxidation of Al while heating in N2 in the presence of a minor oxygen content.61 The Mo2TiC2 MXene was also found to be thermally stable throughout the temperature range (RT to 800 °C), except for a slight gradual decrease in weight of up to 1%, which was due to the unstable surface functional groups, like –F, –O, and –OH. Sn@Mo2TiC2 was also heated in an N2 environment in the same temperature range and an initial weight loss of about 2% was observed between 200 °C to 500 °C, which may have been due to the removal of the CTAB surfactant. A further rapid weight loss of up to 5% was also observed between the temperature range of 500 °C to 590 °C, after which it remained stable.
This was the temperature range where the intercalated MXene showed the worst resistance to thermal degradation. However, most reported MXene nanostructures are stable only up to 350 °C and so our material is thermally more stable.62,63 The results of the TGA were strongly consistent with the XRD data, which confirmed the successful intercalation of the amorphous nanocomplex on Mo2TiC2 MXene.
The differential scanning calorimetry (DSC) curves for Mo2TiAlC2, Mo2TiC2, and Sn@Mo2TiC2 are also shown in Fig. 2c. It was observed that all the samples showed their initial peak around 110 °C, which suggests that the change in mass rate happened in all the samples at that point due to the loss of the minor moisture content among the layered structures and a relative endothermic heat flow reaction occurred. A similar kind of large peak was observed in the DSC data around 500 °C in all the samples, but it was more prominent in the case of Mo2TiC2 and Sn@Mo2TiC2 because the rate of mass loss was more significant in the Mo2TiC2 and Sn@Mo2TiC2 structures as compared to the very small mass gain rate in Mo2TiAlC2, which further indicated that a large endothermic heat flow occurred among the material structures due to the change in the mass loss rate. As we already discussed in the TGA results, the mass loss was due to the unstable surface termination groups and low thermal degradation resistance, respectively, at high temperature.59
UV-vis spectroscopic analysis was performed to study the optical properties of our structures. The results are shown in Fig. 2d, which exhibit that the peak values for the absorption of Mo2TiC2 and Sn@Mo2TiC2 were obtained at 194 and 207 nm, respectively. The shift in peak positions identified that the band gap was reduced after adding Sn2+ in MXene, which might be due to some new states' formation between the valence and conduction bands. The inset Tauc plot curve was also plotted to calculate the value of the band gaps of Mo2TiC2 and Sn@Mo2TiC2, which varied from 1.6 eV (for pristine MXene) to 1.3 eV (for Sn-intercalated MXene). This result is an indication of the successful intercalation of Sn2+.64
To study the effect of Sn2+ on the surface area of the MXene, BET analysis was performed in the presence of N2 isotherm at 77 K. Fig. 2e shows the results of the adsorption–desorption data for both the samples Mo2TiC2 and Sn@Mo2TiC2. Fig. 2e shows that the surface area for the nanohybrid (Sn@Mo2TiC2) increased up to 30 m2 g−1 compared to 12 m2 g−1 for pure Mo2TiC2 MXene, which indicated that the hybrid material had a more porous nature compared to pure MXene. Fig. 2f shows the pore-width distribution, which was calculated through the non-local density functional theory (NL DFT) method and inset plot of the cumulative pore volume vs. pore width.65 The results of the inset plot of Fig. 2f show that the pore volume increased from 0.015 to 0.042 cm3 g−1, which was again consistent with the BET results (whereby the surface area increased from 12 to 30 m2 g−1). Hence the increase in porosity of the Sn@Mo2TiC2 was increased due to the interaction of Sn2+ with the Mo2TiC2 nanosheets. These results were also consistent with the XRD data of Mo2TiC2 and Sn@Mo2TiC2, where a shift in diffraction angle toward the lower side was observed in the main peak of the 002 plane for both the samples, and as a result the c-LP values were increased from 2.58 to 2.93 nm, respectively (Mo2TiC2 to Sn@Mo2TiC2). So, the increase in c-LP also became the cause of the increase in d value from 1.29 to 1.47 nm for Mo2TiC2 to Sn@Mo2TiC2. Finally, we can say that the increase in the surface area of our hybrid material was basically due to the interaction of Mo2TiC2 with Sn2+.
The surface morphology of the Mo2TiC2 MXene and Sn@Mo2TiC2 was studied by scanning electron microscopy (SEM), as shown in Fig. 3 a and b. Both the SEM micrographs of MXene and the hybrid material Sn@Mo2TiC2 (1 and 2 μm) showed a layered nature, respectively. Fig. 3b shows presence of Sn2+ in the prepared sample. In addition, Sn2+ was noted in the spectra, confirming its presence in the hybrid (ESI Fig. S2†).
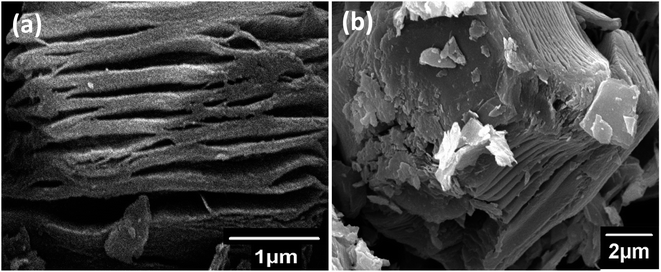 |
| Fig. 3 Scanning electron microscopy (SEM) images of both the samples (a) Mo2TiC2 MXene image taken at 1 μm, (b) Sn@Mo2TiC2 hybrid image taken at 2 μm. | |
Moreover, the SEM image for the hybrid material showed that the Sn2+ intercalation did not destroy the layered structure of MXene in Sn@Mo2TiC2, which was also confirmed by the XRD data (Fig. 2a). SEM energy dispersive X-ray spectroscopy (EDX) was also performed to confirm the Sn2+ content in the Sn@Mo2TiC2 hybrid in (wt%) percentage along with the EDX spectra. The EDX spectra and elemental composition of both the samples along with their SEM images are displayed in Fig. S1 and S2 in the (ESI) data,† where the small peaks corresponding to aluminum showed the presence of a small fraction of the unreacted MAX phase in the MXene sample. The unfitted peak to the left of the Mo peak is a continuum (background). The EDX results also confirmed the presence of F and O surface termination groups.
FTIR spectroscopy was used to confirm the successful Sn2+-exchange absorption phenomenon on the Mo2TiC2 MXene. Fig. 4a shows the FTIR spectra of Mo2TiC2 before and after treating it with SnCl2·2H2O solutions. The peaks observed at 2318, 2100, and 1996 cm−1 belonged to the stretching vibration of the bonds C
O, C
C, and C
C, respectively, as shown in Fig. 4a.66 The main two peaks at 615 and 560 cm−1 were due to the deformation molecular vibrations of Ti–O and Mo–O in the case of the Mo2TiC2 sample. However, a new peak appeared at 750 cm−1 in the case of Sn@Mo2TiC2, which was due to Sn–O bond vibrations.37 Basically, the split in the peak around 750 was due to the successful intercalation of the amorphous nanocomplex among the layers of MXene.
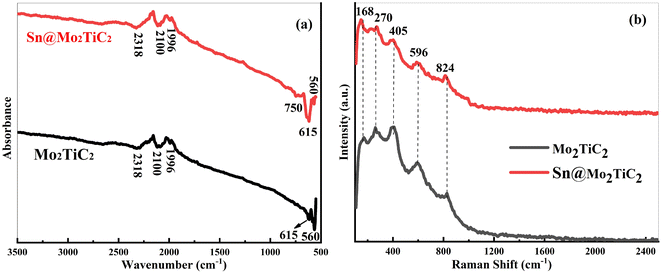 |
| Fig. 4 (a) FTIR spectra of Mo2TiC2 and the Sn@Mo2TiC2 nanohybrid, (b) Raman spectra of Mo2TiC2 and the Sn@Mo2TiC2 nanohybrid. | |
Fig. 4b shows the Raman spectra of Mo2TiC2 and the Sn@Mo2TiC2 MXene nanohybrid. The peaks that appeared at 168, 270, 405, 596, and 824 cm−1 closely matched with the previously published data and no significant difference appeared in the peak positions observed after interaction with Sn2+.55 The peak that appeared around 168 cm−1 was due to the Eg mode of vibrations of both Mo and Ti atoms in the presence of the –O terminated Mo2TiC2 MXene. The peak at around 270 cm−1 appeared due to the Eg vibration due to oxygen. The other three peaks at 405, 596, and 824 cm−1 suggested the vibrations of carbon atoms present in the MXene structure. In literature, Kim et al. assigned the peak that appears at 405 cm−1 to –O vibrations, but Chen et al. assigned it to –C vibrations.67,68 Fig. 4a also shows that no new peak appeared after the intercalation of Sn2+, which is again evidence that the amorphous nanocomplex formed on Mo2TiC2 due to interaction with Sn2+, so the results of the Raman spectra were also consistent with the XRD results.
Cyclic voltammetry
The electrochemical performance was studied by cyclic voltammetry. Fig. 5a and b show the CV plots for Mo2TiC2 MXene and Sn@Mo2TiC2 at different scan rates of 2, 5, 10, 20, 50, 100, 150, and 200 mV s−1 under a specific potential window. Two peaks were observed at all scan rates, namely due to the electrochemical insertion of K+ ions (cathodic peak) and the extraction of K+ ions called the anodic peak, indicating the successful electrochemical process. The oxidation and reduction reactions showed some specific values of voltages for all the scan rates. The average oxidation and reduction potential for Mo2TiC2 and Sn@Mo2TiC2 were 0.41, 0.42 V and 0.30, 0.25 V, respectively, suggesting the presence of a strong faradaic phenomenon in both electrodes.69 These CV curves indicated that by increasing the scan rate, the value of the current increased, according to the relation in the ESI (SI1†), but the value of the electrochemical capacitance decreased due to the lesser interaction of the electrolyte with the electrode at high scan rates. Moreover, the value of gravimetric capacitance was observed to be higher in Sn@Mo2TiC2 because Sn2+ interaction with MXene provided sufficiently high electroactive sites for easy electrolyte transportation and enhanced the effective surface area for better electrochemical kinetics at all the scan rates compared to the pristine MXene. Hence, the highest value of gravimetric capacitance was observed for both samples at a scan rate of 2 mV s−1, which was 212 F g−1 for Mo2TiC2 and 670 F g−1 for Sn@Mo2TiC2. These results show that the value of specific capacitance increased for the Sn-intercalated electrode, which may be due to its large charge-storage ability owing to the pillaring effect. The formula used to measure the values of specific capacitance is given in the ESI(SI2†).70,71
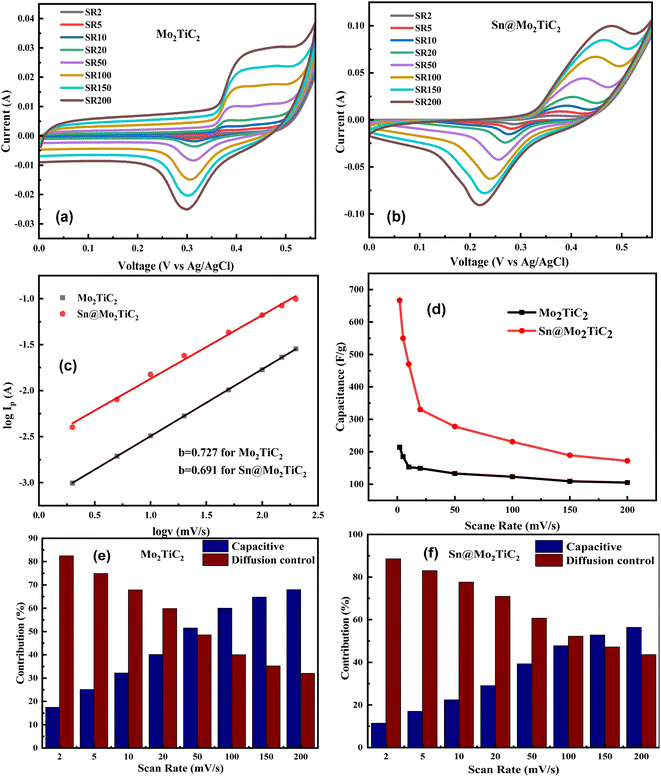 |
| Fig. 5 CV plots using 1 M KOH electrolyte for (a) Mo2TiC2 MXene and (b) the Sn@Mo2TiC2 nanohybrid at different scan rates. (c) Combine b value plots for both Mo2TiC2 and the Sn@Mo2TiC2 nanohybrid. (d) Scan rate vs. capacitance the F g−1 plot for both Mo2TiC2 and the Sn@Mo2TiC2 nanohybrid, (e) plots between the scan rate and percentage contribution of Mo2TiC2 for the capacitive and diffusion control processes, (f) plots between the scan rate and percentage contribution of the Sn@Mo2TiC2 nanohybrid for the capacitive and diffusion control processes. | |
Fig. 5c shows the plot between log
Ip and log
v to measure the b values for Mo2TiC2 and Sn@Mo2TiC2, where Ip represents the peak current, and v is used for the scan rate (SI3†). Generally, the value of b = 1 or 0.5 (where b = 1 represents the capacitive process and b = 0.5 indicates the diffusion control dominated process), but most of the time, the value of the b parameter lies between 1 and 0.5, which is evident of a combined charge-storage mechanism.
Here we measured the value of b for both the samples Mo2TiC2 and Sn@Mo2TiC2 (b = 0.727 and b = 0.691), respectively, as shown in Fig. 5c, where the slightly low b value of our as-synthesized hybrid electrode indicated that it was a more diffusion control process as compared to Mo2TiC2, which indicated that there were some more active sites available for charge storage in the hybrid material due to its porous nature. Meanwhile, the value of b (0.69) ranged between 1 and 0.5 for the hybrid electrode Sn@Mo2TiC2, revealing that the electrochemical reaction kinetics were associated with both charge-storage mechanisms for the diffusion control process and capacitive-like effects as well. Fig. 5d shows the plot between the specific capacity (F g−1) and scan rate (mV s−1) for Mo2TiC2 and Sn@Mo2TiC2. The values of the hybrid electrode were found to be higher at all the scan rates in comparison to Mo2TiC2 MXene. Here we see that the specific capacities of both electrodes decreased at high scan rates, which was due to the limitations of the reaction kinetics.
To study the combined charge storage mechanism further due to the capacitive process and diffusion control, we used the relation (SI4) in the ESI† where the total peak current Ip is divided into k1v (capacitive process) and k2v0.5 (diffusion control process), which represent the contribution of the charge-storage mechanism in both ways. Furthermore, the values of k1 and k2 could be determined by plotting a graph between Ip v−0.5 and v0.5,72 as given in SI5 in the ESI.† The slope represents the k1 value and the point of the intercept defines the value of k2. We measured all these values for both the samples of Mo2TiC2 MXene and Sn@Mo2TiC2. After calculating the percentage contributions of the diffusion and capacitive process values for both Mo2TiC2 and Sn@Mo2TiC2 at all scan rates (2 to 200 mV s−1), we plotted the results in Fig. 5 e and f.
The percentage contributions of the diffusion and capacitive process of Mo2TiC2 MXene and Sn@Mo2TiC2 showed that the value of diffusion control was high at low scan rates, which was due to the maximum interaction of the electrolyte ions with the electrodes as compared to at a high scan rate. By comparing the results of the diffusion control and capacitive process contributions for both the samples Mo2TiC2 MXene and Sn@Mo2TiC2, it could be concluded that the value for diffusion control was improved in the case of the hybrid electrode Sn@Mo2TiC2 from 82% to 88% and 32% to 43% at scan rates of 2 to 200 mV s−1 compared to Mo2TiC2. This evidence indicates that the electrochemical reaction kinetics dominated due to the diffusion control process in our as-synthesized hybrid electrode. The increase in the diffusion process contribution was also observed in the Sn2+-treated Mo2TiC2, which was due to the larger surface area, large spacing among the layers of MXene, and high electronic conductivity of the hybrid electrode.
Fig. 6a shows the higher values of the anodic and cathodic peak currents at all scan rates for the Sn@Mo2TiC2 electrode as compared to Mo2TiC2 MXene, which suggests that the charge-storage ability or insertion and extraction of ions were higher in the hybrid electrode due to its good electrochemical activity. The uniform growth of Sn2+ on the surface of Mo2TiC2 proved helpful to produce more active sites and enhance the contact area with the electrolyte solution to improve the electrochemical performance and kinetics of the redox reaction. Fig. 6b presents a comparison of the already reported data with our hybrid electrode, where the x-axis presents the values of different electrolyte solutions used for the various electrochemical studies along with their molar concentrations and the y-axis represents the specific capacitance in F g−1. So, our as-synthesized hybrid electrode showed the best charge-storage capacity of about 670 F g−1.70,73–79 The further extended details of the comparison data are listed in Table 1 in the ESI† which includes the details of the materials and electrolytes used for electrochemical testing along with their achieved specific capacitance.
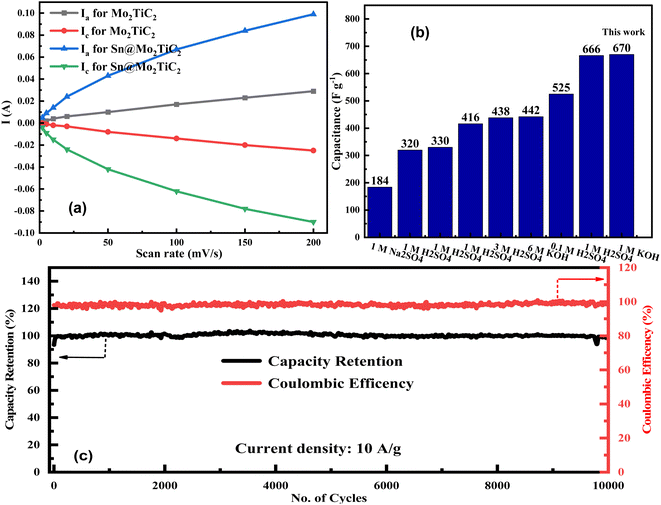 |
| Fig. 6 (a) Anodic and cathodic peak currents vs. the scan rates calculated for both Mo2TiC2 and Sn@Mo2TiC2 electrodes. (b) Comparison of our hybrid Sn@Mo2TiC2 electrode capacitance with the reported data in the literature. (c) Long-term cyclic performance of the Sn@Mo2TiC2 nanohybrid at current density of 10 A g−1. | |
Table 1 “d” and “c” values calculated for the 002 plane
Sample |
Plane hkl |
Angle (2θ) |
d value (nm) |
c-LP (Å) |
Mo2TiAlC2 |
002 |
9.4 |
0.94 |
18.8 |
Mo2TiC2 |
002 |
6.8 |
1.29 |
25.8 |
Sn@Mo2TiC2 |
002 |
6.0 |
1.47 |
29.3 |
Fig. 6c shows the long-term cyclic performance of our hybrid electrode Sn@Mo2TiC2 for 10
000 cycles at a current density of 10 A g−1 (with a maximum capacitance of 184 F g−1). The value of capacity retention was observed to remain stable at up to 99% throughout the 10
000 cycles. The intercalated hybrid electrode was observed to be stable electrochemically, which was due to the fast electrolyte transport (through the excessive conductive channels produced due to Sn intercalation) within the electrode, which enhanced its overall capacity retention with no change in the coulombic efficiency of 100%. The values of specific capacitance for our hybrid electrode remained high at all scan rates compared to pristine Mo2TiC2 MXene, which demonstrated the enhanced charge-storage capacity of our hybrid material as compared to pure MXene.
The electrochemical conductivity of both samples was examined in 1 M KOH through EIS analysis, as shown in Fig. 7a. The Nyquist plot EIS data were fitted with the Randle plus Warburg series model, and the results in the high-frequency range are also shown in Fig. 7a. Herein, the equivalent circuit, shown in the inset of Fig. 7b, consisted of the charge-transfer resistance Rct, solution resistance Rs, Warburg impedance Zw related to the diffusion of K+ ions, and CPE (phase constant element). Both the solution resistance Rs and Warburg impedance Zw, related to the electrolyte solution and diffusion of electroactive ions, were the same for all the electrodes, but the charge-transfer resistance Rct and CPE were directly influenced by the properties of the hybrid electrode, because they depended on the conductive properties of the as-prepared electrodes.73 The values of charge-transfer resistance Rct measured for Mo2TiC2 and Sn@Mo2TiC2 were 575 and 292 Ω, respectively. The large difference in the charge-transfer resistance (283 Ω) was basically evidence of the enhanced diffusion process and fast reaction kinetics due to the high electronic conductivity of our as-synthesized hybrid electrode Sn@Mo2TiC2. Similarly, the value observed for CPE for both the samples Mo2TiC2 and Sn@Mo2TiC2 also increased from 0.0168 to 0.0246 F, respectively.
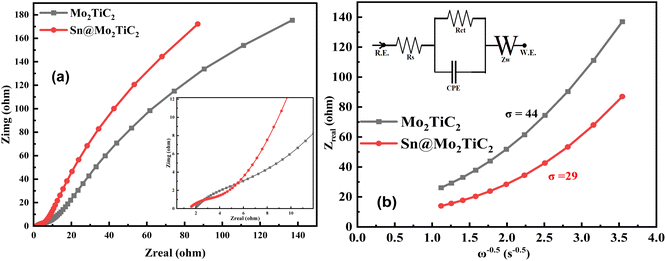 |
| Fig. 7 (a) Nyquist plot of the EIS spectra and inset of the high-frequency region for both Mo2TiC2 and Sn@Mo2TiC2. (b) Real impedance Zreal vs. ω−0.5 data and in the set equivalent circuit provided for fitting the EIS data for both Mo2TiC2 and Sn@Mo2TiC2. | |
Fig. 7b shows the plot between the real impedance and frequency ω−0.5, with the plot basically drawn to measure the value of the diffusion coefficient. For that purpose, eqn (SI6) in the ESI† shows the relationship between the real impedance Zreal and angular frequency (ω) in the low-frequency regions.80–82 Here, σ belongs to the Warburg factor, which could be calculated directly from the slope of Fig. 7b. The coefficient of diffusion for K+ ions was calculated through eqn (SI7) in the ESI† which clarifies the diffusion coefficient is directly proportional to R (gas constant) and T (absolute temperature) and inversely proportional to the A (surface area), n (no. of electrons), F (Faraday constant), C (solution concentration), and D (diffusion coefficient). Here in eqn (SI7) in the ESI† all the factors are constant except “σ”, so the value of the diffusion coefficient increases as the σ decreases, so the measured values of σ for Mo2TiC2 and Sn@Mo2TiC2 were 44 and 29, respectively. Because the value of σ for Sn@Mo2TiC2 was low, the diffusion value of Sn@Mo2TiC2 was high compared to Mo2TiC2; hence the results were also consistent with the EIS Nyquist spectra and cyclic voltammetry data.
Conclusion
We successfully synthesized a Sn@Mo2TiC2 hybrid via the facile liquid-phase pre-intercalated cetyltrimethylammonium bromide (CTAB) method for obtaining enhanced electrochemical properties compared to MXene. Our synthesis method proved successful to enhance the gallery height of Mo2TiC2 by increasing the c-LP in the case of the hybrid (c-LC increased from 25.8 to 29.3 Å) and no additional peak was observed due to Sn2+ intercalation, which confirmed the amorphous nanocomplex structure. The TGA results confirmed that our hybrid material was stable up to 500 °C and had a reduced band gap, identifying the interaction between Mo2TiC2 and Sn2+, which was studied through UV-vis spectroscopy. The effective surface area of our synthesized nanostructure was also increased from 12 to 30 m2 g−1 compared to Mo2TiC2 MXene. The EDX spectra confirmed the composition of Sn2+ in Mo2TiC2 and the FTIR spectra also confirmed the presence of Sn2+ on Mo2TiC2 due to the split in the peak at 750 cm−1. The CV results exhibited the improved electrochemical properties of our hybrid electrode, which was tested for supercapacitor applications. The value of the specific capacitance increased from 212 to 670 F g−1 in the case of Mo2TiC2 to the Sn@Mo2TiC2 hybrid and the measured b value also showed the diffusion control mechanism was dominant in the as-prepared hybrid electrode. The cyclic performance of Sn@Mo2TiC2 was also measured at a current density of 10 A g−1, which was also observed to be stable at up to 99% throughout the 10
000 cycles. The EIS data also showed the low charge-transfer resistance and high diffusion coefficient of the prepared hybrid electrode. The use of MXenes as highly porous and stable electrodes opens the way to construct efficient electronic energy-storage devices.
Author contributions
Irfan Ali managed the whole experimentation and paper writing process; Zulqarnain Haider helped in EIS data collection; Syed Rizwan Hussain supervised the whole project, helped in paper writing, and conceived the research idea.
Conflicts of interest
The authors declared there is no competing interest.
Acknowledgements
The authors thank the Higher Education Commission (HEC) of Pakistan for providing research funding under Project No. 20-14784/NRPU/R&D/HEC/2021.
References
- L. Grigorios Kyriakopoulos and G. Arabatzis, Electrical Energy Storage Systems in Electricity Generation: Energy Policies, Innovative Technologies, and Regulatory Regimes, Renewable Sustainable Energy Rev., 2016, 56(C), 1044–1067 CrossRef.
- Y. Zhang, K. Hu and J. Ren, et al., A Sandwich-Like Si/SiC/Nanographite Sheet as a High-Performance Anode for Lithium-Ion Batteries, Dalton Trans., 2019, 48, 17683–17690 RSC.
- L. Wu, J. Zheng and L. Wang, et al., PPy-Encapsulated SnS2 Nanosheets Stabilized by Defects on a TiO2 Support as a Durable Anode Material for Lithium-Ion Batteries, Angew. Chem., Int. Ed., 2019, 58, 811 CrossRef CAS PubMed.
- Y. Yu, L. Gu, C. Zhu, S. Tsukimoto, P. A. van Aken and J. Maier, Reversible Storage of Lithium in Silver-Coated Three-Dimensional Macroporous Silicon, Adv. Mater., 2010, 22, 2247–2250 CrossRef CAS PubMed.
- J. Zhuang, X. Xu, G. Peleckis, W. Hao, S. X. Dou and Y. Du, Silicene: a Promising Anode for Lithium-Ion Batteries, Adv. Mater., 2017, 29, 1606716 CrossRef PubMed.
- Y. Zhang, Y. Jiang, Y. Li, B. Li, Z. Li and C. Niu, Preparation of Nanographite Sheets Supported Si Nanoparticles by In Situ Reduction of Fumed SiO2with Magnesium for Lithium-Ion Battery, J. Power Sources, 2015, 281, 425 CrossRef CAS.
- Y. Huang, C. Zhu and S. Zhang, et al., Ultrathin Bismuth Nanosheets for Stable Na-Ion Batteries: Clarification of Structure and Phase Transition by In Situ Observation, Nano Lett., 2019, 19, 1118 CrossRef CAS PubMed.
- M. Armand and J. M. Tarascon, Building Better Batteries, Nature, 2008, 451, 652–657 CrossRef CAS PubMed.
- M. S. Whittingham, Lithium Batteries and Cathode Materials, Chem. Rev., 2004, 104, 4271 CrossRef CAS.
- J. Luo, C. Wang, H. Wang, X. Hu, E. Matios, X. Lu, W. Zhang, X. Tao and W. Li, Pillared MXene with Ultralarge Interlayer Spacing as a Stable Matrix for High Performance Sodium Metal Anodes, Adv. Funct. Mater., 2019, 29, 1805946–1805958 CrossRef.
- A. Geim and K. Novoselov, The Rise of Graphene, Nat. Mater., 2007, 6, 183–191 CrossRef CAS PubMed.
- B. Radisavljevic, A. Radenovic, J. Brivio, V. Giacometti and A. Kis, Single-Layer MoS2 Transistors, Nat. Nanotechnol., 2011, 6, 147–150 CrossRef CAS.
- K. S. Novoselov, D. Jiang, F. Schedin, T. J. Booth, V. V. Khotkevich, S. V. Morozov and A. K. Geim, Two-Dimensional Atomic Crystals, Proc. Natl. Acad. Sci. U. S. A., 2005, 102, 10451–10453 CrossRef CAS.
- R. Ma and T. Sasaki, Nanosheets of oxides and Hydroxides: Ultimate 2D Charge-Bearing Functional Crystallites, Adv. Mater., 2010, 22, 5082–5104 CrossRef CAS PubMed.
- J. N. Coleman, M. Lotya, A. Neill, S. D. Bergin, P. J. King, U. Khan, K. Young and A. Gaucher, et.al., Two-Dimensional Nanosheets Produced by Liquid Exfoliation of Layered Materials, Science, 2011, 331, 568–571 CrossRef CAS PubMed.
- J. B. Wu, R. Q. Guo, X. H. Huang and Y. Lin, Construction of Self-Supported Porous TiO2/NiO Core/Shell Nanorod Arrays for Electrochemical Capacitor Application, J. Power Sources, 2013, 243, 317–322 CrossRef CAS.
- Q. Guo, N. Chen and L. Qu, Two-Dimensional Materials of Group-IVA Boosting the Development of Energy Storage and Conversion, Carbon Energy, 2020, 2, 54–71 CrossRef.
- J. Ren, Y. Huang and H. Zhu, et.al., Recent Progress on MOF-Derived Carbon Materials for Energy Storage, Carbon Energy, 2020, 2, 176–202 CrossRef CAS.
- M. Naguib, M. Kurtoglu, V. Presser, J. Lu, J. J. Niu, M. Heon, L. Hultman, Y. Gogotsi and M. W. Barsoum, Two-Dimensional Nanocrystals Produced by Exfoliation of Ti3AlC2, Adv. Mater., 2011, 23, 4248–4253 CrossRef CAS PubMed.
- M. Naguib, O. Mashtalir, J. Carle, V. Presser, J. Lu, L. Hultman, Y. Gogotsi and M. Barsoum, Two-Dimensional Transition Metal Carbides, ACS Nano, 2012, 6, 1322–1331 CrossRef CAS PubMed.
- M. R. Lukatskaya, O. Mashtalir, C. E. Ren, Y. Agnese, P. Rozier, P. L. Taberna, M. Naguib, P. Simon, M. W. Barsoum and Y. Gogotsi, Cation Intercalation and High Volumetric Capacitance of Two-Dimensional Titanium carbide, Science, 2013, 341, 1502–1505 CrossRef CAS PubMed.
- M. Naguib, J. Halim, J. Lu, K. M. Cook, L. Hultman, Y. Gogotsi and M. W. Barsoum, New Two-Dimensional Niobium and Vanadium Carbides as Promising Materials for Li-Ion Batteries, J. Am. Chem. Soc., 2013, 135, 15966–15969 CrossRef CAS.
- M. Ghidiu, M. R. Lukatskaya, M. Q. Zhao, Y. Gogotsi and M. W. Barsoum, Conductive Two-Dimensional Titanium Carbide 'Clay' with High Volumetric Capacitance, Nature, 2014, 516, 78–81 CrossRef CAS PubMed.
- Z. Ling, C. E. Ren, M. Q. Zhao, J. Yang, J. M. Giammarco, J. S. Qiu, M. W. Barsoum and Y. Gogotsi, Flexible and Conductive MXene Films and Nanocomposites with High Capacitance, Proc. Natl. Acad. Sci. U.S.A., 2014, 111, 16676–16681 CrossRef CAS PubMed.
- O. Mashtalir, M. R. Lukatskaya, M. Q. Zhao, M. W. Barsoum and Y. Gogotsi, Amine-Assisted Delamination of Nb2C MXene for Li-Ion Energy Storage Devices, Adv. Mater., 2015, 27, 3501–3506 CrossRef CAS.
- M. Q. Zhao, C. E. Ren, Z. Ling, M. R. Lukatskaya, C. F. Zhang, K. L. Van Aken, M. W. Barsoum and Y. Gogotsi, Flexible MXene/Carbon Nanotube Composite Paper with High Volumetric Capacitance, Adv. Mater., 2015, 27, 339–345 CrossRef CAS PubMed.
- Q. Tang, Z. Zhou and P. W. Shen, Are MXenes Promising Anode Materials for Li Ion Batteries? Computational Studies on Electronic Properties and Li Storage Capability of Ti3C2 and Ti3C2X2 (X = F, OH) Monolayer, J. Am. Chem. Soc., 2012, 134, 16909–16916 CrossRef CAS PubMed.
- X. Zhu, Z. Cao, W. Wang, H. Li, J. Dong, S. Gao, D. Xu, L. Li, J. Shen and M. Ye, Superior-Performance Aqueous Zinc-Ion Batteries Based on the In Situ Growth of MnO2 Nanosheets on V2CTX MXene, ACS Nano, 2021, 15, 2971–2983 CrossRef CAS.
- M. S. Zhu, Y. Huang, Q. H. Deng, J. Zhou, Z. X. Pei, Q. Xue, Y. Huang, Z. F. Wang, H. F. Li, Q. Huang and C. Y. Zhi, Highly Flexible, Freestanding Supercapacitor Electrode with Enhanced Performance Obtained by Hybridizing Polypyrrole Chains with MXene, Adv. Energy Mater., 2016, 6, 1600969 CrossRef.
- X. Liang, A. Garsuch and L. F. Nazar, Surface Cathodes Based on Conductive MXene Nanosheets for High-Performance Lithium–Sulfur Batteries, Angew. Chem., Int. Ed., 2015, 54, 3907 CrossRef CAS PubMed.
- D. Sun, M. Wang, Z. Li, G. Fan, L.-Z. Fan and A. Zhou, Two-Dimensional Ti3C2 as Anode Material for Li-Ion Batteries, Electrochem. Commun., 2014, 47, 80–83 CrossRef CAS.
- Y. F. Dong, Z. S. Wu, S. H. Zheng, X. H. Wang, J. Q. Qin, S. Wang, X. Y. Shi and X. H. Bao, Ti3C2 MXene-Derived Sodium/Potassium Titanate Nanoribbons for High-Performance Sodium/Potassium ion Batteries with Enhanced
Capacities, ACS Nano, 2017, 11(5), 4792–4800 CrossRef CAS.
- J. Wang, J. Tang, B. Ding, V. Malgras, Z. Chang, X. D. Hao, Y. Wang, H. Dou, X. G. Zhang and Y. Yamauchi, Hierarchical Porous Carbons with Layer-By-Layer Motif Architectures from Confined Soft-Template Self-Assembly in Layered Materials, Nat. Commun., 2017, 8, 15717 CrossRef CAS PubMed.
- C. F. Zhang, B. Anasori, A. Seral-Ascaso, S. H. Park, N. McEvoy, A. Shmeliov, G. S. Duesberg, J. N. Coleman, Y. Gogotsi and V. Nicolosi, Transparent, Flexible, and Conductive 2D Titanium Carbide (MXene) Films with High Volumetric Capacitance, Adv. Mater., 2017, 29, 1702678 CrossRef.
- X. H. Wu, Z. Y. Wang, M. Z. Yu, L. Y. Xiu and J. S. Qiu, Stabilizing the MXenes by Carbon Nanoplating for Developing Hierarchical Nanohybrids with Efficient Lithium Storage and Hydrogen Evolution Capability, Adv. Mater., 2017, 29, 1607017 CrossRef.
- R. T. Wang, S. J. Wang, Y. B. Zhang, D. D. Jin, X. Y. Tao and L. Zhang, Graphene Coupled Ti3C2 MXenes-Derived TiO2 Mesostructure: Promising Sodium-Ion Capacitor Anode with Fast Ion Storage and Long-Term Cycling, J. Mater. Chem. A, 2018, 6, 1017 RSC.
- J. Luo, X. Tao, J. Zhang, Y. Xia, H. Huang, L. Zhang, Y. Gan, C. Liang and W. Zhang, Sn4+ ion Decorated Highly Conductive Ti3C2 MXene: Promising Lithium-Ion Anodes with Enhanced Volumetric Capacity and Cyclic Performance, ACS Nano, 2016, 10, 2491–2499 CrossRef CAS PubMed.
- J. Luo, C. Fang, C. Jin, H. Yuan, O. Sheng, R. Fang, W. Zhang, H. Huang, Y. Gan, Y. Xia, C. Liang, J. Zhang, W. Li and X. Tao, Tunable Pseudocapacitance Storage of MXene by Cation Pillaring for High Performance Sodium-Ion Capacitors, J. Mater. Chem. A, 2018, 6, 7794 RSC.
- B. Li, D. Zhang, Y. Liu, Y. Yu, S. Li and S. Yang, Flexible Ti3C2 MXene-Lithium Film with Lamellar Structure for Ultrastable Metallic Lithium Anodes, Nano Energy, 2017, 39, 654–661 CrossRef CAS.
- H. Liu, C. Y. Duan, C. H. Yang, W. Q. Shen, F. Wang and Z. F. Zhu, A Novel Nitrite Biosensor Based on the Direct Electrochemistry of Hemoglobin Immobilized on MXene-Ti3C2, Sens. Actuators, B, 2015, 218, 60–66 CrossRef CAS.
- F. Wang, C. H. Yang, C. Y. Duan, D. Xiao, Y. Tang and J. F. Zhu, An Organ-Like Titanium Carbide Material (MXene) with Multilayer Structure Encapsulating Hemoglobin for a Mediator-Free Biosensor, J. Electrochem. Soc., 2015, 162, B16–B21 CrossRef CAS.
- F. Shahzad, M. Alhabeb, C. B. Hatter, B. Anasori, S. M. Hong, C. M. Koo and Y. Gogotsi, Electromagnetic Interference Shielding with 2D Transition Metal Carbides (MXenes), Science, 2016, 353, 1137–1140 CrossRef CAS PubMed.
- J. Liu, H. B. Zhang, R. H. Sun, Y. F. Liu, Z. S. Liu, A. G. Zhou and Z. Z. Yu, Hydrophobic, Flexible, and Lightweight MXene Foams for High-Performance Electromagnetic Interference Shielding, Adv. Mater., 2017, 29, 1702367 CrossRef PubMed.
- Q. R. Zhang, J. Teng, G. D. Zou, Q. M. Peng, Q. Du, T. F. Jiao and J. Y. Xiang, Efficient Phosphate Sequestration for Water Purification by Unique Sandwich-like MXene/Magnetic Iron Oxide Nanocomposites, Nanoscale, 2016, 8, 7085–7093 RSC.
- C. E. Ren, K. B. Hatzell, M. Alhabeb, Z. Ling, K. A. Mahmoud and Y. Gogotsi, Charge and Size-Selective Ion Sieving Through Ti3C2Tx MXene Membranes, J. Phys. Chem. Lett., 2015, 6, 4026–4031 CrossRef CAS PubMed.
- K. Rasool, M. Helal, A. Ali, C. E. Ren, Y. Gogotsi and K. A. Mahmoud, Antibacterial Activity of Ti3C2Tx MXene, ACS Nano, 2016, 10, 3674–3684 CrossRef CAS PubMed.
- M. Naguib, M. Kurtoglu, V. Presser, J. Lu, J. Niu, M. Heon, L. Hultman, Y. Gogotsi and M. W. Barsoum, Two-Dimensional Nanocrystals Produced by Exfoliation of Ti3AlC2, Adv. Mater., 2011, 23, 4248–4253 CrossRef CAS PubMed.
- M. Naguib and Y. Gogotsi, Synthesis of Two-Dimensional Materials by Selective Extraction, Acc. Chem. Res., 2015, 48, 128–135 CrossRef CAS PubMed.
- Q. Peng, J. Guo, Q. Zhang, J. Xiang, B. Liu, A. Zhou, R. Liu and Y. Tian, Unique Lead Adsorption Behavior of Activated Hydroxyl Group in Two-Dimensional Titanium Carbide, J. Am. Chem. Soc., 2014, 136, 4113–4116 CrossRef CAS PubMed.
- J. T. Kloprogge, Synthesis of Smectites and Porous Pillared Clay Catalysts, A Review, J. Porous Mater., 1998, 5, 5–41 CrossRef CAS.
- A. Gil, L. M. Gandia and M. A. Vicente, Recent Advances in the Synthesis and Catalytic Applications of Pillared Clays, Catal. Rev., 2000, 42, 145–212 CrossRef CAS.
- J. Cheng, S. Ming Yu and P. Zuo, Horseradish Peroxidase Immobilized on Aluminum-Pillared Interlayered Clay for the Catalytic Oxidation of Phenolic Wastewater, Water Res., 2006, 40, 283–290 CrossRef CAS PubMed.
- J. Luo, W. Zhang, H. Yuan, C. Jin, L. Zhang, H. Huang, C. Liang, Y. Xia, J. Zhang, Y. Gan and X. Tao, Pillared Structure Design of MXene with Ultra-Large Interlayer Spacing for High Performance Lithium-Ion Capacitors, ACS Nano, 2017, 11(3), 2459–2469 CrossRef CAS PubMed.
- J. Luo, C. Wang, H. Wang, X. Hu, E. Matios, X. Lu, W. Zhang, X. Tao and W. Li, Pillared MXene with Ultralarge Interlayer Spacing as a Stable Matrix for High Performance Sodium Metal Anodes, Adv. Funct. Mater., 2019, 29, 1805946 CrossRef.
- P. A. Maughan, L. Bouscarrat, V. R. Seymour, S. Shao, S. J. Haigh, R. Dawson, N. Tapia-Ruiz and N. Bimbo, Pillared Mo2TiC2 MXene for high-power and long-life lithium and sodium-ion batteries, Nanoscale Adv., 2021, 3, 3145–3158 RSC.
- P. A. Maughan, N. T. Ruiz and N. Bimbo, In situ Pillared MXene as a Viable Zinc-Ion Hybrid Capacitor, Electrochim. Acta, 2020, 341, 136061 CrossRef CAS.
- J. Luo, J. Zheng, J. Nai, C. Jin, H. Yuan, O. Sheng, Y. Liu, R. Fang, W. Zhang, H. Huang, Y. Gan, Y. Xia, C. Liang, J. Zhang, W. Li and X. Tao, Atomic Sulfur Covalently Engineered Interlayers of Ti3C2 MXene for Ultra-Fast Sodium-Ion Storage by Enhanced Pseudocapacitance, Adv. Funct. Mater., 2019, 29, 1808107 CrossRef.
- B. Anasori, M. R. Lukatskaya and Y. Gogotsi, 2D Metal Carbides and Nitrides (MXenes) for Energy Storage, Nat. Rev. Mater., 2017, 2, 16098 CrossRef CAS.
- H. Kim, B. Anasori, Y. Gogotsi and H. N. Alshareef, Thermoelectric Properties of Two-Dimensional Molybdenum-Based MXenes, Chem. Mater., 2017, 29, 6472–6479 CrossRef CAS.
- M. Naguib, R. R. Unocic, B. L. Armstrong and J. Nanda, Large-Scale Delamination of Multi-Layers Transition Metal Carbides and Carbonitrides MXenes, Dalton Trans., 2015, 44, 9353–9358 RSC.
- X. H. Wang and Y. C. Zhou, Stability, and Selective Oxidation of Aluminum in Nano Laminate Ti3AlC2 Upon Heating in Argon, Chem. Mater., 2003, 15, 3716–3720 CrossRef CAS.
- J. Yu Si, B. Tawiah, W. L. Sun, B. Lin, C. Wang, A. C. Y. Yuen, B. Y. A. Li, W. Yang and H. D. Lu, et.al., Functionalization of MXene Nanosheets for Polystyrene Towards High Thermal Stability and Flame Retardant Properties, J. Polym., 2019, 11, 976 Search PubMed.
- V. Chaudhary, A. Gautam, Y. K. Mishra and A. Kaushik, Emerging MXene Polymer Hybrid Nanocomposites for High-Performance Ammonia Sensing and Monitoring, J. Nanomater., 2021, 11, 2496 CrossRef CAS PubMed.
- X. Jiang, A. V. Kuklin, A. Baev, Y. Ge, H. Agren, H. Zhang and P. N. Prasad, Two-Dimensional MXenes: From Morphological to Optical, Electric, and Magnetic Properties and Applications, Phys. Rep., 2020, 848, 1–58 CrossRef CAS.
- P. A. Maughan, V. R. Seymour, R. Bernardo, Gavito, D. J. Kelly, S. Shao, S. Tantisriyanurak, R. Dawson, S. J. Haigh, R. J. Young, N. Tapia-Ruiz and N. Bimbo, Porous Silica-Pillared MXenes with Controllable Interlayer Distances for Long-Life Na-Ion Batteries, Langmuir, 2020, 36(16), 4370–4382 CrossRef CAS PubMed.
- Z. U. D. Babar, J. Fatheema, N. Arif, M. S. Anwar, S. Gul, M. Iqbal and S. Rizwan, Magnetic Phase Transition from Paramagnetic in Nb2AlC-MAX to Superconductivity-Like Diamagnetic in Nb2C-MXene: An Experimental and Computational Analysis, RSC Adv., 2020, 10, 25669 RSC.
- H. Kim, B. Anasori, Y. Gogotsi and H. N. Alshareef, Thermoelectric Properties of Two-Dimensional Molybdenum-Based MXenes, Chem. Mater., 2017, 29, 6472–6479 CrossRef CAS.
- C. Chen, et al., MoS2-on-MXene Heterostructures as Highly Reversible Anode Materials for Lithium-Ion Batteries, Angew. Chem., Int. Ed., 2018, 57, 1846–1850 CrossRef CAS PubMed.
- Q. X. Xia, J. Fu, J. M. Yun, R. S. Maneb and K. H. Kim, High Volumetric Energy Density Annealed-MXene Nickel Oxide/MXene Asymmetric Supercapacitor, RSC Adv., 2017, 7, 11000–11011 RSC.
- A. Zaheer, S. A. Zahra, M. Z. Iqbal, A. Mahmood, S. A. Khan and S. Rizwan, Nickel-Adsorbed Two-Dimensional Nb2C MXene for Enhanced Energy Storage Applications, RSC Adv., 2022, 12, 4624 RSC.
- S. A. Zahra, B. Anasori, M. Z. Iqbal, F. Ravaux, A. Tarawneh and M. S. Rizwana, Enhanced Electrochemical Performance of Vanadium Carbide MXene Composites for Supercapacitors, APL Mater., 2022, 10, 060901 CrossRef CAS.
- R. Li, X. Yu, X. Bian and F. Hu, Preparation, and Electrochemical Performance of VO2 Hollow Spheres as a Cathode for Aqueous Zinc Ion Batteries, RSC Adv., 2019, 9, 35117 RSC.
- T. Yaqoob, M. Rani, A. Mahmood, R. Shafique, S. Khan, N. K. Janjua, A. A. Shah, A. Ahmad and A. A. Al-Kahtani, MXene/Ag2CrO4 Nanocomposite as Supercapacitors Electrode, Materials, 2021, 14, 6008 CrossRef CAS PubMed.
- X. Zang, J. Wang, Y. Qin, T. Wang, C. He, Q. Shao, H. Zhu and N. Cao, Enhancing Capacitance Performance of Ti3C2Tx MXene as Electrode Materials of Supercapacitor: From Controlled Preparation to Composite Structure Construction, Nano-Micro Lett., 2020, 12, 77 CrossRef CAS PubMed.
- Y. Dall'Agnese, M. R. Lukatskaya, K. M. Cook, P. L. Taberna, Y. Gogotsi and P. Simon, High Capacitance of Surface Modified 2D Titanium Carbide in Acidic Electrolyte, Electrochem. Commun., 2014, 48, 118–122 CrossRef.
- C. Zhang, et al., Synthesis and Charge Storage Properties of Hierarchical Niobium Pentoxide/Carbon/Niobium Carbide (MXene) Hybrid Materials, Chem. Mater., 2016, 28(11), 3937–3943 CrossRef CAS.
- M. Boota, B. Anasori, C. Voigt, M. Q. Zhao, M. W. Barsoum and Y. Gogotsi, Pseudocapacitive Electrodes Produced by Oxidant-Free Polymerization of Pyrrole Between the Layers of 2D Titanium Carbide (MXene), Adv. Mater., 2016, 28, 1517–1522 CrossRef CAS PubMed.
- X. Wang, Q. Fu, J. Wen, X. Ma, C. Zhu, X. Zhang and D. Qi, 3D Ti3C2Txaerogels with Enhanced Surface Area For High Performance Supercapacitors, Nanoscale, 2018, 10, 20828–20835 RSC.
- M. Fatima, et al., Nb-Doped MXene with Enhanced Energy Storage Capacity and Stability, Front. Chem., 2020, 8, 1–8 CrossRef PubMed.
- H. Liu, et al., Kinetic Study on LiFePO4/C Nanocomposites Synthesized by Solid State Technique, J. Power Sources, 2006, 159, 717–720 CrossRef CAS.
- A. J. Bard and L. R. Faulkner, Electrochemical Methods, John Wiley & Sons, Inc., 2001, 2 edn, p. 211 Search PubMed.
- S. Khamsanga1, R. Pornprasertsuk, T. Yonezawa, A. A. Mohamad and S. Kheawhom, δ-MnO2 nanofower/Graphite Cathode for Rechargeable Aqueous Zinc Ion Batteries, Sci. Rep., 2019, 9, 8441 CrossRef PubMed.
Footnote |
† Electronic supplementary information (ESI) available: Some important formulas for calculation of the electrochemical parameters are provided in the supplementary information. See DOI: https://doi.org/10.1039/d2ra05552j |
|
This journal is © The Royal Society of Chemistry 2022 |
Click here to see how this site uses Cookies. View our privacy policy here.