DOI:
10.1039/D2RA06149J
(Paper)
RSC Adv., 2022,
12, 33304-33312
A series of N-trinitromethyl-substituted polynitro-pyrazoles: high-energy-density materials with positive oxygen balances†
Received
29th September 2022
, Accepted 14th November 2022
First published on 22nd November 2022
Abstract
An N-trinitromethyl strategy was employed for the synthesis of polynitro-pyrazole based high-energy-density compounds with great potential as energetic materials. The new compounds were characterized by 1H and 13C NMR, IR spectroscopy, elemental analysis, differential scanning calorimetry, and single-crystal X-ray diffraction. Compound 10 exhibits high energetic properties, has a positive oxygen balance (OB) of +2.1%, and an excellent specific impulse (272.4 s), making it a potential high-energy dense oxidizer to replace AP in solid rocket propellants. The nitration of 7 with HNO3/H2SO4 yielded the green primary explosive 12, which showed higher density, higher performance, better oxygen balance and lower sensitivities to those of currently used diazodinitrophenol. Compound 13 is a nitrogen and oxygen rich secondary explosive with a high OB (+5.0%), comparable energy (D = 9030 m s−1; P = 35.6 GPa; η = 1.03) to HMX, and much lower mechanical sensitivity (IS = 12 J, FS = 240 N).
Introduction
Energetic materials represent an important class of compounds that can rapidly release a large amount of energy when subjected to appropriate external stimuli to accomplish propulsion, damage and other purposes, and are widely used in civil and military applications.1 Creating new nitrogen-rich, energetic compounds has been a long-standing goal of chemists and has attracted considerable interest due to the technical challenges involved in synthesis and isolation.2 New high-energy-density materials (HEDMs) must meet increasing energy requirements, also considering oxygen balance, thermal stability and sensitivity.
In recent years, five-membered nitrogen-rich heterocyclic compounds (azoles) have emerged as the most promising skeleton for the design and synthesis of advanced HEDMs.3 The azoles have a large number of N–N and C–N bonds and aromatic heterocycles, and their energy is released from the high positive heat of formation rather than by the oxidation of the carbon backbone as in traditional explosives (eg: TNT, TATB).4 Therefore, azoles tend to have high energy density and good thermal stability. Among them, pyrazole is easy to carry out electrophilic substitution reactions such as nitration and halogenation due to the compactness, stability and modifiability of its own structure, and the density and nitrogen content of nitro-substituted pyrazole increase with the increase of the nitro group on the ring.5 It can improve the detonation performance of the target compound. In recent years, many valuable nitropyrazole energetic compounds have been synthesized successively, such as 4-amino-3,5-dinitropyrazole (LLM-116), 3,6-dinitro-1,5-dihydropyrazolo[4,3-c]pyrazole (DNPP), and 3,4,5-trinitropyrazole (TNP).6
However, they have not yet found use as energetic materials due to the presence of strongly acidic N–H protons. Salt formation,7 N-hydroxylation,8 N-dinitromethylation,9 N-trinitromethylation,10 N-nitromethylation,11 N,N′-alkyl bridge,12 N-methylation,13 N-amination14 are among the various approaches that have been used to address this problem (Fig. 1). However, except for hydroxylation and di/tri-nitromethylation, the oxygen balances of nitropyrazoles cannot be improved, and the energy performances of the modified compounds are often reduced. Unfortunately, N-hydroxy derivatives were found to be generally very sensitive to impact, for example 3,4,5-trinitropyrazole-1-ol15 was tested to be IS = 1 J. Therefore, in this work, we report the N-trinitromethylation of four nitropyrazoles, and the obtained six di/trinitromethyl-substituted energetic compounds. Most of these compounds were found to have detonation performances superior to that of RDX, positive oxygen balances and specific impulses higher than that of HMX.
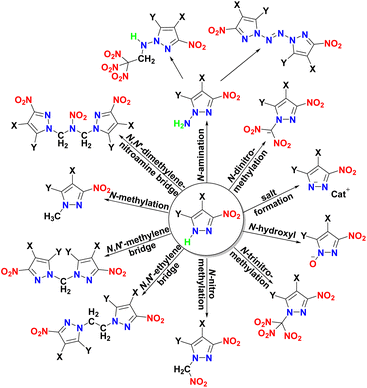 |
| Fig. 1 Various approaches to remove the acidic N–H proton in nitropyrazole (X, Y: –NO2, –NH2, –N3, –H, etc.). | |
Results and discussion
Synthesis
The synthetic routes are shown in Scheme 1. Compounds 1–4 were synthesized according to the literature.5b,6c,12b By the reaction of 1, 2, and 4 with bromoacetone under alkaline conditions, N-alkyl-functionalized nitropyrazoles 6, 5, and 9 were easily obtained in the yields of 86.5, 81.3, and 75.2%, respectively. While compounds 7 and 8 were obtained by reaction of 3 and 4 with KBr and chloroacetone in DMF at 70 °C. Compounds 5–8 were nitrated with a mixture of 98% H2SO4 and 98% HNO3 for 2–5 h at 60 °C to give the corresponding trinitromethyl compounds 10–12 and 14. Interestingly, when 7 was nitrated by the same mixed acid system at room temperature for 6 h, 13 was obtained in 44.9% yield. While 9 was treated similarly, N-dinitromethyl substituted 15 was obtained.
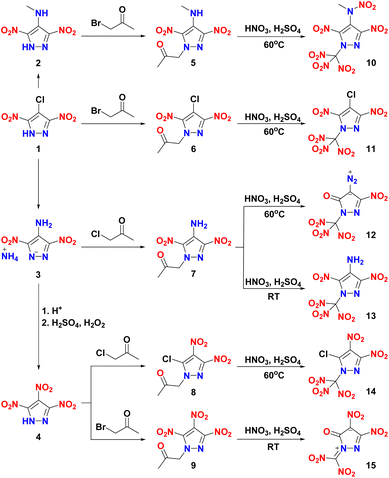 |
| Scheme 1 Synthetic routes for compounds 10–15. | |
Single crystal X-ray diffraction
Crystals of 2, 6, 7, and 10–15 suitable for single-crystal X-ray diffraction were obtained via the slow evaporation of their methanol or diethyl ether solution at room temperature, and their structures are shown in Fig. 2, and Fig. S1–S6.† Their crystallographic data, bond lengths, bond angles, and hydrogen bonds are given in the ESI.†
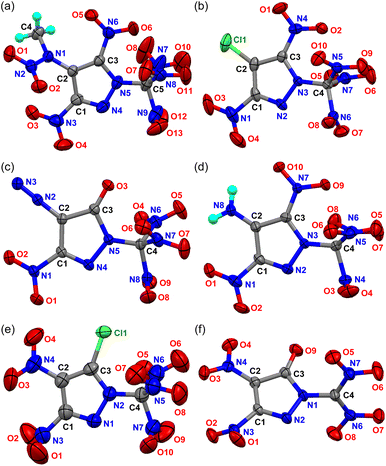 |
| Fig. 2 Crystal structures of 10–15 (a–f). Thermal ellipsoids are drawn at the 50% probability level. | |
Compound 2 crystallizes in a monoclinic crystal system (space group Pc). Compound 6 and 7 all crystallizes in the monoclinic P21c space group. Their C–C, C–N, and N–N bond lengths are normal in pyrazoles. Many hydrogen bonds can be clearly observed from their packing diagrams (Fig. S2, S4 and S6†).
Compound 10 crystallizes in the orthorhombic space group Pbca with a density of 1.894 g cm−3 at 150 K and eight molecules per unit cell. Its density is much higher than that of N-(3,5-dinitro-1H-pyrazol-4-yl)-N-methylnitramide5b (1.74 g cm−3). Only the nitro group attached to C3 is substantially coplanar with the pyrazole ring, which could be seen from the torsional angle of O6–N6–C3–N5 (2.59°) and O5–N6–C3–C2 (3.43°). The adjacent trinitromethyl group is staggered so that its two nearer nitro groups straddle the ring plane fairly symmetrically (see Fig. 2a). The nitrogen atom N9 of the other nitro group is almost in line with C1 and N4 on the pyrazole ring (N9–N4–C1: 172.39°), and the distance from N4 is 2.561 Å. Even in this optimal conformation, several nonbonding distances are significantly less than their corresponding van der Waals (e.g., O6⋯N7 = 2.702 Å, O6⋯N8 = 2.757 Å, van der Waals O⋯N = 2.90 Å), which is a good indicator of repulsive strain. A comparison of bond angles provides further evidence of strain as bond angles near the close contact region are several degrees larger than the corresponding types of bond angles further away; e.g., the closer trinitromethyl N–C–N angle is 112.32° (N7–C5–N8), while the farther ones are 105.85° (N7–C5–N9) and 104.65° (N8–C5–N9). Combining with the literature,16 it is shown that the similar arrangement is formed when the trinitromethyl group is in the ortho position of the nitro group.
Compounds 11–13 all crystallize in the monoclinic space group P21/n. Their calculated densities are 1.960 g cm−3 (173 K), 1.927 g cm−3 (170 K), and 1.895 g cm−3 (193 K), higher than that of 10 (1.894 g cm−3 at 150 K). All substituents except trinitromethyl group in 11–13 are nearly coplanar with the pyrazole ring. The structural features of the trinitromethyl group in the three compounds are similar to those of 10. Compared with 4-diazo-5-nitro-pyrazol-3-one,17 the C
O bond in N-trinitromethyl-substituted compound 12 is shorter, but both the C–NO2 and C–N2+ bonds are longer.
Compound 14 crystallizes in the monoclinic space group P21/c with four molecules per unit cell and a calculated density of 1.859 g cm−3 at 296 K. Compound 15 crystallizes with two water molecules in the triclinic P
space group with two molecules per unit cell. The C1 and C2 positions of the pyrazole ring in compounds 14 and 15 are adjacent nitro groups, and the C3 position is an electronegative single atom (chlorine and oxygen). Due to steric hindrance, the nitro group at C2 twists slightly out of the plane of the pyrazole ring, while the nitro group at C1 has a larger twist angle.
A series of short intermolecular O⋯O and O⋯N contacts could be observed in the crystals, indicating the presence of weak interactions between the single molecules in the solid state. However, only a few hydrogen bonding interactions were found in 10, 13 and 15·2H2O (Fig. 3).
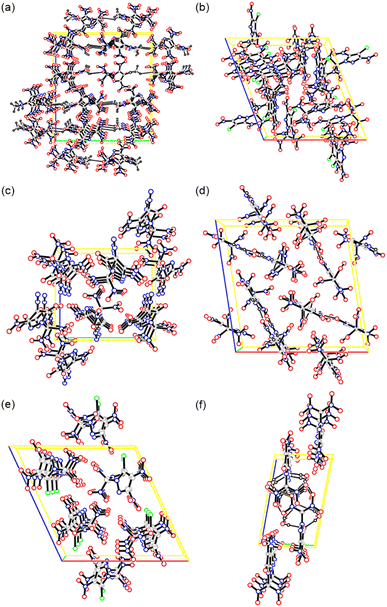 |
| Fig. 3 Packing diagrams of 10–15 (a–f). Unit cell indicated and dashed lines represent hydrogen bonding. | |
To gain more insight into the intermolecular interactions, two-dimensional (2D) fingerprints of crystals and the associated Hirshfeld surfaces (Fig. 4) were analyzed using Crystalexplorer 17.5 (ref. 18). The red and blue areas on the Hirshfeld surfaces represent high and low close contact populations, respectively. Fig. 4 shows red dots scattered in many orientations, mainly near the oxygen atoms of the trinitromethyl, nitro, and carbonyl groups, thus indicating strong intermolecular O⋯O, N⋯O, O⋯N, and O⋯C interactions. In addition to these interactions, compounds 11 and 14 have apparent Cl⋯O interactions between the chlorine and the oxygen atoms of the nitro groups in the adjacent molecule. Compounds 10, 13 and 15 also have H⋯O interactions, that is, hydrogen bonds. Furthermore, in the 2D fingerprint plots of crystal 13 (Fig. 4k), a pair of spikes on bottom left (H⋯O & O⋯H interactions) indicate intermolecular hydrogen bonds. While, there is only one spike in 15, because its molecule contains no hydrogen and only acts as hydrogen bond acceptors. According to the di + de values of the spikes, it can be seen that the O⋯H hydrogen bonds in 15 are stronger than the N⋯H hydrogen bonds in 13. In fact, there are also weak O⋯H interactions in 10, and their proportion (28.2%) is even higher than those (22.8%) in 13. Except for compound 15, the proportions of O⋯O interactions are more than 50%, indicating that compounds 10–14 are high dense. Since the oxygen atoms are in the trinitromethyl and nitro groups, higher proportion of close O⋯O contacts indicates that more nitro groups exposed on the molecular surface, may leading to an increased possibility of unexpected explosion.
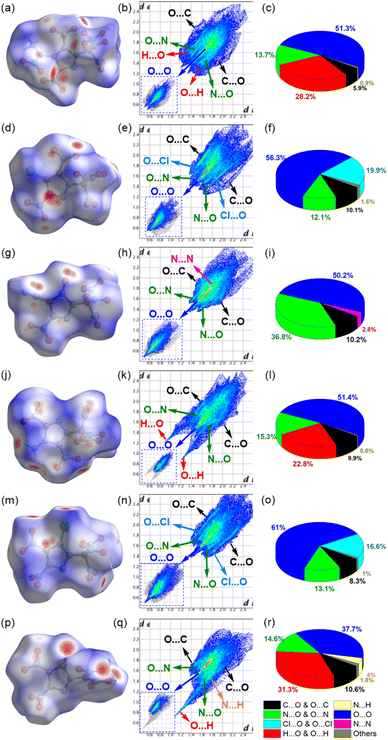 |
| Fig. 4 (a–c) Hirshfeld surface, 2D fingerprint plot, and pie graph of the individual atomic contact percentage for 10. (d–f) Hirshfeld surface, 2D fingerprint plot, and pie graph of the individual atomic contact percentage for 11. (g–i) Hirshfeld surface, 2D fingerprint plot, and pie graph of the individual atomic contact percentage for 12. (j–l) Hirshfeld surface, 2D fingerprint plot, and pie graph of the individual atomic contact percentage for 13. (m–o) Hirshfeld surface, 2D fingerprint plot, and pie graph of the individual atomic contact percentage for 14. (p–r) Hirshfeld surface, 2D fingerprint plot, and pie graph of the individual atomic contact percentage for 15. | |
Physicochemical and energetic properties
To identify the decomposition temperatures of high energy density compounds 10–15, differential scanning calorimetry (DSC) with a heating rate of 5 °C min−1 was used. The results are shown in Fig. 5 and Table 1.
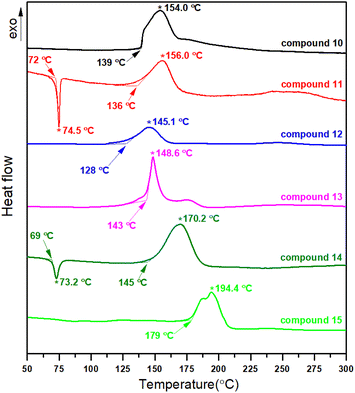 |
| Fig. 5 DSC curves of compounds 10–15 measured with a heating rate of 5 °C min−1. | |
Table 1 Physicochemical properties of 10, 11, 12, 13, 14 and 15
Compound |
Formula |
Tda [°C] |
ρb [g cm−3] |
ΔHfc [kJ mol−1] |
Dd [m s−1] |
Pe [GPa] |
ISf [J] |
FSg [N] |
OB(CO2)h [%] |
Ni [%] |
N + Oj [%] |
Ispk [%] |
ηl |
Decomposition temperature (onset temperature from DSC at a heating rate of 5 °C min−1). Density, measured at 25 °C by a gas pycnometer. Heat of formation. Detonation velocity calculated by EXPLO5 V6.05.04. Detonation pressure calculated by EXPLO5 V6.05.04. Impact sensitivity. Friction sensitivity. Oxygen balance for CaHbOcNd, 1600(c-2a-b/2)/Mw; Mw = molecular weight. Nitrogen content. Nitrogen and oxygen content. Specific impulse calculated by EXPLO5 V6.05.04. Calculated metal acceleration ability by Muravyev–Wozniak–Piercey method. |
10 |
C5H3N9O12 |
139 |
1.853 |
516.67 |
9082 |
36.3 |
5 |
100 |
+2.1 |
33.08 |
83.45 |
272.4 |
1.04 |
11 |
C4ClN7O10 |
136 |
1.924 |
457.47 |
8438 |
31.5 |
10 |
220 |
+11.7 |
28.71 |
75.55 |
251.4 |
0.98 |
12 |
C4N8O9 |
128 |
1.891 |
479.75 |
9023 |
35.2 |
3 |
50 |
+5.3 |
36.85 |
84.20 |
263.7 |
1.04 |
13 |
C4H2N8O10 |
143 |
1.866 |
389.92 |
9030 |
35.6 |
12 |
240 |
+5.0 |
34.79 |
84.46 |
266.5 |
1.03 |
14 |
C4ClN7O10 |
145 |
1.859 |
456.38 |
8184 |
29.0 |
15 |
200 |
+11.7 |
28.71 |
75.55 |
251.4 |
0.95 |
15 |
C4N6O9 |
179 |
1.861 |
386.55 |
8821 |
33.7 |
20 |
180 |
+5.8 |
30.44 |
82.60 |
264.6 |
1.04 |
RDX20 |
C3H6N6O6 |
210 |
1.806 |
86.3 |
8801 |
33.6 |
7.5 |
120 |
−21.61 |
37.84 |
81.06 |
258 |
0.96 |
HMX20 |
C4H8N8O8 |
279 |
1.904 |
116.1 |
9193 |
37.8 |
7 |
112 |
−21.61 |
37.84 |
81.06 |
258 |
1.00 |
Among the six title compounds, only compounds 11 and 14 containing chlorine substituents have obvious endothermic peaks before decomposition, and the corresponding melting points were 72 °C (11) and 69 °C (14), respectively. All other compounds are directly decomposed without going through the melting process (Fig. 5). The decomposition temperatures of 10–14 range from 128 to 145 °C, which are consistent with the decomposition temperatures of most trinitromethyl functionalized compounds.19 Compound 14, as an isomer of 11, has a decomposition temperature nearly 10 °C higher than that of 11. N-dinitromethyl substituted nitropyrazole 15 are more thermally stable than all N-trinitromethyl substituted nitropyrazoles.
The mechanical sensitivities of compounds 10–15 toward impact (IS) and friction (FS) were determined by using a BAM drop hammer apparatus and BAM friction tester, respectively. The results are summarized in Table 1. Compound 12 (IS = 3 J, FS = 50 N) is the most sensitive among them, due to the structure of its diazonium inner salt. N-trinitromethyl makes its impact sensitivity higher than that of the unsubstituted 4-diazo-5-nitro-pyrazol-3-one (IS = 4.5 J),17 but the friction sensitivity is slightly reduced. Six nitro groups are in one molecule of 10, resulting in its impact and friction sensitivities of 5 J and 100 N. Other compounds have sensitivities ranging from 10–20 J and 180–240 N, which are more insensitive than RDX (7.5 J, 120 N) and HMX (7 J, 112 N).20 It is worth mentioning that 13 has a “NO2–NH2–NO2” structure similar to TATB and LLM-105, which may be an important reason for its lower sensitivity than 1-trinitromethyl-3,4,5-trinitropyrazole (7 J, 120 N).10a
The electrostatic potential (ESP) on the molecular surface here is used to analyze the relationship between molecular structure and impact sensitivity. The ESP-mapped vdW surfaces of 10–15 are shown in Fig. 6, with electron-rich areas marked blue and electron-deficient marked red. Surface local maxima and minima of ESP are represented as orange and cyan spheres, respectively. We can see that the positive electrostatic potential is mainly distributed near the pyrazole ring and the methyl, chlorine, amino and diazo groups. The negative electrostatic potential is mainly distributed around the oxygen atoms of the nitro or carbonyl groups. According to the results of the Multiwfn21 analysis, the average values of the positive electrostatic potential of the six compounds are greater than those of the negative electrostatic potential. The charge distribution of the molecular surface of impact-sensitive compounds corresponds to electron-poor areas above C–NO2 bonds. Molecules that are more sensitive to impact have a larger electron deficiency (larger maxima of ESP) in this region than molecules that are less sensitive.22 Compounds 12 and 10 show extremely intense electropositive areas, and the contrast is considerably stronger than others, which corresponds to the higher impact sensitivities and fits with the results of studies by Murray and Politzer.23 It is worth mentioning that, the area of the positive potential region (128.7 Å2) in 13 is slightly smaller than the negative potential region (129.4 Å2), which is contrary to the general phenomenon in high energy density compounds. This may be an important reason for its relative insensitivity. For the dinitromethyl substituted 15, the electron density appears to be more evenly distributed over the molecular surface than in the case for the trinitromethyl substituted 10–14. This would account for a lower impact sensitivity of 15 (20 J) compared to 11–14 (3–15 J).
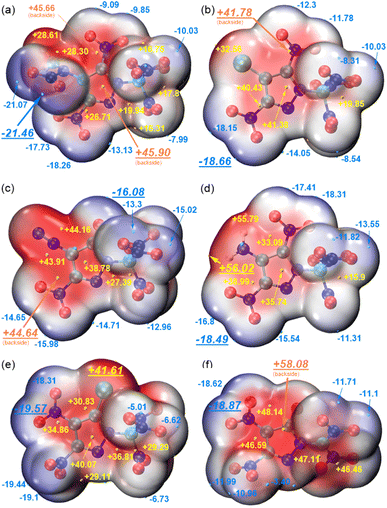 |
| Fig. 6 The ESP-mapped vdW surfaces of 10–15 (a–f). ESP colour (blue-white-red) range from −0.04 to +0.04 Hartrees (a.u.). | |
Their densities were determined at room temperature by a gas pycnometer to be 1.853 (10) to 1.924 g cm−3 (11), which are higher than that of RDX (1.80 g cm−3). Heats of formation were calculated using the Gaussian 09 (Revision A.02) suite of programs24 with isodesmic reactions (ESI†). Compounds 10–15 have relatively high positive heats of formation (ΔHf) falling between 386.55 (15) and 516.67 (10) kJ mol−1, which exceed both RDX (86.3 kJ mol−1) and HMX (116.1 kJ mol−1). Using the calculated ΔHf values and the measured densities, the detonation parameters of 10–15 were calculated using the EXPLO5 V6.05.04 program.25 Their detonation pressures (P) were predicted to be 29.0–36.3 GPa and the detonation velocities (D) is 8184–9082 m s−1. Compounds 10, 12, 13, and 15 exhibited higher detonation properties compared to RDX (P = 33.6 GPa, D = 8801 m s−1).20 In addition, the results calculated according to the Muravyev–Wozniak–Piercey method26 show that compounds 10, 12, 13, and 15 have slightly higher metal acceleration abilities (1.03–1.04) than HMX (1.00).
Energetic materials with a zero or positive oxygen balance (OB) convert all C into CO2 and all H into H2O. It is desirable that the energy can be completely released upon explosion while producing a minimal amount of toxic gas, so they can be considered “greener” than those with negative OBs.27 The OB values of 10–15 are all positive (+2.1% to +11.7%), indicating that they are potential high-energy oxidizers for solid propellants. Therefore, specific impulse (Isp), the most significant measure of the efficiency of a propellant (in seconds), was also calculated by using EXPLO5 V6.05.04.25 Compounds 10, 12, 13, and 15 have specific impulses of 263.7–272.4 s, which are much higher than those of AP (157 s),19 ADN (202 s)19 and HMX (258 s).20
Introduction of a N-trinitromethyl moiety is an effective way to enhance the OB of an energetic material. This is seen with the N-trinitromethyl substituted nitropyrazoles 10 (+2.1%), 12 (+5.3%), and 13 (+5.0%) having higher OB than their analogues N-(3,5-dinitro-1H-pyrazol-4-yl)-N-methylnitramide (−27.6%),5b 4-diazo-5-nitro-pyrazol-3-one (−36.1%),17 and LLM-116 (−32.4%).6b In addition to the OB, their densities and heats of formation also increased to varying degrees (only the density of 13 decreased by 0.03 g cm−3). In turn, their detonation velocities, detonation pressures, and metal acceleration abilities have all increased (Fig. 7). Taken together, these comparisons highlight the N-trinitromethyl strategy as an efficient functional modification to enhance OB and energy properties.
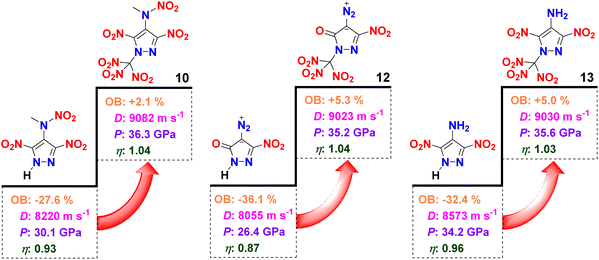 |
| Fig. 7 Comparison of trinitromethyl substituted nitropyrazoles. | |
Conclusions
In conclusion, a series of N-trinitromethyl-substituted polynitro-pyrazoles were obtained by a simple and efficient synthesis, including N-acetomethylation followed by N-trinitromethylation. The novel compounds and their precursors were characterized by 1H and 13C NMR spectroscopy, elemental analysis, IR spectroscopy, and differential scanning calorimetry. The structures of 2, 6, 7, and 10–15 were confirmed by single-crystal X-ray diffraction. Compound 10 exhibits excellent energetic performance (ρ = 1.853 g cm−3; ΔHf = 516.67 kJ mol−1; D = 9082 m s−1; P = 36.3 GPa), has a positive OB of +2.1%, and a promising specific impulse (272.4 s), making it an applicable high-energy dense oxidizer to replace AP in solid rocket propellants. The nitration of 7 with HNO3/H2SO4 mixed acid generated in situ yielded the diazonium inner salt of polynitropyrazole 12, the higher density, higher performance, better oxygen balance and lower sensitivity of which relative to diazodinitrophenol (DDNP) make it a competitive candidate as a green primary explosive. Compound 13 is a secondary explosive with a high OB (+5.0%), comparable energy (D = 9030 m s−1; P = 35.6 GPa; η = 1.03) to HMX, and much lower sensitivity to external stimuli (IS = 12 J, FS = 240 N).
Experimental
Caution! All the nitro compounds are energetic materials. And they tend to explode unpredictably, under certain conditions. Caution should be exercised at all times during the handling of any of these compounds. Leather coat, ear protection, latex gloves, and face shield are strongly recommended for the experimental operation.
4-Chloro-3,5-dinitro-1H-pyrazole (1),12b N-methyl-3,5-dinitro-1H-pyrazol-4-amine (2),5b ammonium 4-amino-3,5-dinitropyrazole-1-ide (3),12b 3,4,5-trinitro-1H-pyrazole (4),6c 1-(4-amino-3,5-dinitro-1H-pyrazol-1-yl)propan-2-one (7),9 and 1-(3,4,5-trinitro-1H-pyrazol-1-yl)propan-2-one (9)28 were synthesized according to the literature.
1-(4-(Methylamino)-3,5-dinitro-1H-pyrazol-1-yl)propan-2-one (5)
A solution of bromoacetone (2.88 g, 21 mmol, 1.765 mL) in acetone (3 mL) was added drop-wise to a solution of compound 2 (3.74 g, 20 mmol) and NaOH (0.8 g, 20 mmol) in water (10 mL). The resulting mixture was stirred at room temperature for 18 h and a precipitate was isolated, which was washed with ice-water (20 mL), dried in air, giving 5 as a brown solid (3.95 g, 81.3%). Tm: 143 °C. 1H NMR: δ 7.41, 7.40, 5.61, 2.98, 2.97, 2.26 ppm; 13C NMR: δ 200.36, 141.71, 132.58, 130.69, 33.17, 26.93 ppm. IR (ATR):
3352, 2940, 2360, 1729, 1608, 1518, 1486, 1435, 1415, 1382, 1366, 1331, 1306, 1208, 1178, 1119, 1035, 898, 830, 815, 790, 745, 658, 611, 581 cm−1. Elemental analysis for C7H9N5O5 (243.179): calcd C 34.57, H 3.73, N 28.80%. Found: C 34.49, H 3.66, N 28.72%.
1-(4-Chloro-3,5-dinitro-1H-pyrazol-1-yl)propan-2-one (6)
a solution of bromoacetone (2.88 g, 21 mmol, 1.765 mL) in acetone (3 mL) was added drop-wise to a solution of compound 1 (3.85 g, 20 mmol) and NaOH (0.8 g, 20 mmol) in water (10 mL). The resulting mixture was stirred at room temperature for 18 h and a precipitate was isolated, which was washed with ice-water (20 mL), dried in air, giving 6 as a white solid (4.30 g, 86.5%). Tm: 117 °C. 1H NMR: δ 5.82, 2.32 ppm; 13C NMR: δ 199.63, 148.66, 142.53, 106.13, 64.15, 26.96 ppm. IR (ATR):
3585, 3015, 2938, 2359, 1737, 1557, 1538, 1501, 1448, 1408, 1361, 1317, 1205, 1174, 1144, 1071, 1045, 993, 895, 826, 813, 789, 744, 685, 647, 631, 549 cm−1. Elemental analysis for C6H5ClN4O5 (248.579): calcd C 28.99, H 2.03, N 22.54%. Found: C 28.92, H 2.11, N 22.48%.
1-(5-Chloro-3,4-dinitro-1H-pyrazol-1-yl)propan-2-one (8)
Compound 4 (0.609 g, 3 mmol) was dissolved in DMF (3 mL), followed by the addition of sodium bicarbonate (0.252 g, 3 mmol) and potassium bromide (0.357 g, 3 mmol) in stirring. The reaction system was heated to 70 °C, then chloroacetone (0.333 g, 3.6 mmol, 0.287 mL) was added drop-wise, and the reaction was maintained at 70 °C for 2 h. After cooling to room temperature, the reaction was quenched in cold water and a precipitate was isolated, which was washed with ice-water (20 mL), dried in air, giving 8 as a white solid (0.223 g, 29.9%). Tm: 131 °C. 1H NMR: δ 5.61, 2.30 ppm; 13C NMR: δ 199.12, 147.12, 131.34, 123.29, 60.12, 27.00 ppm. IR (ATR):
2999, 2954, 2184, 2173, 1993, 1965, 1731, 1538, 1506, 1428, 1400, 1361, 1336, 1310, 1200, 1174, 1071, 1061, 986, 900, 849, 833, 814, 795, 754, 698, 662, 648, 621, 587, 544 cm−1. Elemental analysis for C6H5ClN4O5 (248.579): calcd C 28.99, H 2.03, N 22.54%. Found: C 29.06, H 2.09, N 22.49%.
N-(3,5-Dinitro-1-(trinitromethyl)-1H-pyrazol-4-yl)-N-methylnitramide (10)
Compound 5 (0.365 g, 1.5 mmol) was added to an ice-cold 98% H2SO4 (2.4 mL) and the mixture was stirred at 0–5 °C for 30 min. Then 98% HNO3 (2.6 mL) was added drop-wise into the mixture at 0 °C. After slowly warming to room temperature, the mixture was heated to 60 °C. The final reaction was stirred at 60 °C for 2 h. After pouring into ice-water with vigorous stirring, the final mixture was filtered, washed with ice-water and dried in air, giving 10 as a white solid (0.40 g, 70.0%). Td: 139 °C. 1H NMR: δ 3.81 ppm; 13C NMR: δ 206.56, 152.40, 142.14, 119.66, 41.22 ppm. IR (ATR):
3566, 2934, 2360, 1646, 1633, 1610, 1559, 1478, 1454, 1434, 1397, 1365, 1329, 1289, 1261, 1242, 1150, 1087, 1062, 991, 945, 862, 837, 822, 794, 766, 752, 667, 592, 562 cm−1. Elemental analysis for C5H3N9O12 (381.130): calcd C 15.76, H 0.79, N 33.08%. Found: C 15.82, H 0.81, N 33.11%.
4-Chloro-3,5-dinitro-1-(trinitromethyl)-1H-pyrazole (11)
Compound 6 (1.39 g, 5.6 mmol) was added to an ice-cold 98% H2SO4 (6.0 mL) and the mixture was stirred at 0–5 °C for 30 min. Then 98% HNO3 (6.5 mL) was added drop-wise into the mixture at 0 °C. After slowly warming to room temperature, the mixture was heated to 60 °C. The final reaction was stirred at 60 °C for 5 h. After pouring into ice-water with vigorous stirring, the final mixture was filtered, washed with ice-water and dried in air, giving 11 as a white solid (1.25 g, 65.4%). Tm: 72 °C; Td: 136 °C. 13C NMR: δ 206.91, 154.58, 144.41, 115.41 ppm. IR (ATR):
3586, 2935, 2360, 1737, 1646, 1627, 1606, 1575, 1533, 1428, 1390, 1314, 1257, 1167, 1090, 988, 857, 830, 816, 792, 762, 745, 724, 629, 576 cm−1. Elemental analysis for C4ClN7O10 (341.533): calcd C 14.07, N 28.71%. Found: C 14.16, N 28.78%.
4-Diazo-5-nitro-2-(trinitromethyl)-2,4-dihydro-3H-pyrazol-3-one (12)
Compound 7 (0.343 g, 1.5 mmol) was added to an ice-cold 98% H2SO4 (2.4 mL) and the mixture was stirred at 0–5 °C for 30 min. Then 98% HNO3 (2.6 mL) was added drop-wise into the mixture at 0 °C. After slowly warming to room temperature, the mixture was heated to 60 °C. The final reaction was stirred at 60 °C for 3 h. After pouring into ice-water with vigorous stirring, the final mixture was filtered, washed with ice-water and dried in air, giving 12 as a white solid (0.316 g, 69.3%). Td: 128 °C. 13C NMR: δ 158.75, 155.20, 150.62, 123.47 ppm. IR (ATR):
3501, 2934, 2359, 2197, 1751, 1634, 1596, 1542, 1482, 1446, 1389, 1357, 1319, 1262, 1193, 1156, 1061, 1023, 995, 860, 837, 822, 792, 763, 725, 696, 674, 638, 606, 567 cm−1. Elemental analysis for C4N8O9 (304.091): calcd C 15.80, N 36.85%. Found: C 15.73, N 36.82%.
3,5-Dinitro-1-(trinitromethyl)-1H-pyrazol-4-amine (13)
Compound 7 (0.343 g, 1.5 mmol) was added to an ice-cold 98% H2SO4 (2.4 mL) and the mixture was stirred at 0–5 °C for 30 min. Then 98% HNO3 (2.6 mL) was added drop-wise into the mixture at 0 °C. After slowly warming to room temperature within 2 h, the final reaction was stirred at room temperature for 6 h. After pouring into ice-water with vigorous stirring, the insoluble impurities were filtered off, and the filtrate was left to stand for 12 h, and a large amount of precipitates were formed. Washed with ice-water and dried in air, giving 13 as a pale solid (0.217 g, 44.9%). Td: 143 °C. 1H NMR: δ 8.07 ppm; 13C NMR: δ 206.41, 148.76, 133.18, 130.74 ppm. IR (ATR):
3675, 3484, 3361, 2987, 2901, 2199, 1756, 1659, 1633, 1618, 1599, 1578, 1539, 1469, 1388, 1310, 1265, 1224, 1066, 1052, 975, 863, 838, 820, 794, 758, 738, 691, 655, 639, 597 cm−1. Elemental analysis for C4H2N8O10 (322.106): calcd C 14.92, H 0.63, N 34.79%. Found: C 14.99, H 0.66, N 34.85%.
5-Chloro-3,4-dinitro-1-(trinitromethyl)-1H-pyrazole (14)
Compound 8 (1.39 g, 5.6 mmol) was added to an ice-cold 98% H2SO4 (6.0 mL) and the mixture was stirred at 0–5 °C for 30 min. Then 98% HNO3 (6.5 mL) was added drop-wise into the mixture at 0 °C. After slowly warming to room temperature, the mixture was heated to 60 °C. The final reaction was stirred at 60 °C for 3 h. After pouring into ice-water with vigorous stirring, the final mixture was filtered, washed with ice-water and dried in air, giving 14 as a white solid (1.07 g, 56.1%). Tm: 69 °C; Td: 145 °C. 13C NMR: δ 206.25, 151.88, 136.72, 124.90 ppm. IR (ATR):
2887, 1966, 1621, 1606, 1563, 1537, 1487, 1456, 1361, 1328, 1265, 1140, 1084, 981, 965, 863, 834, 812, 791, 764, 701, 662, 634, 623, 599, 571, 565, 551 cm−1. Elemental analysis for C4ClN7O10 (341.533): calcd C 14.07, N 28.71%. Found: C 14.12, N 28.76%.
1-(Dinitromethylene)-3,4-dinitro-5-oxo-4,5-dihydro-1H-pyrazol-1-ium-4-ide (15)
Compound 9 (0.389 g, 1.5 mmol) was added to an ice-cold 98% H2SO4 (2.4 mL) and the mixture was stirred at 0–5 °C for 30 min. Then 98% HNO3 (2.6 mL) was added drop-wise into the mixture at 0 °C. After slowly warming to room temperature within 2 h, the final reaction was stirred at room temperature for 6 h. After pouring into ice-water with vigorous stirring, the solution was left to stand for 12 h, a small amount of precipitates were formed. Washed with ice-water and dried in air, giving 15 as a white solid (0.154 g, 37.2%). Td: 179 °C. 13C NMR: δ 200.33, 140.25, 131.54, 130.15 ppm. IR (ATR):
1635, 1511, 1372, 1318, 1279, 1122, 1044, 824, 756, 559 cm−1. Elemental analysis for C4N6O9 (276.077): calcd C 17.40, N 30.44%. Found: C 17.33, N 30.51%.
Conflicts of interest
There are no conflicts to declare.
Acknowledgements
Financial support of this work is provided by the National Natural Science Foundation of China (No. 22105102, and No. 21975127), the Young Elite Scientist Sponsorship Program by CAST (no. YESS20210074), and the Fundamental Research Funds for the Central Universities (No. 30921011204).
Notes and references
-
(a) M. B. Talawar, R. Sivabalan, T. Mukundan, H. Muthurajan, A. K. Sikder, B. R. Gandhe and A. Subhananda Rao, J. Hazard. Mater., 2009, 161, 589–607 CrossRef CAS PubMed;
(b) D. G. Piercey, D. E. Chavez, B. L. Scott, G. H. Imler and D. A. Parrish, Angew. Chem., Int. Ed., 2016, 55, 15315–15318 CrossRef CAS PubMed.
-
(a) T. M. Klapötke, C. Petermayer, D. G. Piercey and J. Stierstorfer, J. Am. Chem. Soc., 2012, 134, 20827–20836 CrossRef;
(b) P. Yin, Q. Zhang and J. M. Shreeve, Acc. Chem. Res., 2016, 49, 4–16 CrossRef CAS;
(c) W. Zhang, J. Zhang, M. Deng, X. Qi, F. Nie and Q. Zhang, Nat. Commun., 2017, 8, 181 CrossRef PubMed;
(d) P. Yin, J. Zhang, G. H. Imler, D. A. Parrish and J. M. Shreeve, Angew. Chem., Int. Ed., 2017, 56, 8834–8838 CrossRef CAS.
-
(a) H. Gao and J. M. Shreeve, Chem. Rev., 2011, 111, 7377–7436 CrossRef CAS;
(b) A. A. Dippold and T. M. Klapötke, J. Am. Chem. Soc., 2013, 135, 9931–9938 CrossRef CAS.
-
(a) Y. Qu and S. P. Babailov, J. Mater. Chem. A, 2018, 6, 1915–1940 RSC;
(b) Y. Zhang, Y. Li, J. Hu, Z. Ge, C. Sun and S. Pang, Dalton Trans., 2019, 48, 1524–1529 RSC.
-
(a) S. Zhang, Z. Gao, D. Lan, Q. Jia, N. Liu, J. Zhang and K. Kou, Molecules, 2020, 25, 3475 CrossRef CAS PubMed;
(b) C. He, J. Zhang, D. A. Parrish and J. M. Shreeve, J. Mater. Chem. A, 2013, 1, 2863–2868 RSC;
(c) M. Deng, Y. Feng, W. Zhang, X. Qi and Q. Zhang, Nat. Commun., 2019, 10, 1339 CrossRef PubMed;
(d) H. Huang, Y. Shi, H. Li, H. Li, A. Pang and J. Yang, Org. Lett., 2020, 22, 5866–5869 CrossRef CAS PubMed.
-
(a) S. Ek and N. V. Latypov, J. Heterocycl. Chem., 2014, 51, 1621–1627 CrossRef CAS;
(b) M. Zhang, P. F. Pagoria, G. H. Imler and D. Parrish, J. Heterocycl. Chem., 2019, 56, 781–787 CrossRef CAS;
(c) G. Hervé, C. Roussel and H. Graindorge, Angew. Chem., Int. Ed., 2010, 49, 3177–3181 CrossRef;
(d) Y. Li, Y. Shu, B. Wang, S. Zhang and L. Zhai, RSC Adv., 2016, 6, 84760–84768 RSC;
(e) W. Zhang, H. Xia, R. Yu, J. Zhang, K. Wang and Q. Zhang, Propellants, Explos., Pyrotech., 2020, 45, 546–553 CrossRef CAS;
(f) P. Yin, J. Zhang, L. A. Mitchell, D. A. Parrish and J. M. Shreeve, Angew. Chem., Int. Ed., 2016, 55, 12895–12897 CrossRef CAS.
-
(a) Y. Feng, J. Wang and Z. Li, Cryst. Growth Des., 2021, 21, 4725–4731 CrossRef CAS;
(b) Y. Zhang, Y. Huang, D. A. Parrish and J. M. Shreeve, J. Mater. Chem., 2011, 21, 6891–6897 RSC;
(c) M. F. Bölter, A. Harter, T. M. Klapötke and J. Stierstorfer, ChemPlusChem, 2018, 83, 804–811 CrossRef.
-
(a) P. Yin, L. A. Mitchell, D. A. Parrish and J. M. Shreeve, Chem.–Asian J., 2017, 12, 378–384 CrossRef CAS;
(b) P. Yin, L. A. Mitchell, D. A. Parrish and J. M. Shreeve, Angew. Chem., Int. Ed., 2016, 55, 14409–14411 CrossRef CAS PubMed.
- B. Guo, X. Zhang, X. Lin, H. Huang and J. Yang, New J. Chem., 2021, 45, 20426–20431 RSC.
-
(a) W. Zhang, Y. Yang, Y. Wang, T. Fei, Y. Wang, C. Sun and S. Pang, Chem. Eng. J., 2023, 451, 138609 CrossRef CAS;
(b) I. L. Dalinger, I. A. Vatsadze, T. K. Shkineva, A. V. Kormanov, M. I. Struchkova, K. Yu. Suponitsky, A. A. Bragin, K. A. Monogarov, V. P. Sinditskii and A. B. Sheremetev, Chem.–Asian J., 2015, 10, 1987–1996 CrossRef CAS PubMed.
- D. Kumar, G. H. Imler, D. A. Parrish and J. M. Shreeve, J. Mater. Chem. A, 2017, 5, 10437–10441 RSC.
-
(a) J. Zhang, C. He, D. A. Parrish and J. M. Shreeve, Chem.–Eur. J., 2013, 19, 8929–8936 CrossRef CAS;
(b) D. Fischer, J. L. Gottfried, T. M. Klapötke, K. Karaghiosoff, J. Stierstorfer and T. G. Witkowski, Angew. Chem., Int. Ed., 2016, 55, 16132–16135 CrossRef CAS;
(c) P. Yin, J. Zhang, D. A. Parrish and J. M. Shreeve, Chem.–Eur. J., 2014, 20, 16529–16536 CrossRef CAS PubMed.
-
(a) I. L. Dalinger, I. A. Vatsadze, T. K. Shkineva, G. P. Popova, S. A. Shevelev and Y. V. Nelyubina, J. Heterocycl. Chem., 2013, 50, 911–924 CrossRef CAS;
(b) P. Yin, C. He and J. M. Shreeve, J. Mater. Chem. A, 2016, 4, 1514–1519 RSC.
-
(a) P. Yin, J. Zhang, C. He, D. A. Parrish and J. M. Shreeve, J. Mater. Chem. A, 2014, 2, 3200–3208 RSC;
(b) G. Li, H. Huang, J. Yang, C. Yan, W. Li and H. Duan, Polyhedron, 2021, 199, 115098 CrossRef CAS;
(c) P. Yin, D. A. Parrish and J. M. Shreeve, Chem.–Eur. J., 2014, 20, 6707–6712 CrossRef CAS.
- Y. Zhang, D. A. Parrish and J. M. Shreeve, J. Mater. Chem., 2012, 22, 12659–12665 RSC.
- Y. Xu, C. Shen, Q. Lin, P. Wang, C. Jiang and M. Lu, J. Mater. Chem. A, 2016, 4, 17791–17800 RSC.
- Y. Du, J. Zhang, P. Peng, H. Su, S. Li and S. Pang, New J. Chem., 2017, 41, 9244–9249 RSC.
- M. A. Spackman and D. Jayatilaka, CrystEngComm, 2009, 11, 19–32 RSC.
- Q. Yu, F. Li, P. Yin, S. Pang, R. J. Staples and J. M. Shreeve, J. Mater. Chem. A, 2021, 9, 24903–24908 RSC.
- N. Fischer, D. Fischer, T. M. Klapötke, D. G. Piercey and J. Stierstorfer, J. Mater. Chem., 2012, 22, 20418–20422 RSC.
- T. Lu and F. Chen, J. Comput. Chem., 2012, 33, 580–592 CrossRef CAS.
- A. Hammerl, T. M. Klapötke, H. Nöth and M. Warchhold, Propellants, Explos., Pyrotech., 2003, 28, 165–173 CrossRef CAS.
-
(a) J. S. Murray, M. C. Concha and P. Politzer, Mol. Phys., 2009, 107, 89–97 CrossRef CAS;
(b) P. Politzer, J. S. Murray, J. M. Seminario, P. Lane, M. E. Grice and M. C. Concha, THEOCHEM, 2001, 573, 1–10 CrossRef CAS.
- M. J. Frisch, G. W. Trucks, H. B. Schlegel, G. E. Scuseria, M. A. Robb, J. R. Cheeseman, G. Scalmani, V. Barone, B. Mennucci, G. A. Petersson, H. Nakatsuji, M. Caricato, X. Li, H. P. Hratchian, A. F. Izmaylov, J. Bloino, G. Zheng, J. L. Sonnenberg, M. Hada, M. Ehara, K. Toyota, R. Fukuda, J. Hasegawa, M. Ishida, T. Nakajima, Y. Honda, O. Kitao, H. Nakai, T. Vreven, J. A. Montgomery Jr., J. E. Peralta, F. Ogliaro, M. Bearpark, J. J. Heyd, E. Brothers, K. N. Kudin, V. N. Staroverov, R. Kobayashi, J. Normand, K. Raghavachari, A. Rendell, J. C. Burant, S. S. Iyengar, J. Tomasi, M. Cossi, N. Rega, J. M. Millam, M. Klene, J. E. Knox, J. B. Cross, V. Bakken, C. Adamo, J. Jaramillo, R. Gomperts, R. E. Stratmann, O. Yazyev, A. J. Austin, R. Cammi, C. Pomelli, J. W. Ochterski, R. L. Martin, K. Morokuma, V. G. Zakrzewski, G. A. Voth, P. Salvador, J. J. Dannenberg, S. Dapprich, A. D. Daniels, O. Farkas, J. B. Foresman, J. V. Ortiz, J. Cioslowski and D. J. Fox, Gaussian 09, Revision A.02, Gaussian, Inc., Wallingford, CT, 2009 Search PubMed.
- M. Sućeska, EXPLO5 V6.05.04, Brodarski Institute, Zagreb, Croatia, 2020 Search PubMed.
- N. V. Muravyev, D. R. Wozniak and D. G. Piercey, J. Mater. Chem. A, 2022, 10, 11054–11073 RSC.
- C. He and J. M. Shreeve, Angew. Chem., Int. Ed., 2016, 55, 772–775 CrossRef CAS.
- S. Zhang, F. Wang, X. Li, K. Yu, X. Qiu, C. Zhu and Z. Zhou, Chinese invention Pat., CN114149372A, 2021 Search PubMed.
|
This journal is © The Royal Society of Chemistry 2022 |
Click here to see how this site uses Cookies. View our privacy policy here.