DOI:
10.1039/D1SC06002C
(Edge Article)
Chem. Sci., 2022,
13, 1382-1389
Surface environment complication makes Ag29 nanoclusters more robust and leads to their unique packing in the supracrystal lattice†
Received
1st November 2021
, Accepted 31st December 2021
First published on 3rd January 2022
Abstract
Silver nanoclusters have received unprecedented attention in cluster science owing to their promising functionalities and intriguing physical/chemical properties. However, essential instability significantly impedes their extensive applications. We herein propose a strategy termed “surface environment complication” to endow Ag29 nanoclusters with high robustness. The Ag29(S-Adm)18(PPh3)4 nanocluster with monodentate PPh3 ligands was extremely unstable and uncrystallizable. By substituting PPh3 with bidentate PPh2py with dual coordination sites (i.e., P and N), the Ag29 cluster framework was twisted because of the generation of N–Ag interactions, and three NO3 ligands were further anchored onto the nanocluster surface, yielding a new Ag29(S-Adm)15(NO3)3(PPh2py)4 nanocluster with high stability. The metal-control or ligand-control effects on stabilizing the Ag29 nanocluster were further evaluated. Besides, Ag29(S-Adm)15(NO3)3(PPh2py)4 followed a unique packing mode in the supracrystal lattice with several intercluster channels, which has yet been observed in other M29 cluster crystals. Overall, this work presents a new approach (i.e., surface environment complication) for tailoring the surface environment and improving the stability of metal nanoclusters.
1 Introduction
Since the advent of metal nanoclusters with atomic precision, these novel nanomaterials have garnered significant interest because of their accurate compositions/constructions and intriguing physicochemical properties.1–8 Indeed, owing to their quantum size effect and discrete electronic energy levels, metal nanoclusters and cluster-based nanomaterials display atomic structure tunable properties, that is, slight tailoring of structures of nanoclusters can trigger remarkable differences in their performances.9–13 Besides, metal nanoclusters have been used as ideal platforms for the meticulous investigation of structure–property correlations.14–20 Consequently, metal nanoclusters are an emerging class of programmable nanomaterials for several promising applications, such as catalysis, drug delivery, energy storage, and biological applications.21–24
In the past two decades, silver nanoclusters have received unprecedented attention in cluster science.25–40 It is widely accepted that silver nanoclusters exhibit promising functionalities and intriguing physical/chemical properties that are obviously different from their gold counterparts.27 Ag-based metal nanoclusters generally display strong photoluminescence that renders them optically active nanomaterials for sensors or biological applications.41–43 However, Ag nanoclusters are essentially unstable relative to Au nanoclusters, which significantly impedes their extensive applications. Developing new approaches to enhance the nanocluster stability remains highly desired.
Recently, our group has developed a M29(S-Adm)18(PPh3)4 (where S-Adm is 1-adamantanethiol) nanocluster system for mapping the structure–property correlations at the atomic level.44–46 Although several M29 nanoclusters, e.g., Pt1Ag28(S-Adm)18(PPh3)4 (Pt1Ag28-PPh3 for short), Au1Ag28(S-Adm)18(PPh3)4, and Pt1Ag12Cu16(S-Adm)18(PPh3)4, have been controllably synthesized and structurally determined, the homo-metal Ag29(S-Adm)18(PPh3)4 (Ag29-PPh3 for short) nanocluster was extremely unstable and uncrystallizable.46 We remain committed to stabilizing the homo-silver Ag29 nanocluster with a new approach.
Herein, a “surface environment complication” strategy has been exploited to endow the Ag29 nanocluster with high robustness. By substituting the monodentate PPh3 (with only the P coordination site) in previously reported Ag29-PPh3 with bidentate PPh2py (with P and N dual coordination sites), the nanocluster surface structure underwent a twist due to the generation of N–Ag interactions. Besides, three NO3 ligands were further anchored onto the nanocluster surface, making the metallic kernel entirely wrapped. The obtained Ag29(S-Adm)15(NO3)3(PPh2py)4 (Ag29-PPh2py for short) nanocluster was much more robust relative to Ag29-PPh3, and its structure was successfully determined by single-crystal X-ray diffraction. Furthermore, based on this nanocluster template, the metal-control and ligand-control effects on stabilizing the Ag29 framework were evaluated. Moreover, at the supramolecular level, Ag29-PPh2py followed a unique packing mode in the crystal lattice with several intercluster channels, while such an aggregation pattern has yet been discovered in other M29 cluster crystals.
2 Experimental methods
Materials
All the following reagents were purchased from Sigma-Aldrich and used without further purification: silver nitrate (AgNO3, 99.5%, metal basis), hexachloroplatinic(IV) acid (H2PtCl6·6H2O, 99.9% metals basis), 1-adamantanethiol (Adm-SH, C10H15SH, 99%), triphenylphosphine (PPh3, 99%), diphenyl-2-pyridylphosphine (PPh2py, 97%), sodium borohydride (NaBH4, 99%), methylene chloride (CH2Cl2, HPLC grade), methanol (CH3OH, HPLC grade), ethanol (CH3CH2OH, HPLC grade), and n-hexane (C6H12, HPLC grade).
Synthesis of Ag29(S-Adm)18(PPh3)4 (Ag29-PPh3)
The preparation of Ag29-PPh3 was based on a reported method.46
Synthesis of Pt1Ag28(S-Adm)18(PPh3)4 (Pt1Ag28-PPh3)
The preparation of Pt1Ag28-PPh3 was based on a reported method.46
Preparation of Ag29(S-Adm)15(NO3)3(PPh2py)4 (Ag29-PPh2py)
In a 50 mL round-bottom flask, 94 mg of AgNO3 was dissolved in 5 mL of MeOH and 10 mL of EtOH, and 50 mg of Adm-SH was added under vigorous stirring. After 20 min, 100 mg of PPh2py was added. Shortly after this, 10 mg of NaBH4 (dissolved in 1 mL of EtOH) was poured in, and the reaction was continued for 12 hours. The obtained solution was centrifuged at 10
000 rpm for 5 minutes, and then the supernatant was collected and evaporated to get the dry product, which was then washed several times with n-hexane to get the final product, i.e., Ag29-PPh2py. The yield was about 30% based on the Ag element (calculated from AgNO3).
Preparation of Pt1Ag28(S-Adm)18(PPh2py)4 (Pt1Ag28-PPh2py)
94 mg of AgNO3 used to synthesize Ag29-PPh2py was substituted by 94 mg of AgNO3 and 10 mg of H2PtCl6·6H2O. Other conditions remained unchanged. The yield for the synthesis of Pt1Ag28-PPh2py was about 45% based on the Ag element (calculated from AgNO3).
Crystallization of the Ag29 nanocluster series
Single crystals of Ag29-PPh2py or Pt1Ag28-PPh2py were cultivated at −4 °C by liquid-diffusing n-hexane into the CH2Cl2 solution of each nanocluster. After a week, red crystals were collected, and the structures of these nanoclusters were determined. Of note, in order to accelerate the crystallization process and improve the crystal quality, the counterions (i.e., Cl−) in these nanoclusters were replaced by SbF6− or BPh4−.47 The reaction equation was [Ag29(S-Adm)15(NO3)3(PPh2py)4]Cl3 + 3SbF6− → [Ag29(S-Adm)15(NO3)3(PPh2py)4](SbF6)3 + 3Cl− or [Pt1Ag28(S-Adm)18(PPh2py)4]Cl2 + 2BPh4− → [Pt1Ag28(S-Adm)18(PPh2py)4](BPh4)2 + 2Cl−.
Characterization
The optical absorption spectra of nanoclusters were recorded using an Agilent 8453 diode array spectrometer.
Electrospray ionization mass spectrometry (ESI-MS) measurements were performed by using a Waters XEVO G2-XS QTof mass spectrometer. The sample was directly infused into the chamber at 5 μL min−1. For preparing the ESI samples, nanoclusters were dissolved in CH2Cl2 (1 mg mL−1) and diluted (v/v = 1
:
1) with CH3OH.
Infrared (IR) measurements were recorded on a Bruker Vertex 80sv Fourier transform IR spectrometer.
X-ray crystallography
The data collection for single-crystal X-ray diffraction (SC-XRD) of Ag29-PPh2py was carried out on a Bruker Smart APEX II CCD diffractometer under a nitrogen flow, using graphite-monochromatized Mo Kα radiation (λ = 0.71073 Å). The data collection for single-crystal X-ray diffraction (SC-XRD) of Pt1Ag28-PPh2py was carried out on a Stoe Stadivari diffractometer under a nitrogen flow, using graphite-monochromatized Cu Kα radiation (λ = 1.54186 Å). Data reductions and absorption corrections were performed using the SAINT and SADABS programs, respectively. The structure was solved by direct methods and refined with full-matrix least squares on F2 using the SHELXTL software package. All non-hydrogen atoms were refined anisotropically, and all the hydrogen atoms were set in geometrically calculated positions and refined isotropically using a riding model. All crystal structures were treated with PLATON SQUEEZE. The diffuse electron densities from these residual solvent molecules were removed. The CCDC number of the Ag29-PPh2py nanocluster is 2115749. The CCDC number of the Pt1Ag28-PPh2py nanocluster is 2117814.
3 Results and discussion
Ag29-PPh3 was prepared by a literature method.46 Although the Ag29-PPh3 nanocluster was uncrystallizable because of its weak stability, several of its alloyed derivatives have been structurally determined, including Pt1Ag28-PPh3, Au1Ag28(S-Adm)18(PPh3)4, and Pt1Ag12Cu16(S-Adm)18(PPh3)4.44–46 In this context, alloying has been used as an efficient approach to improve the stability of the M29 framework.46Fig. 1 depicts the proposed structure of Ag29-PPh3. Of note, the Ag13 kernel in Ag29-PPh3 might follow a FCC (face-centered cubic) configuration for two reasons: (i) the consistent FCC configuration of the M13 kernel in PPh3 and S-Adm co-stabilized M29 nanoclusters,44–46 and (ii) the different absorption profiles of Ag29-PPh3 and Ag29-PPh2py (discussed below). However, such a verification calls for more experimental efforts.
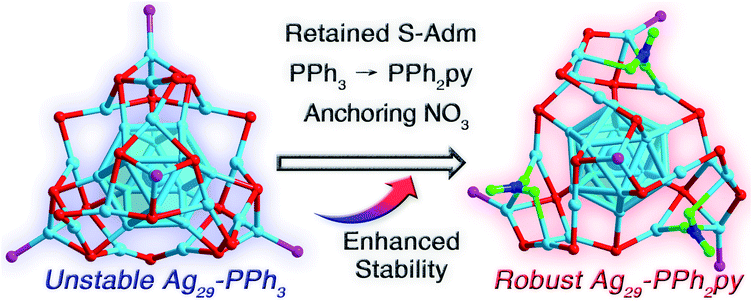 |
| Fig. 1 Structural comparison between unstable Ag29-PPh3 and robust Ag29-PPh2py. Compared with Ag29-PPh3, the Ag29-PPh2py nanocluster contained retained S-Adm ligands, while the surface PPh3 ligands were altered to PPh2py, and several NO3 ligands were arranged on the nanocluster surface. Color legends: light blue sphere, Ag; red sphere, S; magenta sphere, P; blue sphere, N; green sphere, O. For clarity, all C and H atoms are omitted. Of note, the structure of Ag29-PPh3 is proposed in this figure. | |
At the same time, we unremittingly made efforts to stabilize the homo-silver Ag29 and determine its atomically precise structure. Considering that (i) the unchanging S-Adm ligand could retain the basic framework of the Ag29 nanocluster47,48 and (ii) the introduction of N-coordination sites in original ligands would generate new N–metal interactions that might enhance the structural robustness,49–52 we were motivated to substitute the PPh3 ligand with PPh2py while retaining the S-Adm ligand in the nanocluster synthesis. A new Ag29 nanocluster, formulated as Ag29(S-Adm)15(NO3)3(PPh2py)4 (Ag29-PPh2py), was synthesized and further structurally determined owing to its high stability (Fig. 1 and S1†).
Compared with Ag29-PPh3, Ag29-PPh2py contained three fewer S-Adm ligands and three more NO3 ligands, and the number of the phosphine ligands retained was four (Fig. 1). Because of the interactions between N (in PPh2py) and Ag (in the cluster), the surface structure of Ag29-PPh2py displayed more obvious distortion relative to Ag29-PPh3 (Fig. 1 and S2†). Besides, three NO3 ligands were observed on the nanocluster surface via Ag–O interactions. For the three O atoms in each NO3, the two inward O linked to two Ag atoms or one Ag atom, while the outward O was naked (Fig. 1 and S2†). The presence of NO3 in the cluster system has been verified by IR measurement (Fig. S3†). ESI-MS measurement was performed to validate the molecular composition and determine the valence state of the nanocluster. As shown in Fig. S4,† the experimental mass signals at 2292.30 and 2271.64 Da matched well with the theoretical results of [Ag29(S-Adm)15(NO3)3(PPh2py)4]3+ and [Ag29(S-Adm)15(NO3)2(PPh2py)4]3+, respectively. In this context, the NO3 ligand on the nanocluster surface was more prone to be dissociated relative to S-Adm and PPh2py ligands. Besides, the “+3” valence state of Ag29-PPh2py was tallied with the presence of 3SbF6− counterions with an Ag29 cluster molecule in the crystal lattice (Fig. S1†). According to the valence state of the Ag29-PPh2py nanocluster, its nominal electron count was determined to be 8,53i.e., 29(Ag) − 15(SR) − 3(NO3) − 3(charge) = 8e, the same as that of Ag29-PPh3.
Structurally, the Ag29-PPh2py nanocluster contains an icosahedral Ag13 kernel (Fig. 2A). Of note, for other structurally determined M29(S-Adm)18(PR3)4 nanoclusters, their Ag13 kernels follow a FCC configuration.46 The difference between these two kernel configurations originates from their distinguishable surface environments via a “surface-kernel structure transfer effect”. The Ag13 kernel of Ag29-PPh2py is first wrapped by three same Ag4(S-Adm)2(PPh2py)1 motif structures that are further fixed by three S-Adm bridges (Fig. 2B and C), giving rise to an Ag25(S-Adm)9(PPh2py)3 structure (Fig. 2D). Such three Ag4(S-Adm)2(PPh2py)1 motifs or three S-Adm bridges are in C3 axial symmetry. Besides, an Ag4(S-Adm)6(PPh2py)1 surface unit caps the Ag25(S-Adm)9(PPh2py)3 structure to present an Ag29(S-Adm)15(PPh3py)4 structure (Fig. 2E and F). In this context, the four PPh2py ligands follow different bonding modes in the nanocluster framework: three PPh2py are dually bonded onto the nanocluster via both Ag–P and Ag–N interactions, while the remaining one is singly bonded onto the nanocluster vertex via the Ag–P interaction (Fig. S2†). Of note, the Ag29(S-Adm)15(PPh3py)4 structure is still bare to a certain extent, and three NO3 ligands, which originated from the AgNO3 reactant, are further anchored onto the nanocluster surface (Fig. 2G), making the Ag29 kernel fully protected and yielding the overall structure of Ag29-PPh2py (Fig. 2H). The complete structure of Ag29-PPh2py follows a C3 axial symmetry, and the axis of the symmetry passes through the vertex P and the innermost Ag atoms (Fig. S5†).
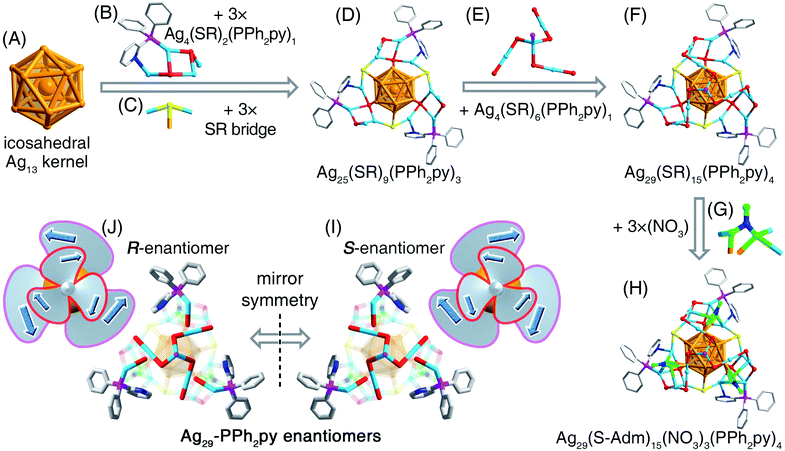 |
| Fig. 2 Structural anatomy of the Ag29-PPh2py nanocluster. (A) The icosahedral Ag13 kernel. (B) and (C) The Ag4(S-Adm)2(PPh2py)1 surface and S-Adm bridge-like units. (D) The Ag25(S-Adm)9(PPh2py)3 structure. (E) The Ag4(S-Adm)6(PPh2py)1 surface unit. (F) Ag29(S-Adm)15(PPh3py)4 structure. (G) The surface NO3 ligand. (H) Overall structure of the Ag29-PPh2py nanocluster. (J) and (I) The Ag29-PPh2py nanocluster enantiomers. Color legends: orange sphere, kernel Ag; light blue sphere, surface Ag; red/yellow sphere, S; magenta sphere, P; blue sphere, N; green sphere, O. For clarity, all H atoms and several C atoms are omitted. | |
In the crystal lattice of Ag29-PPh2py, two nanocluster enantiomers were observed, labeled as the R-nanocluster enantiomer and S-nanocluster enantiomer in Fig. 2I and J. Each type of enantiomer displayed a bilayer rotation: (i) for the S-nanocluster enantiomer, the inner-layer (i.e., the Ag4(S-Adm)6(PPh2py)1) was counterclockwise while the outer-layer (i.e., assembly of three surface Ag1(S-Adm)1(PPh2py)1) was clockwise (Fig. 2I); (ii) for the R-nanocluster enantiomer, the rotations of the inner-layer and outer-layer were opposite to those of the S-nanocluster enantiomer (Fig. 2J). Since the quantities of R- and S-nanocluster enantiomers are the same in the crystal lattice, the nanocluster samples were racemic.
The Ag29-PPh3 and Ag29-PPh2py nanoclusters with distinguishable kernel structures and surface environments exhibited different optical absorptions. The CH2Cl2 solution of Ag29-PPh3 showed an intense absorption at 413 nm and a shoulder band at 506 nm (Fig. S6,† black line). By comparison, the CH2Cl2 solution of Ag29-PPh3 showed several apparent UV-vis signals at 401, 438, and 530 nm (Fig. S6,† red line). The difference in optical absorptions of these two Ag29 nanoclusters suggested their distinct electronic structures.54,55 The photoluminescence properties of Ag29-PPh3 and Ag29-PPh2py nanoclusters were further compared. As shown in Fig. S7,† the CH2Cl2 solution of Ag29-PPh3 was red emissive with an intense signal at 622 nm. By comparison, the Ag29-PPh2py was non-emissive in the solution state. The different photophysical properties originated from their distinct electronic structures.54,55
The thermal stability of these two Ag29 nanoclusters was then compared in air. As shown in Fig. 3A, the characteristic optical peaks of Ag29-PPh3 continuously decreased in the first three hours and completely disappeared within six hours, demonstrating the decomposition of the nanoclusters. In this context, the Ag29-PPh3 nanocluster was unstable. In vivid contrast, the optical absorptions of Ag29-PPh2py remained unchanged for 24 hours (Fig. 3B), which suggested the high robustness of this nanocluster. Besides, the difference in stability was primarily responsible for the crystallographic discrepancy of these two Ag29 nanoclusters: the Ag29-PPh3 nanocluster was uncrystallizable, whereas the crystal structure of Ag29-PPh2py was successfully determined.
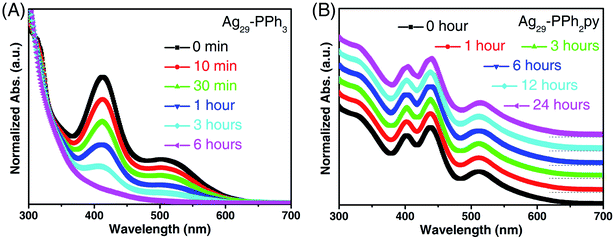 |
| Fig. 3 Stability of different Ag29 nanoclusters. (A) Time-dependent optical absorptions of Ag29-PPh3 in CH2Cl2 in air. (B) Time-dependent optical absorptions of Ag29-PPh2py in CH2Cl2 in air. | |
Collectively, as depicted in Fig. 4A, two approaches have been presented to endow the unstable Ag29-PPh3 nanocluster with enhanced stability: (i) the metal control approach (e.g., from unstable Ag29-PPh3 to stable Pt1Ag28-PPh3),46 and (ii) the ligand control approach (i.e., from unstable Ag29-PPh3 to stable Ag29-PPh2py). These two disparately stabilizing approaches raised an interesting question: which type of the Pt1Ag28 nanocluster would be generated when the metal control and the ligand control were performed simultaneously in the synthesis (Fig. 4B)?
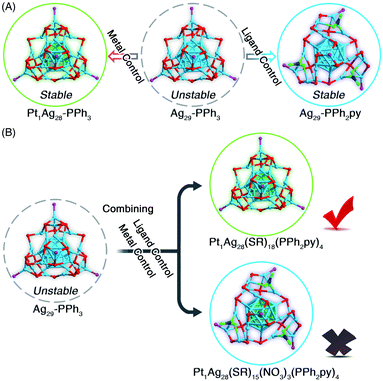 |
| Fig. 4 Metal control versus ligand control on the Ag29 nanocluster template. (A) From unstable Ag29-PPh3 to stable Pt1Ag28-PPh3via metal control, or from unstable Ag29-PPh3 to stable Ag29-PPh2pyvia ligand control. (B) From unstable Ag29-PPh3 to stable Pt1Ag28-PPh2pyvia both metal control and ligand control. Color legends: light blue sphere, Ag; dark green sphere, Pt; red sphere, S; magenta sphere, P; blue sphere, N; green sphere, O. For clarity, all C and H atoms are omitted. | |
As inspired by the aforementioned results, two types of Pt1Ag28 nanoclusters with different surface environments might be generated (Fig. 4B): Pt1Ag28(S-Adm)18(PPh2py)4 with a maintained framework or Pt1Ag28(S-Adm)15(NO3)3(PPh2py)4 with a twisted framework. After the crystallographic analysis, we determined its structure as the framework-retained Pt1Ag28(S-Adm)18(PPh2py)4 (Pt1Ag28-PPh2py for short). The structure of Pt1Ag28-PPh2py was almost the same as that of Pt1Ag28-PPh3 (Fig. S8†).44,46 Although the four PPh2py ligands in Pt1Ag28-PPh2py exposed N coordination sites, these N sites remained uncoordinated in the nanocluster formation (Fig. S8†). Consequently, in the competition between metal control and ligand control in this nanocluster system, the metal control seized a dominant position (Fig. 4B). In other words, when the Pt heteroatom was introduced into the innermost region of the nanocluster, the M29 structure was robust enough to hinder the formation of surface Ag–N interactions, which resulted in a retained cluster framework without any distortion. Besides, in the previously reported intercluster transformation from Pt1Ag28-PPh3 into Pt1Ag28(BDT)12(PPh3)4 (BDT = 1,3-benzenedithiolate), the presence of BDT afforded the kernel transformation from FCC into icosahedron.56 In this context, for the Pt1Ag28 cluster template, the bidentate thiolate ligand (i.e., BDT) showed enhanced ability for directing the nanocluster configuration relative to the bidentate phosphine ligand (i.e., PPh2py).
The Ag29-PPh2py nanocluster molecules followed a crystallographic pattern of “lamellar eutectic” between R-nanocluster and S-nanocluster enantiomers, viewed from both x and y axes (Fig. S9A–C†). The interlayer distance along the z axis was determined to be 34.064 Å (from cluster kernel to cluster kernel, as shown in Fig. S9B†). Significantly, the supracrystal lattice of Ag29-PPh2py showed several intercluster channels with the same diameter of 18.875 Å from the (001) crystalline plane (Fig. 5A and S9D†), which was reminiscent of the behavior of MOFs (metal–organic frameworks).57,58 However, the channel diameter should be remarkably less than 18.875 Å due to the presence of carbon tails from peripheral ligands of nanoclusters (Fig. S10†). The intercluster channel was constructed by symmetrically assembling six cluster molecules into a hexagon, where three molecules were R-nanocluster enantiomers (marked in orange in Fig. 5B), while the other three were S-nanocluster enantiomers (marked in blue in Fig. 5B). Specifically, the intercluster hexagon was composed of two cluster-based triangles in parallel planes in opposite directions, and each triangle contained three cluster molecules in the same enantiomeric configuration (Fig. 5B and C). The intermolecular distance of the cluster-based triangle was 22.224 Å, and the interlayer distance between two adjacent triangles was 18.816 Å (Fig. 5B and C). Furthermore, the arrangement of SbF6− counterions in the supracrystal lattice was analyzed. As shown in Fig. S11,† 2/3 of SbF6− counterions were uniformly organized in the intercluster channels while the others were packed along the C3 axis of symmetry of Ag29-PPh2py nanoclusters. Of note, such a hexagon-like crystallographic packing of Ag29-PPh2py cluster molecules in the supracrystal lattice was unique, which has yet been detected in other M29 nanocluster crystals.44–46,48,59,60 For example, for the crystal lattice of Pt1Ag28-PPh2py, the nanocluster molecules were packed in a layered assembly mode from the x axis, y axis, or z axis, and no intercluster channel was detected (Fig. S12†). In this context, such unique intercluster channels may render the Pt1Ag28-PPh2py crystals potential nanomaterials for gas adsorption-related applications.61–65
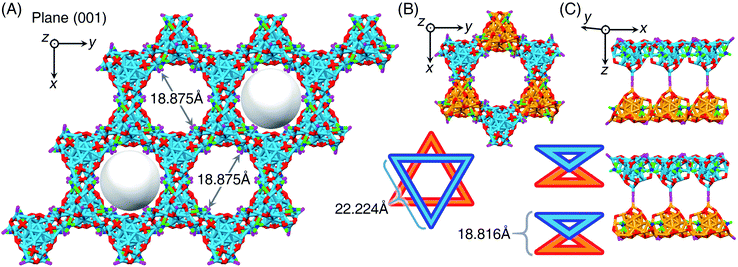 |
| Fig. 5 Packing of Ag29-PPh2py in the supracrystal lattice. (A) Crystalline packing of Ag29-PPh2py, viewed from the (001) plane. (B) Vertical and (C) lateral views of the aggregation pattern of Ag29-PPh2py molecules in the supracrystal lattice. Color legends: light blue sphere, Ag in the S-nanocluster enantiomer; orange sphere, Ag in the R-nanocluster enantiomer; red sphere, S; magenta sphere, P; blue sphere, N; green sphere, O. For clarity, all C and H atoms are omitted. | |
4 Conclusions
In summary, a strategy termed “surface environment complication” has been exploited to render unstable Ag29 highly robust. The surface structure of unstable Ag29(S-Adm)18(PPh3)4 underwent directional distortion due to the generation of Ag–N interactions by substituting the monodentate PPh3 ligand with bidentate PPh2py. Besides, three NO3 ligands were anchored onto the nanocluster surface to entirely protect the Ag29 kernel, yielding a new Ag29(S-Adm)15(NO3)3(PPh2py)4 nanocluster with high robustness. Owing to its enhanced stability, the Ag29(S-Adm)15(NO3)3(PPh2py)4 nanocluster was crystallizable, and its atomically precise structure was successfully determined. On the supramolecular level, the Ag29(S-Adm)15(NO3)3(PPh2py)4 nanocluster molecules followed a unique crystallographic packing mode and displayed several intercluster channels. This study thus presented a novel strategy for tailoring the surface environment of metal nanoclusters, and also provided fundamental insights into the controllable synthesis of highly robust silver nanoclusters. Future work will focus on promoting this strategy to other ligand-protected metal nanoclusters.
Data availability
All the data supporting this article have been included in the main text and the ESI.†
Author contributions
C. X. and Q. Y. carried out the experiments and analyzed the data. X. W., H. L. and H. S. assisted in the analysis. X. K. and M. Z. designed the project, analyzed the data, and wrote the manuscript.
Conflicts of interest
There are no conflicts to declare.
Acknowledgements
We acknowledge the financial support of the NSFC (21631001, 21871001, and 22101001), the Ministry of Education, and the University Synergy Innovation Program of Anhui Province (GXXT-2020-053).
Notes and references
- R. Jin, C. Zeng, M. Zhou and Y. Chen, Chem. Rev., 2016, 116, 10346–10413 CrossRef CAS PubMed
.
- I. Chakraborty and T. Pradeep, Chem. Rev., 2017, 117, 8208–8271 CrossRef CAS PubMed
.
- X. Kang, Y. Li, M. Zhu and R. Jin, Chem. Soc. Rev., 2020, 49, 6443–6514 RSC
.
- Q. Yao, T. Chen, X. Yuan and J. Xie, Acc. Chem. Res., 2018, 51, 1338–1348 CrossRef CAS PubMed
.
- A. W. Cook and T. W. Hayton, Acc. Chem. Res., 2018, 51, 2456–2464 CrossRef CAS PubMed
.
- N. Xia and Z. Wu, Chem. Sci., 2021, 12, 2368–2380 RSC
.
- S. Takano and T. Tsukuda, J. Am. Chem. Soc., 2021, 143, 1683–1698 CrossRef CAS PubMed
.
- S. Kenzler and A. Schnepf, Chem. Sci., 2021, 12, 3116–3129 RSC
.
- K. Kwak and D. Lee, Acc. Chem. Res., 2019, 52, 12–22 CrossRef CAS PubMed
.
- B. Nieto-Ortega and T. Bürgi, Acc. Chem. Res., 2018, 51, 2811–2819 CrossRef CAS PubMed
.
- M. Agrachev, M. Ruzzi, A. Venzo and F. Maran, Acc. Chem. Res., 2019, 52, 44–52 CrossRef CAS PubMed
.
- T. Higaki, Q. Li, M. Zhou, S. Zhao, Y. Li, S. Li and R. Jin, Acc. Chem. Res., 2018, 51, 2764–2773 CrossRef CAS PubMed
.
- X. Kang and M. Zhu, Chem. Soc. Rev., 2019, 48, 2422–2457 RSC
.
- N. A. Sakthivel and A. Dass, Acc. Chem. Res., 2018, 51, 1774–1783 CrossRef CAS PubMed
.
- Y. Liu, X. Chai, X. Cai, M. Chen, R. Jin, W. Ding and Y. Zhu, Angew. Chem., Int. Ed., 2018, 57, 9775–9779 CrossRef CAS PubMed
.
- S. Ito, S. Takano and T. Tsukuda, J. Phys. Chem. Lett., 2019, 10, 6892–6896 CrossRef CAS PubMed
.
- Y. Wang, E. Makkonen, X. Chen and T. Bürgi, Chem. Sci., 2021, 12, 9413–9419 RSC
.
- W. Fei, S. Antonello, T. Dainese, A. Dolmella, M. Lahtinen, K. Rissanen, A. Venzo and F. Maran, J. Am. Chem. Soc., 2019, 141, 16033–16045 CrossRef CAS PubMed
.
- M. Hesari, H. Ma and Z. Ding, Chem. Sci., 2021, 12, 14540–14545 RSC
.
- H. Yoshida, M. Ehara, U. D. Priyakumar, T. Kawai and T. Nakashima, Chem. Sci., 2020, 11, 2394–2400 RSC
.
- W. Kurashige, Y. Niihori, S. Sharma and Y. Negishi, Coord. Chem. Rev., 2016, 320, 238–250 CrossRef
.
- J. Yan, B. K. Teo and N. Zheng, Acc. Chem. Res., 2018, 51, 3084–3093 CrossRef CAS PubMed
.
- M. H. Naveen, R. Khan and J. H. Bang, Chem. Mater., 2021, 33, 7595–7612 CrossRef CAS
.
- T. Kawawaki, Y. Kataoka, S. Ozaki, M. Kawachi, M. Hirata and Y. Negishi, Chem. Commun., 2021, 57, 417–440 RSC
.
- B. Bhattarai, Y. Zaker, A. Atnagulov, B. Yoon, U. Landman and T. P. Bigioni, Acc. Chem. Res., 2018, 51, 3104–3113 CrossRef CAS PubMed
.
- L. He and T. Dong, CrystEngComm, 2021, 23, 7369–7379 RSC
.
- C. P. Joshi, M. S. Bootharaju, M. J. Alhilaly and O. M. Bakr, J. Am. Chem. Soc., 2015, 137, 11578–11581 CrossRef CAS PubMed
.
- A. Desireddy, B. E. Conn, J. Guo, B. Yoon, R. N. Barnett, B. M. Monahan, K. Kirschbaum, W. P. Griffith, R. L. Whetten, U. Landman and T. P. Bigioni, Nature, 2013, 501, 399–402 CrossRef CAS PubMed
.
- H. Yang, Y. Wang, H. Huang, L. Gell, L. Lehtovaara, S. Malola, H. Häkkinen and N. Zheng, Nat. Commun., 2013, 4, 2422 CrossRef PubMed
.
- L. G. AbdulHalim, M. S. Bootharaju, Q. Tang, S. Del Gobbo, R. G. AbdulHalim, M. Eddaoudi, D.-e. Jiang and O. M. Bakr, J. Am. Chem. Soc., 2015, 137, 11970–11975 CrossRef CAS PubMed
.
- K.-G. Liu, X.-M. Gao, T. Liu, M.-L. Hu and D.-e. Jiang, J. Am. Chem. Soc., 2020, 142, 16905–16909 CrossRef CAS PubMed
.
- M. Qu, H. Li, L.-H. Xie, S.-T. Yan, J.-R. Li, J.-H. Wang, C.-Y. Wei, Y.-W. Wu and X.-M. Zhang, J. Am. Chem. Soc., 2017, 139, 12346–12349 CrossRef CAS PubMed
.
- F. Tian and R. Chen, J. Am. Chem. Soc., 2019, 141, 7107–7114 CrossRef CAS PubMed
.
- A. Jana, M. Jash, A. K. Poonia, G. Paramasivam, M. R. Islam, P. Chakraborty, S. Antharjanam, J. Machacek, S. Ghosh, K. N. V. D. Adarsh, T. Base and T. Pradeep, ACS Nano, 2021, 15, 15781–15793 CrossRef CAS PubMed
.
- X. Liu, J. Chen, J. Yuan, Y. Li, J. Li, S. Zhou, C. Yao, L. Liao, S. Zhuang, Y. Zhao, H. Deng, J. Yang and Z. Wu, Angew. Chem., Int. Ed., 2018, 57, 11273–11277 CrossRef CAS PubMed
.
- L. Qin, F. Sun, X. Ma, G. Ma, Y. Tang, L. Wang, Q. Tang, R. Jin and Z. Tang, Angew. Chem., Int. Ed., 2021, 60, 26136–26141 CrossRef CAS PubMed
.
- X.-Q. Liang, Y.-Z. Li, Z. Wang, S.-S. Zhang, Y.-C. Liu, Z.-Z. Cao, L. Feng, Z.-Y. Gao, Q.-W. Xue, C.-H. Tung and D. Sun, Nat. Commun., 2021, 12, 4966 CrossRef CAS PubMed
.
- S.-F. Yuan, C.-Q. Xu, W.-D. Liu, J.-X. Zhang, J. Li and Q.-M. Wang, J. Am. Chem. Soc., 2021, 143, 12261–12267 CrossRef CAS PubMed
.
- R. S. Dhayal, J.-H. Liao, Y.-C. Liu, M. H. Chiang, S. Kahlal, J.-Y. Saillard and C. W. Liu, Angew. Chem., Int. Ed., 2015, 54, 3702–3706 CrossRef CAS PubMed
.
- H. Yang, Y. Wang, X. Chen, X. Zhao, L. Gu, H. Huang, J. Yan, C. Xu, G. Li, J. Wu, A. J. Edwards, B. Dittrich, Z. Tang, D. Wang, L. Lehtovaara, H. Häkkinen and N. Zheng, Nat. Commun., 2016, 7, 12809 CrossRef CAS PubMed
.
- Y. Tao, M. Li, J. Ren and X. Qu, Chem. Soc. Rev., 2015, 44, 8636–8663 RSC
.
- Y. Nie, X. Tao, Y. Zhou, X. Yuan, Y. Zhuo, Y.-q. Chai and R. Yuan, Anal. Chem., 2021, 93, 1120–1125 CrossRef CAS PubMed
.
- P. Lopez, H. H. Lara, S. M. Mullins, D. M. Black, H. M. Ramsower, M. M. Alvarez, T. L. Williams, X. Lopez-Lozano, H.-C. Weissker, A. P. García, I. L. Garzón, B. Demeler, J. L. Lopez-Ribot, M. J. Yacamán and R. L. Whetten, ACS Appl. Nano Mater., 2018, 1, 1595–1602 CrossRef CAS PubMed
.
- X. Kang, M. Zhou, S. Wang, S. Jin, G. Sun, M. Zhu and R. Jin, Chem. Sci., 2017, 8, 2581–2587 RSC
.
- X. Kang, L. Huang, W. Liu, L. Xiong, Y. Pei, Z. Sun, S. Wang, S. Wei and M. Zhu, Chem. Sci., 2019, 10, 8685–8693 RSC
.
- X. Kang, X. Wei, S. Jin, Q. Yuan, X. Luan, Y. Pei, S. Wang, M. Zhu and R. Jin, Proc. Natl. Acad. Sci. U. S. A., 2019, 116, 18834–18840 CrossRef CAS PubMed
.
- X. Kang, S. Jin, L. Xiong, X. Wei, M. Zhou, C. Qin, Y. Pei, S. Wang and M. Zhu, Chem. Sci., 2020, 11, 1691–1697 RSC
.
- X. Kang, X. Wei, S. Wang and M. Zhu, Inorg. Chem., 2020, 59, 8736–8743 CrossRef CAS PubMed
.
- Y. Jin, C. Zhang, X.-Y. Dong, S.-Q. Zang and T. C. W. Mak, Chem. Soc. Rev., 2021, 50, 2297–2319 RSC
.
- R.-W. Huang, Y.-S. Wei, X.-Y. Dong, X.-H. Wu, C.-X. Du, S.-Q. Zang and T. C. W. Mak, Nat. Chem., 2017, 9, 689–697 CrossRef CAS PubMed
.
- Z. Lei, X.-L. Pei, Z.-G. Jiang and Q.-M. Wang, Angew. Chem., Int. Ed., 2014, 53, 12771–12775 CrossRef CAS PubMed
.
- M. J. Alhilaly, R.-W. Huang, R. Naphade, B. Alamer, M. N. Hedhili, A.-H. Emwas, P. Maity, J. Yin, A. Shkurenko, O. F. Mohammed, M. Eddaoudi and O. M. Bakr, J. Am. Chem. Soc., 2019, 141, 9585–9592 CrossRef CAS PubMed
.
- M. Walter, J. Akola, O. Lopez-Acevedo, P. D. Jadzinsky, G. Calero, C. J. Ackerson, R. L. Whetten, H. Grönbeck and H. Häkkinen, Proc. Natl. Acad. Sci. U. S. A., 2008, 105, 9157–9162 CrossRef CAS PubMed
.
- Q. Tang, G. Hu, V. Fung and D.-e. Jiang, Acc. Chem. Res., 2018, 51, 2793–2802 CrossRef CAS PubMed
.
- C. M. Aikens, Acc. Chem. Res., 2018, 51, 3065–3073 CrossRef CAS PubMed
.
- M. S. Bootharaju, S. M. Kozlov, Z. Cao, A. Shkurenko, A. M. El-Zohry, O. F. Mohammed, M. Eddaoudi, O. M. Bakr, L. Cavallo and J.-M. Basset, Chem. Mater., 2018, 30, 2719–2725 CrossRef CAS
.
- O. M. Yaghi, M. O'Keeffe, N. W. Ockwig, H. K. Chae, M. Eddaoudi and J. Kim, Nature, 2003, 423, 705–714 CrossRef CAS PubMed
.
- Q. Yang, Q. Xu and H.-L. Jiang, Chem. Soc. Rev., 2017, 46, 4774–4808 RSC
.
- X. Kang, X. Wei, S. Jin, S. Wang and M. Zhu, Inorg. Chem., 2021, 60, 4198–4206 CrossRef CAS PubMed
.
- X. Kang, F. Xu, X. Wei, S. Wang and M. Zhu, Sci. Adv., 2019, 5, eaax7863 CrossRef CAS PubMed
.
- R. Custelcean, Chem. Sci., 2021, 12, 12518–12528 RSC
.
- B. Hua, Y. Ding, L. O. Alimi, B. Moosa, G. Zhang, W. S. Baslyman, J. Sessler and N. M. Khashab, Chem. Sci., 2021, 12, 12286–12291 RSC
.
- X. Wei, X. Kang, Q. Yuan, C. Qin, S. Jin, S. Wang and M. Zhu, Chem. Mater., 2019, 31, 4945–4952 CrossRef CAS
.
- X. Wei, X. Kang, Z. Zuo, F. Song, S. Wang and M. Zhu, Nat. Sci. Rev., 2021, 8, nwaa077 CrossRef CAS PubMed
.
- G. Liu, W. S. Y. Wong, M. Kraft, J. W. Ager, D. Vollmer and R. Xu, Chem. Soc. Rev., 2021, 50, 10674–10699 RSC
.
Footnotes |
† Electronic supplementary information (ESI) available: Fig. S1–S12 for the crystal structure, ESI-MS and UV-vis results of nanoclusters. CCDC 2115749 and 2117814. For ESI and crystallographic data in CIF or other electronic format see DOI: 10.1039/d1sc06002c |
‡ C. X. and Q. Y. contributed equally to this work. |
|
This journal is © The Royal Society of Chemistry 2022 |
Click here to see how this site uses Cookies. View our privacy policy here.