DOI:
10.1039/D1SC06135F
(Edge Article)
Chem. Sci., 2022,
13, 2729-2734
Atropoenantioselective palladaelectro-catalyzed anilide C–H olefinations viable with natural sunlight as sustainable power source†
Received
4th November 2021
, Accepted 9th February 2022
First published on 10th February 2022
Abstract
Enantioselective electrocatalyzed transformations represent a major challenge. We herein achieved atropoenantioselective pallada-electrocatalyzed C–H olefinations and C–H allylations with high efficacy and enantioselectivity under exceedingly mild reaction conditions. With (S)-5-oxoproline as the chiral ligand, activated and non-activated olefins were suitable substrates for the electro-C–H activations. Dual catalysis was devised in terms of electro-C–H olefination, along with catalytic hydrogenation. Challenging enantiomerically-enriched chiral anilide scaffolds were thereby obtained with high levels of enantio-control in the absence of toxic and cost-intensive silver salts. The resource-economy of the transformation was even improved by directly employing renewable solar energy.
Introduction
Organic electrochemistry has recently emerged as a transformative platform in molecular syntheses,1 enabling the use of electrons as sustainable redox reagents within electron-catalysis manifolds.2 Regio- and chemo-selective transformations have thus been accomplished by dialling in the appropriate electrolysis potential. However, despite recent advances in asymmetric electrochemistry,3 enantioselective electrocatalysis continues to be scarce. This can be ascribed to key challenges, such as electrochemical degradation of the catalyst or of the chiral ligand, while unfavourable interactions of the electrolyte within the enantio-determining transition state need to be fully controlled to achieve full selectivity control.
Chiral axis represent key scaffolds in natural products, biologically active compounds, chiral ligands and functional materials.4 Particularly, biaryls are omnipresent and their syntheses have been accomplished by means of cross-coupling, arene assembly and the functionalization of racemic or prochiral biaryls, among others.5 In sharp contrast, the asymmetric assembly of molecules bearing an acyclic anilide motif with axial chirality has arguably received significantly less attention.6 As this structural moiety has a greater degree of rotational freedom than have biaryls, the control of the enantioselectivity is considerably more difficult. Thus, exceedingly mild reaction conditions are required to ensure effective chiral induction, as well as to warrant the atropostability of the thus-obtained products. In this regard, Curran and Taguchi have made pioneering contributions towards the efficient catalytic asymmetric synthesis of such compounds.7 The efficiency of the enantioinduction was improved by inter alia nitrogen-functionalizations,8 cycloadditions for arene construction,9 organocatalytic nucleophilic additions10 or C–H functionalizations by dynamic kinetic resolution, with major advances by Shi.11 Contrasting with this indisputable progress, these strategies suffer from the use of prefunctionalized substrates or – in the latter case of C–H activations – from stoichiometric amounts of expensive and toxic strong silver(I) chemical oxidants, giving rise to undesirable by-products in stoichiometric quantities. This significantly compromises the resource-economy of the overall strategy.12
In this context, we very recently reported on the asymmetric metalla-electrocatalyzed C–H activation enabled by a transient directing group, that is through substrate controlled selectivity.13 In sharp contrast, we have now developed a strategy for asymmetric electrocatalysis via electro-catalyst control to access synthetically-meaningful axially-chiral anilides by electro-oxidative palladium(II)-catalyzed C–H activation (Fig. 1). Hence, dynamic kinetic resolution provided access to a wide range of chiral anilides with a readily available and inexpensive chiral ligand, featuring outstanding levels of resource-economy, electricity as the sacrificial oxidant, and molecular hydrogen as the only by-product.
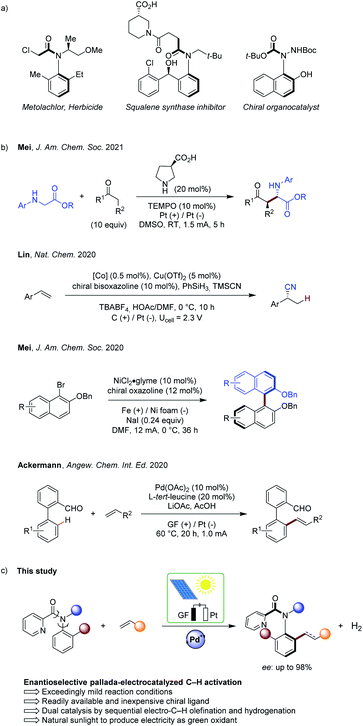 |
| Fig. 1 (a) Examples of chiral anilides in crop protection, pharmaceuticals and asymmetric synthesis. (b) Recent examples of asymmetric organic electrocatalysis. (c) Enantioselective electro-catalyzed C–H activation to access chiral anilides by electrochemistry. | |
Results and discussion
Optimisation
We initiated our studies by probing the enantioselective C–H olefination of N-benzyl-N-(2-isopropylphenyl)picolinamide (1a) with tert-butyl acrylate (2a). We were pleased to note that under a 4.0 mA constant current electrolysis (CCE), the expected product 3aa could be isolated in 11% yield and with 78% ee (Table 1, entry 2).14 The use of catalytic amounts of the redox-mediator15 1,4-benzoquinone (BQ) proved to be beneficial to improve the efficacy, to give the product 3aa in 78% yield and with 97% ee (entries 3–6), which is likely due to a stabilization of palladium(0) species. The catalysis was efficient both under air or under a nitrogen atmosphere (entries 3 and 7). Addition of an electrolyte did not affect the reaction outcome (entry 8). At 60 °C, the enantioselectivity of the reaction remained unchanged (entry 1). However, a slight decrease in enantioselectivity was found at an elevated temperature of 70 °C and an increased current of 6 mA (entry 9). At 60 °C, increasing the current to 6 mA did not alter the asymmetric olefination (entry 10), yet a lower current decreased the yield significantly (entry 11).
Table 1 Optimization of the atroposelective electrocatalyzed C–H olefinationa
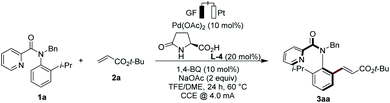
|
Entry |
Deviation from standard conditions |
Yield [%] |
ee [%] |
Reaction conditions: undivided cell, 1a (0.5 mmol), 2a (1.5 mmol), [Pd] (10 mol%), L-4 (20 mol%), 1,4-BQ (10 mol%), NaOAc (1.0 mmol), 2,2,2-trifluoroethanol (2.5 mL), DME (2.5 mL), 24 h, graphite felt (GF) anode, Pt-plate cathode, isolated yields.
Constant anodic potential with a silver as the reference electrode.
|
1 |
No deviation |
90 |
98 |
2 |
50 °C, no 1,4-BQ |
11 |
78 |
3 |
50 °C |
78 |
97 |
4 |
50 °C, Cu(OAc)2·H2O instead of 1,4-BQ |
32 |
76 |
5 |
50 °C, (4-BrC6H4)3N instead of 1,4-BQ |
22 |
86 |
6 |
50 °C, ferrocene instead of 1,4-BQ |
57 |
96 |
7 |
50 °C, under N2 atmosphere |
65 |
98 |
8 |
50 °C, adding n-Bu4NPF6 (2 equiv.) |
60 |
98 |
9 |
70 °C, 6 mA instead of 4 mA |
70 |
96 |
10 |
6 mA instead of 4 mA |
88 |
97 |
11 |
2 mA instead of 4 mA |
51 |
98 |
12 |
Divided cell setup |
45 |
95 |
13 |
CPEb @ 0.8 V |
63 |
96 |
14 |
CPEb @ 0.4 V |
81 |
97 |
The efficiency of the electrocatalysis dropped when using a divided cell setup (entry 12). Finally, we noted that the reaction also proceeded in a constant potential electrolysis (CPE) mode and a high efficiency and very good enantioselectivity was observed at 0.4 V (entries 13 and 14). Control experiments confirmed the essential role of the electricity and the palladium catalyst.14
Robustness
With the optimized electrocatalysis conditions in hand, we next studied the scope of the atropoenantioselective C–H olefination of anilides 1 (Scheme 1). First, we explored the reaction of different olefinic partners 2. Various acrylates 2a–2c proved thereby amenable to obtain products 3 with excellent enantioselectivities. Vinyl-ketones 2d and tertiary or secondary amides 2e and 2i were well-tolerated, as were styrenes 2f and 2g. Fluorinated styrene 2g allowed to access the expected product 3ag in 65% yield and with 94% ee. Vinyl phosphonates 2h could successfully be used likewise. Olefins derived from natural products, such as menthol (2j) or linalool (2k), were also very well-tolerated.
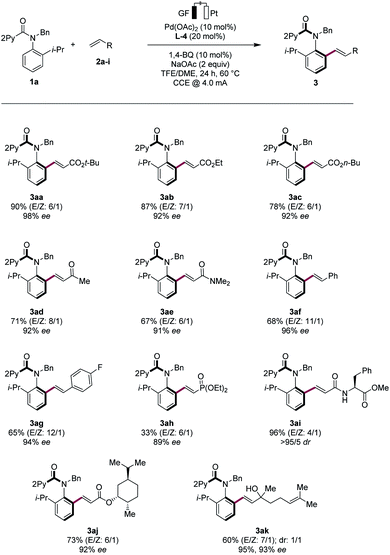 |
| Scheme 1 Scope of the reaction regarding the olefin partner. | |
Second, challenging allylations were probed, starting from non-activated alkenes 2l and 2m. Thereby, products 3al and 3am were obtained respectively, reflecting the robust scope of the pallada-electrocatalysis (Scheme 2).
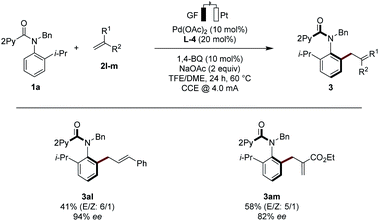 |
| Scheme 2 Atropoenantioselective C–H allylation by electrochemistry. | |
Non-activated alkenes 1-octene (2n) and 9-decen-1-ol (2o) were also identified as suitable substrates for the enantioselective pallada-electrocatalysis. Here, subsequent stirring of the electrocatalysis mixture under an atmosphere of hydrogen provided access to the alkylated products 3an and 3ao with high enantioselectivities (Scheme 3). The mild nature of the palladaelectro-catalyzed C–H functionalization was reflected by the full tolerance of the free hydroxyl group in substrate 2o.
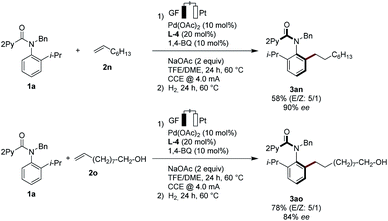 |
| Scheme 3 Dual electrocatalysis and hydrogenation manifold. | |
Third, various N-substituted anilides 1b–h were tested in the electrocatalytic asymmetric C–H olefination. Replacing the isopropyl ortho-substituent by a naphthyl group gave the desired product 3ia in 65% yield and 88% ee, while a methyl group led to a slight decrease in the enantioselectivity. However, by lowering the electrocatalysis temperature to 40 °C, we achieved a high enantioselectivity. Both electron-donating and electron-withdrawing groups were well tolerated on the pyridine motif, as was evidenced by the successful use of the anilides 1k–o (Scheme 4).
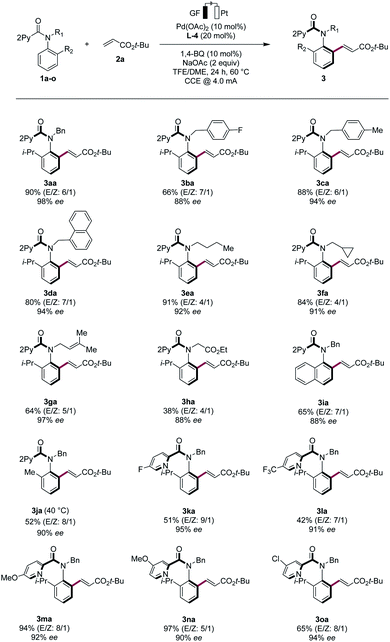 |
| Scheme 4 Scope of the reaction regarding the anilide partner. | |
To significantly improve the resource-economy12 of our strategy, we next performed the constant current electrolysis solely powered by renewable solar energy.16 To this end, we employed commercially available photovoltaic cells as the only power supply, guaranteeing high activity and enantioselectivity, when directly using natural sunlight (Scheme 5).
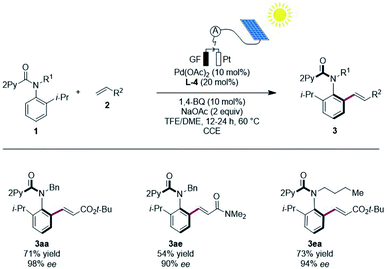 |
| Scheme 5 Renewable solar energy for atroposelective C–H olefinations. | |
Mechanistic studies
Finally, we became interested in unravelling the mode of action of the asymmetric pallada-electrocatalysis. Given that bimetallic Pd–Ag or Ag–Ag species have previously been suggested to facilitate the C–H activations,17 we hypothesized whether electrocatalysis would not only improve the sustainability, but also change the nature of the rate-determining step. In line with this hypothesis, a kinetic isotope effect (KIE) of kH/kD ≈ 1.3 was suggestive of a fast C–H metalation step (Scheme 6a),14 contrasting with a KIE of 2.3 when employing silver(I) oxidants.11b Qualitative in operando infrared spectroscopy allowed us to unravel a short induction period (Scheme 6b).14 Furthermore, we performed detailed studies by means of cyclic voltammetry (Scheme 6c).14 Thus, we observed a decrease of the onset oxidation potential in the presence of BQ, indicating its beneficial effect on the anodic oxidation. Thus, the BQ is proposed to serve a dual role, namely as a redox mediator as well as a ligand to stabilize palladium(0) species. A current dependence was next explored within a range from 1.0 to 6.0 mA (Scheme 6d). These findings were indicative of the electron transfer step being the rate limiting step, with a plateau being reached above 4.0 mA.
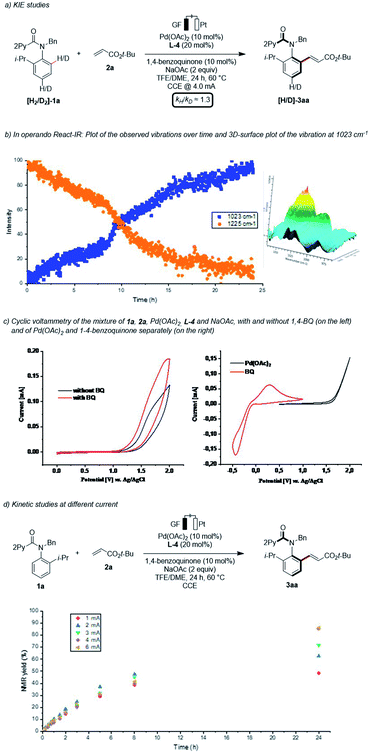 |
| Scheme 6 Key mechanistic experiments. Cyclic voltammetry measurements were recorded in TFE/DME (1/1) at a substrate concentration of 5.0 mM and with 0.1 M n-Bu4NPF6 as supporting electrolyte. The scan rate is 100 mV s−1.14 | |
Conclusions
We have reported on unprecedented catalyst-controlled atroposelective pallada-electrocatalyzed C–H activations, which provide access to synthetically-meaningful chiral anilides without stoichiometric amount of chemical oxidants. 1,4-Benzoquinone was identified as the catalytic redox-mediator of choice, with molecular hydrogen as the only stoichiometric by-product. Thereby, an electrochemical degradation of the palladium catalyst could be avoided with sodium acetate as additive, serving both as a base and as an electrolyte without altering the enantioselectivity. Overall, we accessed enantioenriched anilides with high levels of enantioselectivity under exceedingly mild reaction conditions. Dual catalysis proved to be likewise viable, allowing electrocatalyzed C–H olefination, along with subsequent hydrogenation. The asymmetric electrocatalysis employed electricity as a sustainable oxidant, and could even be carried out using a commercial solar panel with natural sunlight as the sole power source.
Data availability
All experimental data, procedures for data analysis and pertinent data sets are provided in the ESI.†
Author contributions
J. F. and L. A. conceived the project, J. F. and X. H. performed the experiments, X. H. performed CV studies. J. F. and L. A. wrote the manuscript.
Conflicts of interest
There are no conflicts to declare.
Acknowledgements
Generous support by the ERC Advanced Grant no. 101021358 (conferred on L. A.), DFG (Gottfried-Wilhelm-Leibniz award to L. A.) and the CSC (PhD fellowship to X. H.) is gratefully acknowledged.
Notes and references
- For representative reviews on organic electrochemistry, see:
(a) L. F. T. Novaes, J. Liu, Y. Shen, L. Lu, J. M. Meinhardt and S. Lin, Chem. Soc. Rev., 2021, 50, 7941–8002 RSC
;
(b) C. Ma, P. Fang, D. Liu, K.-J. Jiao, P.-S. Gao, H. Qiu and T.-S. Mei, Chem. Sci., 2021, 12, 12866–12873 RSC
;
(c) P. Gandeepan, L. H. Finger, T. H. Meyer and L. Ackermann, Chem. Soc. Rev., 2020, 49, 4254–4272 RSC
;
(d) Y.-K. Xing, Q.-L. Yang, H. Qiu and T.-S. Mei, Acc. Chem. Res., 2020, 53, 300–310 CrossRef
;
(e) J. C. Siu, N. Fu and S. Lin, Acc. Chem. Res., 2020, 53, 547–560 CrossRef CAS PubMed
;
(f) T. H. Meyer, I. Choi, C. Tian and L. Ackermann, Chem, 2020, 6, 2484–2496 CrossRef CAS
;
(g) P. Wang, X. Gao, P. Huang and A. Lei, ChemCatChem, 2020, 12, 27–40 CrossRef CAS
;
(h) R. Francke and R. D. Little, ChemElectroChem, 2019, 6, 4373–4382 CrossRef CAS
;
(i) P. Xiong and H.-C. Xu, Acc. Chem. Res., 2019, 52, 3339–3350 CrossRef CAS
;
(j) T. H. Meyer, L. H. Finger, P. Gandeepan and L. Ackermann, Trends Chem., 2019, 1, 63–76 CrossRef CAS
;
(k) A. Wieben, T. Gieshoff, S. Möhle, E. Rodrigo, M. Zirbes and S. R. Waldvogel, Angew. Chem., Int. Ed., 2018, 57, 5594–5619 CrossRef PubMed
;
(l) S. Tang, Y. Liu and A. Lei, Chem, 2018, 4, 27–45 CrossRef CAS
;
(m) M. D. Kärkäs, Chem. Soc. Rev., 2018, 47, 5786–5865 RSC
;
(n) K. D. Moeller, Chem. Rev., 2018, 118, 4817–4833 CrossRef CAS PubMed
;
(o) N. Sauermann, T. H. Meyer, Y. Qiu and L. Ackermann, ACS Catal., 2018, 8, 7086–7103 CrossRef CAS
;
(p) K.-J. Jiao, C. Ma, P. Fang and T.-S. Mei, ACS Catal., 2018, 8, 7179–7189 CrossRef
;
(q) N. Sauermann, T. H. Meyer and L. Ackermann, Eur. J. Chem., 2018, 24, 16209–16217 CrossRef CAS
;
(r) M. Yan, Y. Kawamata and P. S. Baran, Chem. Rev., 2017, 117, 13230–13319 CrossRef CAS PubMed
;
(s) A. Jutand, Chem. Rev., 2008, 108, 2300–2347 CrossRef CAS
.
- A. Studer and D. P. Curran, Nat. Chem., 2014, 6, 765–773 CrossRef CAS PubMed
.
-
(a) Z.-H. Wang, P.-S. Gao, X. Wang, J.-Q. Gao, X.-T. Xu, Z. He, C. Ma and T.-S. Mei, J. Am. Chem. Soc., 2021, 143, 15599–15605 CrossRef CAS PubMed
;
(b) X. Chang, Q. Zhang and C. Guo, Angew. Chem., Int. Ed., 2020, 59, 12612–12622 CrossRef CAS PubMed
;
(c) L. Song, N. Fu, B. G. Ernst, W. H. Lee, M. O. Frederick, R. A. DiStasio Jr and S. Lin, Nat. Chem., 2020, 12, 747–754 CrossRef CAS
;
(d) H. Qiu, B. Shuai, Y.-Z. Wang, D. Liu, Y.-G. Chen, P.-S. Gao, H.-X. Ma, S. Chen and T.-S. Mei, J. Am. Chem. Soc., 2020, 142, 9872–9878 CrossRef CAS PubMed
;
(e) P.-S. Gao, X.-J. Weng, Z.-H. Wang, C. Zheng, B. Sun, Z.-H. Chen, S.-L. You and T.-S. Mei, Angew. Chem., Int. Ed., 2020, 59, 15254–15259 CrossRef CAS PubMed
;
(f) T. J. DeLano and S. E. Reisman, ACS Catal., 2019, 9, 6751–6754 CrossRef CAS PubMed
For representative reviews on asymmetric electrosynthesis and electrocatalysis, see:
(g) C. Zhu, N. W. J. Ang, T. H. Meyer, Y. Qiu and L. Ackermann, ACS Cent. Sci., 2021, 7, 415–431 CrossRef CAS
;
(h) Q. Lin, L. Li and Z. Luo, Eur. J. Chem., 2019, 25, 10033–10044 CrossRef CAS PubMed
;
(i) M. Ghosh, V. S. Shinde and M. Rueping, Beilstein J. Org. Chem., 2019, 15, 2710–2746 CrossRef CAS PubMed
.
-
(a) J. K. Cheng, S.-H. Xiang, S. Li, L. Ye and B. Tan, Chem. Rev., 2021, 121, 4805–4902 CrossRef CAS PubMed
;
(b) Y.-B. Wang and B. Tan, Acc. Chem. Res., 2018, 51, 534–547 CrossRef CAS PubMed
;
(c) J. E. Smyth, N. M. Butler and P. A. Keller, Nat. Prod. Rep., 2015, 32, 1562–1583 RSC
;
(d) E. Kumarasamy, R. Raghunathan, M. P. Sibi and J. Sivaguru, Chem. Rev., 2015, 115, 11239–11300 CrossRef CAS
;
(e) D. Parmar, E. Sugiono, S. Raja and M. Rueping, Chem. Rev., 2014, 114, 9047–9153 CrossRef CAS
;
(f) J. Yu, F. Shi and L.-Z. Gong, Acc. Chem. Res., 2011, 44, 1156–1171 CrossRef CAS PubMed
;
(g) G. Bringmann, T. Gulder, T. A. M. Gulder and M. Breuning, Chem. Rev., 2011, 111, 563–639 CrossRef CAS PubMed
;
(h) M. C. Kozlowski, B. J. Morgan and E. C. Linton, Chem. Soc. Rev., 2009, 38, 3193–3207 RSC
;
(i) Y. Chen, S. Yekta and A. K. Yudin, Chem. Rev., 2003, 103, 3155–3212 CrossRef CAS PubMed
.
-
(a) J. K. Cheng, S.-H. Xiang, S. Li, L. Ye and B. Tan, Chem. Rev., 2021, 121, 4805–4902 CrossRef CAS PubMed
;
(b) B. Zu, Y. Guo, J. Ke and C. He, Synthesis, 2021, 53, 2029–2042 CrossRef CAS
;
(c) C.-X. Liu, W.-W. Zhang, S.-Y. Yin, Q. Gu and S.-L. You, J. Am. Chem. Soc., 2021, 143, 14025–14040 CrossRef CAS
;
(d) J. Wencel-Delord, A. Panossian, F. R. Leroux and F. Colobert, Chem. Soc. Rev., 2015, 44, 3418–3430 RSC
.
- I. Takahashi, Y. Suzuki and O. Kitagawa, Org. Prep. Proced. Int., 2014, 46, 1–23 CrossRef CAS
.
-
(a) J. Terauchi and D. P. Curran, Tetrahedron: Asymmetry, 2003, 14, 587–592 CrossRef CAS
;
(b) O. Kitagawa, M. Kohriyama and T. Taguchi, J. Org. Chem., 2002, 67, 8682–8684 CrossRef CAS PubMed
.
-
(a) L.-P. Chen, J.-F. Chen, Y.-J. Zhang, X.-Y. He, Y.-F. Han, Y.-T. Xiao, G.-F. Lv, X. Lu, F. Teng, Q. Sun and J.-H. Li, Org. Chem. Front., 2021, 8, 6067–6073 RSC
;
(b) O. Kitagawa, Acc. Chem. Res., 2021, 54, 719–730 CrossRef CAS PubMed
;
(c) D. Li, S. Wang, S. Ge, S. Dong and X. Feng, Org. Lett., 2020, 22, 5331–5336 CrossRef CAS PubMed
;
(d) Y. Kikuchi, C. Nakamura, M. Matsuoka, R. Asami and O. Kitagawa, J. Org. Chem., 2019, 84, 8112–8120 CrossRef CAS PubMed
;
(e) S.-L. Li, C. Yang, Q. Wu, H.-L. Zheng, X. Li and J.-P. Cheng, J. Am. Chem. Soc., 2018, 140, 12836–12843 CrossRef CAS PubMed
;
(f) Y. Liu, X. Feng and H. Du, Org. Biomol. Chem., 2015, 13, 125–132 RSC
;
(g) S. Shirakawa, K. Liu and K. Maruoka, J. Am. Chem. Soc., 2012, 134, 916–919 CrossRef CAS
;
(h) O. Kitagawa, M. Yoshikawa, H. Tanabe, T. Morita, M. Takahashi, Y. Dobashi and T. Taguchi, J. Am. Chem. Soc., 2006, 128, 12923–12931 CrossRef CAS PubMed
.
- K. Tanaka, K. Takeishi and K. Noguchi, J. Am. Chem. Soc., 2006, 128, 4586–4587 CrossRef CAS
.
-
(a) D. Wang, Q. Jiang and X. Yang, Chem. Commun., 2020, 56, 6201–6204 RSC
;
(b) H.-Y. Bai, F.-X. Tan, T.-Q. Liu, G.-D. Zhu, J.-M. Tian, T.-M. Ding, Z.-M. Chen and S.-Y. Zhang, Nat. Commun., 2019, 10, 3063–3071 CrossRef PubMed
;
(c) S. Brandes, M. Bella, A. Kjærsgaard and K. A. Jørgensen, Angew. Chem., Int. Ed., 2006, 45, 1147–1151 CrossRef CAS PubMed
.
-
(a) Y.-J. Wu, P.-P. Xie, G. Zhou, Q.-J. Yao, X. Hong and B.-F. Shi, Chem. Sci., 2021, 12, 9391–9397 RSC
;
(b) Q.-J. Yao, P.-P. Xie, Y.-J. Wu, Y.-L. Feng, M.-Y. Teng, X. Hong and B.-F. Shi, J. Am. Chem. Soc., 2020, 142, 18266–18276 CrossRef CAS PubMed
.
- T. H. Meyer, L. H. Finger, P. Gandeepan and L. Ackermann, Trends Chem., 2019, 1, 63–76 CrossRef CAS
.
-
(a) U. Dhawa, C. Tian, T. Wdowik, J. C. A. Oliveira, J. Hao and L. Ackermann, Angew. Chem., Int. Ed., 2020, 59, 13451–13457 CrossRef CAS PubMed
;
(b) U. Dhawa, T. Wdowik, X. Hou, B. Yuan, J. C. A. Oliveira and L. Ackermann, Chem. Sci., 2021, 12, 14182–14188 RSC
.
- For detailed information, see the ESI.†.
-
(a)
Science of Synthesis: Electrochemistry in Organic Synthesis, ed. L. Ackermann, Thieme, Stuttgart, 2021 Search PubMed
;
(b) R. Francke and R. D. Little, Chem. Soc. Rev., 2014, 43, 2492–2521 RSC
.
-
(a) T. M. Masson, S. D. A. Zondag, K. P. L. Kuijpers, D. Cambié, M. G. Debije and T. Noël, ChemSusChem, 2021, 14, 5417–5423 CrossRef CAS PubMed
;
(b) T. H. Meyer, G. A. Chesnokov and L. Ackermann, ChemSusChem, 2020, 13, 668–671 CrossRef CAS PubMed
;
(c) B. H. Nguyen, R. J. Perkins, J. A. Smith and K. D. Moeller, Beilstein J. Org. Chem., 2015, 11, 280–287 CrossRef CAS PubMed
.
-
(a) Z. Fan, K. L. Bay, X. Chen, Z. Zhuang, H. S. Park, K. Yeung, K. N. Houk and J.-Q. Yu, Angew. Chem., Int. Ed., 2020, 59, 4770–4777 CrossRef CAS PubMed
;
(b) J. Wu, N. Kaplaneris, S. Ni, F. Kaltenhäuser and L. Ackermann, Chem. Sci., 2020, 11, 6521–6526 RSC
;
(c) I. Funes-Ardoiz and F. Maseras, Eur. J. Chem., 2018, 24, 12383–12388 CrossRef CAS PubMed
;
(d) K. L. Bay, Y.-F. Yang and K. N. Houk, J. Organomet. Chem., 2018, 864, 19–25 CrossRef CAS
;
(e) M. D. Lotz, N. M. Camasso, A. J. Canty and M. S. Sanford, Organometallics, 2017, 36, 165–171 CrossRef CAS
.
Footnotes |
† Electronic supplementary information (ESI) available. See DOI: 10.1039/d1sc06135f |
‡ These authors contributed equally to this work. |
|
This journal is © The Royal Society of Chemistry 2022 |
Click here to see how this site uses Cookies. View our privacy policy here.