DOI:
10.1039/D2SC00765G
(Edge Article)
Chem. Sci., 2022,
13, 7886-7891
Copper-catalyzed radical cascade reaction of simple cyclobutanes: synthesis of highly functionalized cyclobutene derivatives†
Received
7th February 2022
, Accepted 10th June 2022
First published on 10th June 2022
Abstract
Cyclobutenes as versatile and highly valuable synthons have been widely applied in synthesis. Although various methods for their synthesis have been well established, new strategies for the construction of the cyclobutene skeleton from simple substrates are still highly desirable. Starting from simple cyclobutanes, the construction of the cyclobutene skeleton especially introducing multiple functional groups simultaneously had never been achieved. Here, we developed a novel radical cascade strategy for the synthesis of highly functionalized cyclobutenes directly from cyclobutanes involving rare cleavage of four or five C–H bonds and formation of two C–N/C–S or three C–Br bonds. With copper as catalyst and N-fluorobenzenesulfonimide (NFSI) as oxidant, a wide range of diaminated, disulfonylated and tribrominated cyclobutene derivatives were efficiently synthesized.
Introduction
Cyclobutenes are versatile and highly valuable building blocks for organic synthesis1 and widely exist as essential structural units in diverse natural products.2 Therefore, the synthesis of these compounds has been the focus of intensive research, resulting in numerous methods. The typical [2 + 2] cycloaddition between an alkyne and an alkene3–5 is still attractive. Nevertheless, electron balance between the two coupling partners was always necessitated (Scheme 1a). Other methods such as 4π-electrocyclization of conjugated dienes or vinyl-allenes,6 and cycloisomerization reactions of enynes or ene-allenyl compounds7 are effective for the construction of the cyclobutene skeleton. While most of these methods are suitable for methylenecyclobutenes or cyclobutene-fused cyclic compounds. Recently, the Maulide group8 reported an intriguing study that employed a highly active cyclobutene-fused lactone as the key intermediate undergoing a nucleophilic ring-opening process to successfully synthesize a series of functionalized cyclobutene carboxylic acid derivatives (Scheme 1b). Although the methods described above have led to great achievements for the construction of cyclobutenes, new strategies for accessing the cyclobutene skeleton from simple substrates along with introducing multiple external functional groups are highly desirable but very rare.
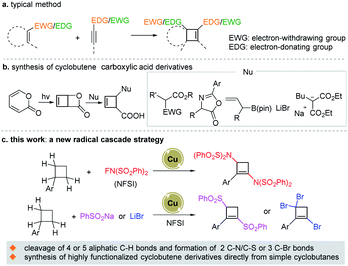 |
| Scheme 1 Synthetic methods of cyclobutene derivatives. | |
Cyclobutanes are a class of important organic synthesis intermediates widely used in various important organic transformations and total synthesis of natural products.9 According to retrosynthetic analysis, if a cyclobutane could undergo dehydrogenation and multiple C–H functionalizations, a highly functionalized cyclobutene might be assembled. There are some examples of the formation of the cyclobutene framework from cyclobutane derivatives (chloro-/bromo-cyclobutane, cyclobutanol, or cyclobutylsulfonate) via elimination reaction.10 However, so far, to the best of our knowledge, the synthesis of highly functionalized cyclobutene derivatives directly from simple cyclobutanes has never been realized. We recognized that this assumption faces three arduous challenges: (1) a relatively higher ring strain energy of cyclobutene compared with cyclobutane (ca. 30.2 kcal mol−1vs. ca. 26.3 kcal mol−1);11 (2) high bond dissociation energy (ca. 100.5 kcal mol−1) of the cyclobutane C–H bond;12 (3) highly selective hydrogen atom abstraction of several sp3 C–H bonds in cyclobutanes. With the vigorous renaissance of radical chemistry, radical cascade reactions have been employed as a powerful strategy for rapidly accessing complex molecules in one step under mild conditions.13 We supposed that multiple sp3 C–H bond radical hydrogen abstraction functionalization cascade reactions might be efficient for the synthesis of multiple functionalized cyclobutene derivatives directly from cyclobutanes. Considering our continuous interest in C–H functionalization via direct hydrogen atom abstraction,14 N-centered radical chemistry,15 and radical asymmetric ring-opening of small ring compounds,16 herein, with copper as a catalyst and N-fluorobenzenesulfonimide (NFSI) as an oxidant and nitrogen source, an efficient method for the synthesis of 1,3-diaminocyclobutene derivatives from cyclobutanes via a radical cascade process was developed. Furthermore, the Cu catalyst/NFSI system was successfully applied to synthesize 1,3-disulfonyl- or 1,3,3-tribromocyclobutene derivatives (Scheme 1c). These transformations involved the highly selective cleavage of four or five C–H bonds and the formation of two C–N/C–S or three C–Br bonds, straightforwardly synthesizing a series of highly functionalized cyclobutene derivatives, which cannot be accessed via known methods.
Results and discussion
In our previous work, by utilizing a copper catalyst/NFSI system, efficient hydrogen atom abstraction and radical amination could be realized.14a,b,15c–i,16 Therefore, in our initial research, we chose the reaction of arylcyclobutane 1a with NFSI in the presence of a Cu catalyst as the model reaction to optimize the reaction conditions. After the reaction was performed at 50 °C for 4 h using 10 mol% CuBr as the catalyst and 12 mol% phen (L1) as the ligand, we found that 1,3-diaminocyclobutene 2a was obtained in 15% yield, along with the formation of 1-aminocyclobutene 3 (6%) and E-1,3-diamino-1,3-diene 4 (5%) (Table 1, entry 1). Viewing this result, we thought that 3 might be an intermediate for the formation of 2a, and 4 should be generated from 2avia a retro-4π-electrocyclization. Therefore, 2a was treated with current conditions, giving 4 in 60% yield. Transformation of 3 was also conducted under the same conditions, and 60% 2a and 23% 4 were obtained, respectively. Next, some experimental parameters were carefully screened (for details, see ESI Table S1†). Interestingly, in the absence of the ligand, the yield of 2a was improved considerably (from 15% to 86%), and only a trace amount of 3 and a very small amount of 4 (5%) were detected (Table 1, entry 4). In order to further inhibit the retro-4π-electrocyclization of 2a, the reaction of 1a and NFSI was conducted at a lower temperature (40 °C). Delightfully, 2a was obtained in 93% yield (Table 1, entry 5). The structure of 2a was unambiguously confirmed by X-ray single-crystal analysis. Notably, the reaction of 1a and NFSI not only formed the cyclobutene skeleton but also installed two amino groups via cleavage of four aliphatic C–H bonds and construction of an sp2 C–N bond and an sp3 C–N bond. Scanning other copper catalysts (Table 1, entries 6–9) and different solvents (Table 1, entries 10–13) showed that CuBr and CH3CN were the optimal catalyst and the best solvent, respectively. A much lower reaction temperature (30 °C) could not improve the yield of 2a (Table 1, entry 14). Decreasing the amount of NFSI resulted in a lower yield of 2a (Table 1, entry 15). Satisfyingly, reducing the loading amount of CuBr to 5 mol% did not affect the yield of 2a (Table 1, entries 16 and 17).
Table 1 The optimization of reaction conditions of 1,3-diaminocyclobutene synthesisa

|
Entry |
Cu cat. |
Ligand |
Solvent |
T (°C) |
2a yield (%) |
Reaction conditions: 1a (0.2 mmol), NFSI (3 equiv.), Cu cat. (10 mol%), solvent (2 mL), N2, 4 h. Yields were determined by 1H NMR of the crude mixture with CH2Br2 as an internal standard.
3 and 4 were obtained in 6% and 5% yields, respectively.
A trace amount of 3 was observed and a very small amount of 4 (6%) was detected.
Cu(CH3CN)4PF6 was used as the catalyst.
NFSI (2.5 equiv.).
CuBr (5 mol%).
CuBr (2 mol%).
|
1b |
CuBr |
L1
|
CH3CN |
50 |
15 |
2 |
CuBr |
L2
|
CH3CN |
50 |
8 |
3 |
CuBr |
L3
|
CH3CN |
50 |
16 |
4c |
CuBr |
None |
CH3CN |
50 |
86 |
5 |
CuBr |
None |
CH3CN |
40 |
93 |
6 |
CuBr2 |
None |
CH3CN |
40 |
51 |
7 |
CuCl |
None |
CH3CN |
40 |
65 |
8 |
CuOAc |
None |
CH3CN |
40 |
44 |
9 |
Cud |
None |
CH3CN |
40 |
45 |
10 |
CuBr |
None |
DCM |
40 |
0 |
11 |
CuBr |
None |
DCE |
40 |
0 |
12 |
CuBr |
None |
THF |
40 |
Trace |
13 |
CuBr |
None |
PhCF3 |
40 |
0 |
14 |
CuBr |
None |
CH3CN |
30 |
75 |
15e |
CuBr |
None |
CH3CN |
40 |
77 |
16f |
CuBr |
None |
CH3CN |
40 |
93 |
17g |
CuBr |
None |
CH3CN |
40 |
76 |
|
With the optimal reaction conditions, we began to assess the generality of the method by investigating the substrate scope of this copper-catalyzed reaction. Diverse arylcyclobutanes were examined, efficiently synthesizing an array of 1,3-diaminocyclobutene derivatives. As shown in Scheme 2a, para-MeO-, EtO-, BnO-, and PhO-substituted arylcyclobutanes 1a–1d could be smoothly converted to the desired 1,3-diaminocyclobutenes 2a–2d in good to excellent yields (65–92%). Ortho-MeO-substituted cyclobutane 1e could also form the desired 2e in 68% yield. Para-methoxyphenylcyclobutane derivatives bearing electron-donating or electron-withdrawing groups such as MeO–, Me–, Cl–, and F– at the ortho- or meta-position of the benzene ring were competent substrates giving the corresponding 2f–2l in 66–90% yields. 4-Methoxy-1-naphthyl substituted cyclobutane 1m was effectively subjected to this reaction to produce 1,3-diaminocyclobutene 2m in 52% yield. Notably, the amido moiety worked well and delivered the expected 2n–2p in good yields. In addition, para-Ph and -F substituted benzylcyclobutanes 1q and 1r were also found to be tolerated in this transformation and formed the desired 1,3-diaminocyclobutenes 2q and 2r with good efficiency. Alkyl substituted cyclobutanes, such as benzylcyclobutane and bromomethylcyclobutane, were ineffective.
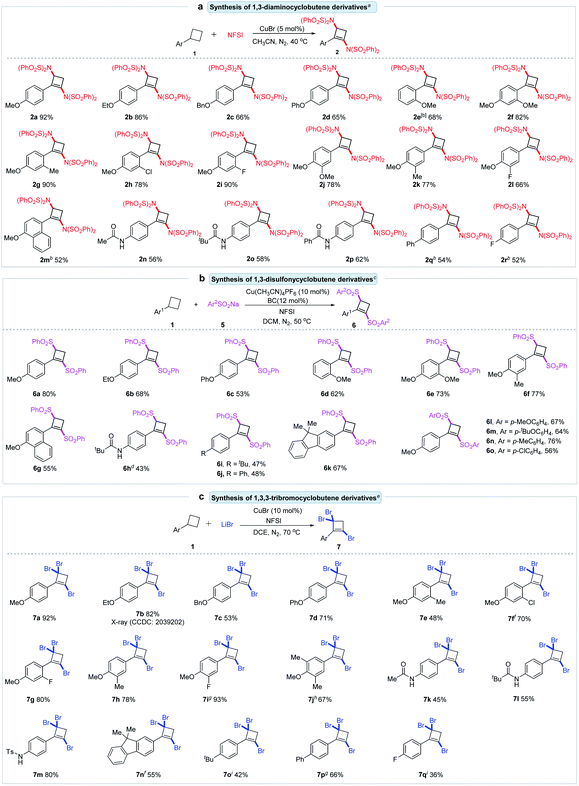 |
| Scheme 2 Synthesis of highly functionalized cyclobutene derivatives directly from simple cyclobutanes. Reaction conditions: a1 (0.2 mmol), NFSI (3 equiv.), CuBr (5 mol%), CH3CN (2 mL) at 40 °C under N2 for 4–9 h. b CuBr (10 mol%). c1 (0.2 mmol), NFSI (4 equiv.), Ar2SO2Na (4 equiv.), Cu(CH3CN)4PF6 (10 mol%), BC (2,9-dimethyl-4,7-diphenyl-1,10-phenanthroline, 12 mol%), DCM (6 mL) at 50 °C under N2 for 20 h. d Ar2SO2Na (4.5 equiv.), 60 °C. e1 (0.2 mmol), NFSI (4.3 equiv.), LiBr (3.3 equiv.), CuBr (10 mol%), DCE (2 mL) at 70 °C under N2 for 4–9 h. f TMSBr (3.3 equiv.) instead of LiBr. g LiBr (4.0 equiv.), CuBr (5 mol%), 2,2′:6′,2′′-terpyridine (6 mol%). h CuBr (5 mol%), 2,2′:6′,2′′-terpyridine (6 mol%), 60 °C. i LiBr (4.0 equiv.). Yields of isolated products are reported. | |
The sulfonyl group is a core unit of many natural products and pharmaceuticals, and the sulfonyl compounds have shown important biologically active properties.17 Based on the achieved synthesis of diamino-substituted cyclobutenes from arylcyclobutanes, we turned to synthesize disulfonyl-substituted cyclobutene derivatives. When sodium phenylsulfite (5a) was added to the solution of 1a in CH3CN in the presence of CuBr (10 mol%), phen (12 mol%) and NFSI (3.5 equiv.), the desired 1,3-disulfonylbutene 6a was obtained in 6% yield (Table S2, entry 1†). After carefully screening the reaction conditions, 6a could be obtained in 80% yield (for details, see ESI Table S2†). As summarized in Scheme 2b, alkoxyl or phenoxyl substituted arylcyclobutanes could react well with 5a to form the corresponding 1,3-disulfonylcyclobutenes 6a–6g in good yields. An acylamino-substituted arylcyclobutane is a suitable substrate, leading to 6h in acceptable yield. Tertiary butyl- and phenyl-substituted arylcyclobutenes, and 1-fluorenylcyclobutane worked smoothly under the reaction conditions to afford the disulfonylcyclobutenes 6i–6k in 47–67% yields. Other sulfites were also examined. Arylsulfites bearing both electron-donating groups, such as methoxy, tertiary butyl and methyl, and electron-withdrawing groups, such as chloride, were well tolerated in this reaction, providing corresponding 1,3-disulfonylcyclobutene derivatives 6l–6o in 56–76% yields. Alkyl sulfites, such as sodium methanesulfinate and sodium phenylmethanesulfinate failed to undergo this reaction.
This novel cascade strategy was further employed to synthesize brominated cyclobutene derivatives from cyclobutanes. When the reaction of 1a (0.2 mmol) and 2.5 equivalents of LiBr was performed in DEC (2 mL) in the presence of NFSI (3.5 equiv.) and CuBr (10 mmol%) at 50 °C for 4 h, surprisingly, 1,3,3-tribromocyclobutene 7a was obtained in 48% yield (see ESI Table S3, entry 1†). Remarkably, unlike the above diamination and disulfonylation, this bromination reaction involved cleavage of five aliphatic C–H bonds and formation of three C–Br bonds. The geminal dibromo unit as a potential carbonyl group provides a good opportunity for further chemical transformations. After carefully optimizing reaction conditions, 7a could be obtained in 92% yield (for details, see ESI Table S3†). Scheme 2c presents the scope of arylcyclobutanes for directly synthesizing a series of 1,3,3-tribromocyclobutenes. Various substituted arylcyclobutanes could react smoothly to provide the expected 2-aryl-1,3,3-tribromocyclobutenes in 36–93% yields. For example, arylcyclobutanes with an alkoxyl (1a–1c) or phenoxyl (1d) group at the para-position could undergo the bromination reaction, forming the desired 7a–7d in good to excellent yields. The single-crystal of 7b further supported the structure of the 1,3,3-tribromocyclobutene. 2/3-Substituted-4-methoxy phenylcyclobutanes could efficiently transform to the corresponding 1,3,3-tribromocyclobutenes 7e–7i in 48–93% yields. The reaction of 3,5-dimethyl-4-methoxycyclobutane could smoothly occur, providing 1,3,3-tribromocyclobutene 7j in good yield. Besides oxygen-containing groups, other groups, such as acylamido, 2-fluorenyl, tertiary butyl, biphenyl and fluorine, were compatible during this reaction, affording the corresponding 7k–7q in 36–80% yields.
To understand the reaction mechanism, some controlling experiments were conducted. The addition of 2,6-di-tert-butyl-4-methylphenol (BHT) or 2,2,6,6-tetramethylpiperidinooxy (TEMPO) was found to completely inhibit the formation of 2a. These results suggested that the reaction might involve radical intermediates. Since 1-aminocyclobutene 3 has been detected and could further transform to 2a and 4 (see conditions optimization of 1,3-diaminocyclobutene synthesis), we hypothesized that the cyclobutane might firstly form a cyclobutene. Then, the prepared cyclobutene 8a was treated with NFSI under standard conditions, furnishing 2a in 28% yield, which supported the existence of the cyclobutene intermediate. Combining all above experimental results and previous work,14,15c–h,16,18 we proposed a possible reaction mechanism. As depicted in Scheme 3, initially, the oxidation of CuI and NFSI formed CuII-coordinated nitrogen-centered radical species A or CuIII species Aʹ.
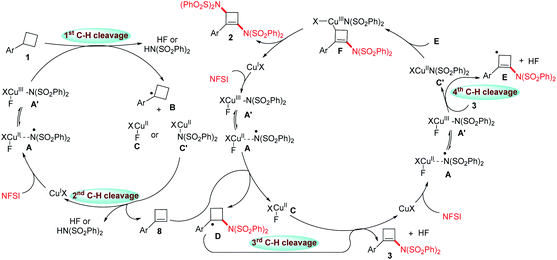 |
| Scheme 3 Proposed reaction mechanism. | |
Aʹ selectively abstracted the benzylic hydrogen atom from cyclobutane 1 to form benzylic radical B followed by β-H elimination to produce cyclobutene 8. Alternatively, B might rebound with CuII species to generate CuIII species followed by β-Cu–H elimination to generate 8.19 Subsequently, the addition of N-centered radical A to 8 and β-H elimination afforded 1-aminocyclobutene derivative 3. Then, the highly regioselective allylic hydrogen atom abstraction formed allylic radical E and CuII–N species Cʹ. The combination of Cʹ and E resulted in CuIII species F, which underwent a reductive elimination to afford 1,3-diaminocyclobutene 2, along with the regeneration of the CuI catalyst. The probable mechanisms for the formation of 1,3-disulfonylcyclobutene 6 and 1,3,3-tribromocyclobutene 7 are discussed in the ESI (see Fig. S1 and S2†). As shown in Scheme 3, the catalytic system of Cu and NFSI is very interesting, and it supports an efficient radical cascade process involving benzylic hydrogen atom abstraction, β-H elimination, radical addition, and allylic hydrogen atom abstraction/functionalization. It might open a new window for designing useful radical cascade reactions of multiple sp3 C–H bonds.
A gram-scale synthesis was carried out to demonstrate the practicability, and the target 1,3-diaminocyclobutene 2a, 1,3-disulfonylcyclobutene 6a and 1,3,3-tribromocyclobutene 7a were obtained in 85%, 68%, and 88% yields, without significant loss of efficiency (Scheme 4a). We also briefly investigated further transformation of these multi-substituted cyclobutenes (Scheme 4b). 1,3-Diaminocyclobutene 2a could smoothly undergo retro-4π-electrocyclization20 at 80 °C to stereo-specifically furnish E-1,3-diaminodiene 4 in excellent yield.21 Additionally, the geminal dibromo unit of 1,3,3-tribromocyclobutene 7a could efficiently transfer into the carbonyl group in the presence of InBr3/HBr to afford cyclobutenone 9 in good yield.22
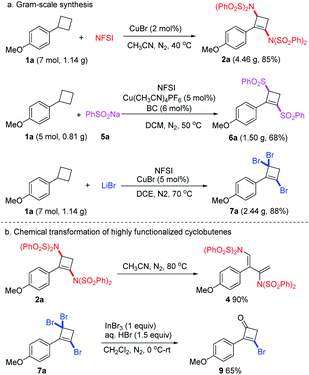 |
| Scheme 4 Gram-scale synthesis and application of the new method. | |
Conclusions
In conclusion, we have developed an unprecedented copper-catalyzed highly efficient radical cascade reaction of simple cyclobutanes, straightforwardly synthesizing diverse 1,3-diaminocyclobutenes, 1,3-disulfonylcyclobutenes, and 1,3,3-tribromocyclobutenes under mild conditions in good yields. This methodology provides the first synthesis of cyclobutene derivatives directly from cyclobutanes along with simultaneously introducing multiple external functional groups. Mechanism study showed that this novel radical cascade transformation might involve cleavage of four or five C–H bonds. Further application for the construction of diverse functionalized cyclobutenes is underway.
Data availability
All experimental and characterization data in this article are available in the ESI.† Crystallographic data for compounds 2a and 7b have been deposited in the Cambridge Crystallographic Data Centre (CCDC) under accession number CCDC 1935659 (2a) and CCDC 2039202 (7b).
Author contributions
Y. Li and Q. Zhang directed the investigations. C. Liu, Y. Li and Q. Zhang prepared the manuscript. C. Liu performed the synthetic experiments and analysed the experimental data. X. Shangguan double-checked the data in the ESI. All authors contributed to the writing of this paper.
Conflicts of interest
There are no conflicts to declare.
Acknowledgements
We thank the National Natural Science Foundation of China (22193012 and 21831002) and the Ten Thousand Talents Program for generous financial support.
Notes and references
-
(a) P. H. Coombes, E. M. Mwangi, B. K. Peters, N. R. Crouch and D. A. Mulholland, Biochem. Syst. Ecol., 2009, 37, 494–1496 CrossRef CAS
;
(b) N. González, J. Rodríguez, R. G. Kerr and C. Jiménez, J. Org. Chem., 2002, 67, 5117–5123 CrossRef PubMed
;
(c) K. M. Zuparova, B. Chommadov, M. K. Yusupov and A. S. Sadykov, Chem. Nat. Compd., 1972, 481–485 CrossRef
;
(d) J. A. Kepler, M. E. Wall, J. E. Mason, C. Bassett, A. T. McPhail and G. A. Sim, J. Am. Chem. Soc., 1967, 89, 1260–1261 CrossRef CAS
.
- J. C. Namyslo and D. E. Kaufmann, Chem. Rev., 2003, 103, 1485–1538 CrossRef CAS PubMed
.
- For selected examples of Lewis-acid catalyzed [2 + 2] cycloaddition, see:
(a) L. Shen, K. Zhao, K. Doitomi, R. Ganguly, Y.-X. Li, Z.-L. Shen, H. Hirao and T.-P. Loh, J. Am. Chem. Soc., 2017, 139, 13570–13578 CrossRef CAS PubMed
;
(b) L. Atkin, Z. Chen, A. Robertson, D. Sturgess, J. M. White and M. A. Rizzacasa, Org. Lett., 2018, 20, 4255–4258 CrossRef CAS PubMed
;
(c) T. Kang, S. Ge, L. Lin, Y. Lu, X. Liu and X. Feng, Angew. Chem., Int. Ed., 2016, 55, 5541–5544 CrossRef CAS PubMed
;
(d) K. Okamoto, T. Shimbayashi, E. Tamura and K. Ohe, Org. Lett., 2015, 17, 5843–5845 CrossRef CAS PubMed
;
(e) K. Ishihara and M. Fushimi, J. Am. Chem. Soc., 2008, 130, 7532–7533 CrossRef CAS PubMed
.
- For selected examples of transition-metal-catalyzed [2 + 2] cycloaddition, see:
(a) M. M. Parsutkar, V. V. Pagar and T. V. RajanBabu, J. Am. Chem. Soc., 2019, 141, 15367–15377 CrossRef CAS PubMed
;
(b) Y. B. Bai, Z. Luo, Y. Wang, J. M. Gao and L. Zhang, J. Am. Chem. Soc., 2018, 140, 5860–5865 CrossRef CAS PubMed
;
(c) D. Kossler and N. Cramer, Chem. Sci., 2017, 8, 1862–1866 RSC
;
(d) V. V. Pagar and T. V. RajanBabu, Science, 2018, 361, 68–72 CrossRef CAS PubMed
;
(e) A. Nishimura, M. Ohashi and S. Ogoshi, J. Am. Chem. Soc., 2012, 134, 15692–15695 CrossRef CAS PubMed
;
(f) C. Schotes and A. Mezzetti, Angew. Chem., Int. Ed., 2011, 50, 3072–3074 CrossRef CAS PubMed
;
(g) J. Treutwein and G. Hilt, Angew. Chem., Int. Ed., 2008, 47, 6811–6813 CrossRef CAS PubMed
.
- For selected examples of [2 + 2] photocycloaddition, see:
(a) S. Ha, Y. Lee, Y. Kwak, A. Mishra, E. Yu, B. Ryou and C.-M. Park, Nat. Commun., 2020, 11, 2509 CrossRef CAS PubMed
;
(b) B. L. Donnelly, L. D. Elliott, C. L. Willis and K. I. Booker-Milburn, Angew. Chem., Int. Ed., 2019, 58, 9095–9098 CrossRef CAS
;
(c) M. M. Maturi and T. Bach, Angew. Chem., Int. Ed., 2014, 53, 7661–7664 CrossRef CAS PubMed
;
(d) T. A. Zeidan, S. V. Kovalenko, M. Manoharan, R. J. Clark, I. Ghiviriga and I. V. Alabugin, J. Am. Chem. Soc., 2005, 127, 4270–4285 CrossRef CAS PubMed
;
(e) J. D. Winkler and E. C. McLaughlin, Org. Lett., 2005, 7, 227–229 CrossRef CAS PubMed
.
- For a recent review on electrocyclization of a conjugated diene, see:
(a) S. C. Coote, Eur. J. Org. Chem., 2020, 10, 1405–1423 CrossRef
; For selected examples of electrocyclization of vinylallenes, see: ;
(b) C. M. Reisinger, P. Rivera-Fuentes, S. Lampart, W. B. Schweizer and F. Diederich, Chem.–Eur. J., 2011, 17, 12906–12911 CrossRef CAS PubMed
;
(c) C. Delas, H. Urabe and F. Sato, J. Am. Chem. Soc., 2001, 123, 7937–7938 CrossRef CAS PubMed
;
(d) M. Murakami, H. Amii, K. Itami and Y. Ito, Angew. Chem., Int. Ed., 1995, 34, 1476–1478 CrossRef CAS
.
- For selected examples of cycloisomerization reactions of enynes, see:
(a) T. Iwai, M. Ueno, H. Okochi and M. Sawamura, Adv. Synth. Catal., 2018, 360, 670–675 CrossRef CAS
;
(b) X. Xin, D. Wang, F. Wu, C. Wang, H. Wang, X. Li and B. Wan, Org. Lett., 2013, 15, 4512–4515 CrossRef CAS PubMed
;
(c) M. Takachi, Y. Kita, M. Tobisu, Y. Fukumoto and N. Chatani, Angew. Chem., Int. Ed., 2010, 49, 8717–8720 CrossRef CAS PubMed
;
(d) J.-B. Xia, W.-B. Liu, T.-M. Wang and S.-L. You, Chem.–Eur. J., 2010, 16, 6442–6446 CrossRef CAS PubMed
;
(e) A. Fürstner, P. W. Davies and T. Gress, J. Am. Chem. Soc., 2005, 127, 8244–8245 CrossRef PubMed
.
- A. Misale, S. Niyomchon and N. Maulide, Acc. Chem. Res., 2016, 49, 2444–2458 CrossRef CAS PubMed
.
-
(a) H. U. Reissig and R. Zimmer, Angew. Chem., Int. Ed., 2015, 54, 5009–5011 CrossRef CAS PubMed
;
(b) T. Seiser, T. Saget, D. N. Tran and N. Cramer, Angew. Chem., Int. Ed., 2011, 50, 7740–7752 CrossRef CAS PubMed
;
(c) E. Lee-Ruff and G. Mlade-nova, Chem. Rev., 2003, 103, 1449–1484 CrossRef CAS PubMed
.
-
A. de Meijere, in Carbocyclic Four-Membered Ring Compounds, Houben-Weyl, Methods of Organic Chemistry, Thieme, Stuttgart, 1997, vol. 17e Search PubMed
.
- P. R. Khoury, J. D. Goddard and W. Tam, Tetrahedron, 2004, 60, 8103–8112 CrossRef CAS
.
- Z. Tian, A. Fattahi, L. Lis and S. R. Kass, J. Am. Chem. Soc., 2006, 128, 17087–17092 CrossRef CAS PubMed
.
- M. P. Plesniak, H.-M. Huang and D. J. Procter, Nat. Rev. Chem., 2017, 1, 0077 CrossRef
.
-
(a) Z. Ni, Q. Zhang, T. Xiong, Y. Zheng, Y. Li, H. Zhang, J. Zhang and Q. Liu, Angew. Chem., Int. Ed., 2012, 51, 1244–1247 CrossRef CAS PubMed
;
(b) T. Xiong, Y. Li, X. Bi, Y. Lv and Q. Zhang, Angew. Chem., Int. Ed., 2011, 50, 7140–7143 CrossRef CAS PubMed
;
(c) Y. Li, Z. Li, T. Xiong, Q. Zhang and X. Zhang, Org. Lett., 2012, 14, 3522–3525 CrossRef CAS PubMed
.
-
(a) T. Xiong and Q. Zhang, Chem. Soc. Rev., 2021, 50, 8857–8873 RSC
;
(b) T. Xiong and Q. Zhang, Chem. Soc. Rev., 2016, 45, 3069–3087 RSC
;
(c) H. Zhang, W. Pu, T. Xiong, Y. Li, X. Zhou, K. Sun, Q. Liu and Q. Zhang, Angew. Chem., Int. Ed., 2013, 52, 2529–2533 CrossRef CAS PubMed
;
(d) H. Zhang, Y. Song, J. Zhao, J. Zhang and Q. Zhang, Angew. Chem., Int. Ed., 2014, 53, 11079–11083 CrossRef CAS PubMed
;
(e) J. Sun, G. Zheng, T. Xiong, Q. Zhang, J. Zhao, Y. Li and Q. Zhang, ACS Catal., 2016, 6, 3674–3678 CrossRef CAS
;
(f) J. Sun, G. Zheng, Y. Fu, L. Wang, Y. Li and Q. Zhang, Org. Lett., 2018, 20, 5597–5600 CrossRef CAS PubMed
;
(g) J. Sun, G. Zheng, Q. Zhang, Y. Wang, S. Yang, Q. Zhang, Y. Li and Q. Zhang, Org. Lett., 2017, 19, 3767–3770 CrossRef CAS PubMed
;
(h) G. Zheng, Y. Li, J. Han, T. Xiong and Q. Zhang, Nat. Commun., 2015, 6, 7011 CrossRef CAS PubMed
.
-
(a) S. Yang, L. Wang, H. Zhang, C. Liu, L. Zhang, X. Wang, G. Zhang, Y. Li and Q. Zhang, ACS Catal., 2019, 9, 716–721 CrossRef CAS
;
(b) L. Wang, X. Wang, G. Zhang, S. Yang, Y. Li and Q. Zhang, Org. Chem. Front., 2019, 6, 2934–2938 RSC
;
(c) X. Wang, L. Wang, S. Yang, L. Zhang, Y. Li and Q. Zhang, Org. Biomol. Chem., 2020, 18, 4932–4935 RSC
.
- S. Y. Lin, T.-K. Yeh, C.-C. Kuo, J.-S. Song, M.-F. Cheng, F.-Y. Liao, M.-W. Chao, H.-L. Huang, Y.-L. Chen, C.-Y. Yang, M.-H. Wu, C.-L. Hsieh, W. Hsiao, Y.-H. Peng, J.-S. Wu, L.-M. Lin, M. Sun, Y.-S. Chao, C. Shih, S.-Y. Wu, S.-L. Pan, M.-S. Hung and S.-H. Ueng, J. Med. Chem., 2016, 59, 419–430 CrossRef CAS PubMed
.
-
(a) J. Li, Z. Zhang, L. Wu, W. Zhang, P. Chen, Z. Lin and G. Liu, Nature, 2019, 574, 516–521 CrossRef CAS PubMed
;
(b) W. Zhang, F. Wang, S. D. Mccann, D. Wang, P. Chen, S. S. Stahl and G. Liu, Science, 2016, 353, 1014–1018 CrossRef CAS PubMed
;
(c) M. A. Lopez, J. A. Buss and S. S. Stahl, Org. Lett., 2022, 24, 597–601 CrossRef CAS PubMed
;
(d) S.-J. Chen, D. L. Golden, S. W. Krska and S. S. Stahl, J. Am. Chem. Soc., 2021, 143, 14438–14444 CrossRef CAS PubMed
;
(e) S.-E. Suh, L. E. Nkulu, S. Lin, S. W. Krska and S. S. Stahl, Chem. Sci., 2021, 12, 10380–10387 RSC
;
(f) A. Vasilopoulos, D. L. Golden, J. A. Buss and S. S. Stahl, Org. Lett., 2020, 22, 5753–5757 CrossRef CAS PubMed
;
(g) J. A. Buss, A. Vasilopoulos, D. L. Golden and S. S. Stahl, Org. Lett., 2020, 22, 5749–5752 CrossRef CAS PubMed
;
(h) S.-E. Suh, S.-J. Chen, M. Mandal, I. A. Guzei, C. J. Cramer and S. S. Stahl, J. Am. Chem. Soc., 2020, 142, 11388–11393 CrossRef CAS PubMed
.
- For recent examples of Cu-catalyzed/mediated β-H elimination involving a radical species,
see:
(a) L. M. Stateman, R. M. Dare, A. N. Paneque and D. A. Nagib, Chem, 2022, 8, 210–224 CrossRef CAS PubMed
;
(b) N. L. Reed, G. A. Lutovsky and T. P. Yoon, J. Am. Chem. Soc., 2021, 143, 6065–6070 CrossRef CAS PubMed
.
-
R. B. Woodward and R. Hoffmann, The conservation of orbital symmetry, Academic Press, 1970 Search PubMed
.
-
4 could also be obtained in 84% yield when the temperature rose to 80 °C.
.
- B. T. Lee, T. O. Schrader, B. C. Martín-Matute, R. Kauffman, P. Zhang and M. L. Snapper, Tetrahedron, 2004, 60, 7391–7739 CrossRef CAS
.
|
This journal is © The Royal Society of Chemistry 2022 |
Click here to see how this site uses Cookies. View our privacy policy here.