DOI:
10.1039/D2SC02054H
(Edge Article)
Chem. Sci., 2022,
13, 6959-6966
Nickel-catalyzed cross-electrophile allylation of vinyl bromides and the modification of anti-tumour natural medicine β-elemene†
Received
9th April 2022
, Accepted 12th May 2022
First published on 12th May 2022
Abstract
Herein, we present a facile and efficient allylation method via Ni-catalyzed cross-electrophile coupling of readily available allylic acetates with a variety of substituted alkenyl bromides using zinc as the terminal reductant. This Ni-catalyzed modular approach displays excellent functional group tolerance and a broad substrate scope, which the creation of a series of 1,4-dienes including several structurally complex natural products and pharmaceutical motifs. Moreover, the coupling strategy has the potential to realize enantiomeric control. The practicality of this transformation is demonstrated through the potent modification of the naturally antitumor active molecule β-elemene.
Introduction
Allylic substitution reactions have been the subject of intense study,1 finding broad applications in the synthesis of natural products.2 In recent decades, vinyl allylation has been generally achieved via transition-metal-catalyzed cross-coupling of vinyl electrophiles with allyl-metallic reagents.3 And the scope of vinyl coupling partners was expanded to organomagnesium, organotin, organoboron reagents and so on, which are coupled with allylic electrophiles to realize the allylation reactions4 (Scheme 1, top). Studies in this field have resulted in numerous well-established synthetic methods by using Pd, Rh, Cu and Ni catalysts;3–6 in particular, asymmetric versions have been developed.7
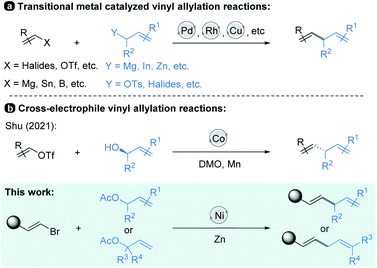 |
| Scheme 1 Allylation of vinyl reagents. | |
On the other hand, reductive cross-coupling of two electrophiles8,9 including allylation of vinyl electrophiles with allylic electrophiles affords an alternative choice by its facile carbon–carbon bond formation without pre-preparation of organometallic nucleophiles.10 For instance, Shu and co-workers recently reported a Co-catalyzed cross-electrophile protocol in which a variety of substituted chiral allylic alcohols undergo highly efficient coupling with mainly vinyl triflates (Scheme 1, middle).11 In this process, alcohols are activated in situ by dimethyl oxalate (DMO)12 through transesterification, and the benzyl oxalates formed participate in the coupling as they are generated.
Although recent years have seen significant progress in advancing the transition-metal-catalyzed vinyl allylation reactions, several challenges remain to be addressed. For example, most of the reactions are limited to the cross-coupling strategy of electrophiles with nucleophilic reagents. In addition, the present methods still suffer from a limited substrate scope and lack of further exploration for the practicality of these transformations. And no examples of the corresponding Ni-catalyzed reductive cross-coupling of vinyl electrophiles13 with allylic electrophiles have been reported. Therefore, systematic studies on the transition-metal-catalyzed coupling of substituted vinyl electrophiles with allylic electrophiles are still in need.
In this paper, we have explored a Ni-catalyzed reductive coupling of various substituted allylic acetates with a series of (E)-alkenyl bromides that efficiently generates 1,4-dienes at mild temperatures (Scheme 1, bottom). The use of the nickel catalyst, zinc and bipyridine ligand L3 (Table 1) is critical in the present study. Structurally complex substrates derived from natural products and approved drugs are well tolerated. More specifically, the vinylation of β-elemene at site 13 could enhance its anti-tumour activity successfully. In addition, we disclose the asymmetric version of the allylic vinylation through the coupling of two electrophiles, although the coupling efficiency and enantioselectivity are still low. And the preliminary mechanistic studies are also discussed.
Table 1 Optimization of the reaction conditions
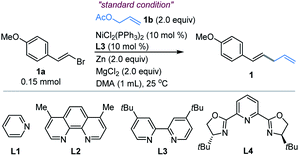
|
Entry |
Variation from standard conditions |
Yield [%]a |
NMR yield using 2,5-dimethyl furan as the internal standard from a mixture containing other impurities after a quick flash column chromatography.
Isolated yield (average of 2 independent runs).
|
1 |
None |
96 (85b) |
2 |
w/o NiCl2(PPh)3 |
Trace |
3 |
w/o Zn |
No reaction |
4 |
w/o L3 |
Trace |
5 |
w/o MgCl2 |
14 |
6 |
NiCl2 instead of NiCl2(PPh)3 |
64 |
7 |
Ni(COD)2 instead of NiCl2(PPh)3 |
17 |
8 |
Co(acac)3 instead of NiCl2(PPh)3 |
No reaction |
9 |
Mn instead of Zn |
68 |
10 |
TDAE instead of Zn |
88 |
11 |
L1 instead of L3 |
48 |
12 |
L2 instead of L3 |
33 |
13 |
L4 instead of L3 |
56 |
14 |
PPh3 instead of L4 |
34 |
15 |
5.0 mmol of 1a |
72b |
Results and discussion
Optimization of the reaction conditions
We first examined the coupling of (E)-1-(2-bromovinyl)-4-methoxybenzene 1a with allyl acetate 1b.14 The reported Ni-catalyzed conditions for allylation of aryl halides in N,N-dimethylacetamide (DMA) gave 1 in <15% yield.15 An extensive survey of the reaction conditions led us to identify that a combination of NiCl2(PPh3)2 (10 mol%), 4,4′-di-tert-butyl-2,2′-bipyridine (L3, 10 mol%) with Zn and MgCl2 in DMA at ambient temperature (25 °C) provided the coupling product 1 in an optimal 85% isolated yield (Table 1, entry 1). The control experiments indicated the necessity of Ni, Zn and ligand L3 (entries 2–4). However, without MgCl2, a significant decrease of the yield was observed (entry 5). According to the literature,16 we speculated that the role of MgCl2 is possibly to activate zinc powder by removal of salts on its surface, while other nickel sources such as NiCl2 and Ni(COD)2 were not satisfactory (entries 6–7, and Table S1†). And Co(acac)3 could not trigger this reaction, which is distinguished from Shu's work11 (entry 8). By contrast, copper salt and iron salt were unreactive (Table S1, entries 15–16†). The use of Mn and TDAE to replace Zn also generated 1 in 68% and 88% yields, respectively (entry 9–10). Replacement of L3 with other pyridine-containing additives did not improve the results (entries 11–13, and Table S2†). The ligand of PPh3 proved to be unsuitable (entry 14). The reactions at 40 °C and 60 °C led to comparable yields, while somewhat lower yield was obtained at 0 °C (Table S3, entries 8–10†). Finally, the reaction on a gram scale gave 1 in 72% isolated yield (entry 15).
Substrate scope
With the optimized conditions in hand, the allylated products 1–15 were produced in fairly good yields by the cross-coupling of (E)-1-(2-bromovinyl)-4-methoxybenzene 1a with a broad range of substituted allylic acetates (Fig. 1). The 1,4-dienes were effectively generated (e.g., 1–8) when using the primary allylic acetates. 81% yield was obtained for 2-substituted allylic acetate 2. The reaction also exhibited good coupling efficiency for 3-substituted allylic acetates within alkyl or aryl groups, as evident in 3–6 including F- and Cl-membered aryl ones. Moreover, (E)-2-methyl-3-phenylallyl acetate gave product 7 in 73% yield. The simultaneous vinylation of the diacetate was successful as exemplified by 8. However, coupling of the sterically more hindered 1-methyl-3-phenyl-allylic acetate with 1a generated product 9 in moderate yield. Finally, the allylic carbonate (10) was proved to be compatible under these reaction conditions, although a low yield was observed. Next, exposure of the 1-substituted allylic acetates to the same conditions delivered the stereoinverted products (11–15) in moderate to excellent yields (Fig. 1, bottom). These secondary and tertiary allylic acetates were tolerated well with a range of functional alkyl or unsaturated groups including methyl, n-pentyl and phenyl. The notable one includes linalyl acetate generated 15 in moderate yield, which derived from linalool. These results indicate that the reactions proceed through a similar (η3-π-allyl) nickel intermediate.15
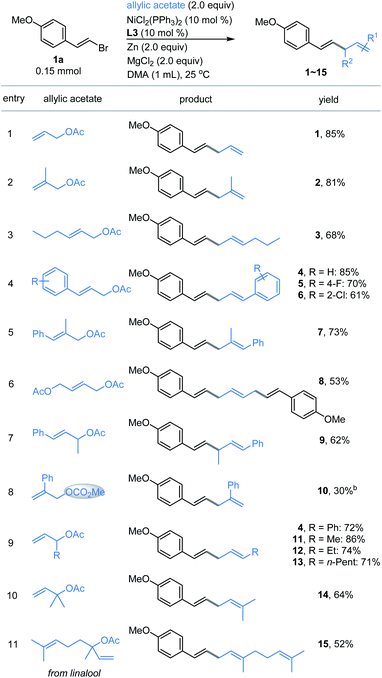 |
| Fig. 1 Scope of allylic acetates. aThe standard reaction conditions; isolated yields are provided (average of 2 independent runs). bNMR yield using 2,5-dimethyl furan as the internal reference. | |
Our attention was then shifted to the scope of the alkenyl halide partner. As shown in Fig. 2, a wide range of β-aryl-substituted (E)-alkenyl bromides bearing electron-deficient substituents such as ester, trifluoromethyl and cyano (17–20) or electron-rich substituents such as methyl, N,N-dimethyl, Bpin and methoxy (21–27) on the arene afforded the desired products smoothly. The electronic properties of alkenyl halides did not show an obvious effect on the efficiency of this transformation. Among the methoxy-substituted products (1, 24–25), the para-one was the most effective. Signally, the β-aryl-substituted (E)-alkenyl bromides bearing multi-substituents on the aromatic ring (26–27) underwent this allylation smoothly with high levels of transformation. Emphatically, (E)-alkenyl chloride (16) and (E)-alkenyl iodide (27) were shown to participate in the reaction to provide low to high yields. In addition, β-naphthyl-substituted (E)-alkenyl bromide (28) was also proved to be compatible. Good coupling results with excellent chemoselectivities were also observed for β-heteroaromatic-substituted (E)-alkenyl bromides, containing various functional groups or moieties such as quinoline (29), indole (30), benzofuran (31), thianaphthene (32) or pyridine (33). Notably, α-alkyl substituted alkenyl bromide, such as 2-bromoindene (34), is also a competent coupling partner. Ferrocene alkenyl bromide (35) could also undergo the reaction, providing access to good stereo-controlled ferrocene derivatives. However, a moderate yield was observed when 1,3-dienyl bromide (36) was used as the substrate. Notably, (Z)-alkenyl bromide (37) is a competent coupling partner, while vinyl triflates such as cyclohexenyl triflate (38) is also an active substrate with 60% yield, which indicated that the Ni-catalyzed conditions seem to be more effective than the analogous Co- or Fe-catalyzed coupling methods.10a–c,16a β-Alkyl-substituted (E)-alkenyl bromides, such as those containing a structurally complex galactose derivative (39), were also shown to be viable substrates with a slight decrease in yield. Moreover, 2,2-difluorovinyl benzene afforded the cis-product 40 in low yield.
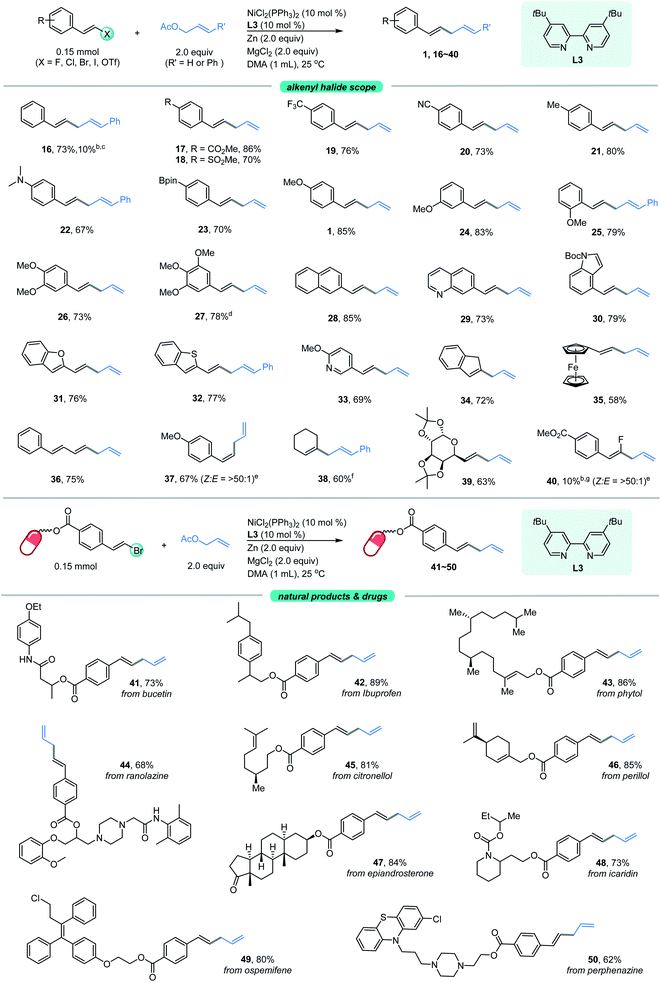 |
| Fig. 2 The scope of alkenyl halides. aThe standard reaction conditions; the isolated yields are provided (average of 2 independent runs). bNMR yield using 2,5-dimethyl furan as the internal reference. c(E)-(2-Chlorovinyl)benzene instead of alkenyl bromide. d(E)-5-(2-Iodovinyl)-1,2,3-trimethoxybenzene instead of alkenyl bromide. eThe E/Z ratio was determined by 1H NMR. fCyclohex-1-en-1-yl trifluoromethanesulfonate instead of alkenyl bromide. gMethyl 4-(2,2-difluorovinyl)benzoate instead of alkenyl bromide. | |
To further expand the practicality of this tactic, we attached the alkenyl bromide moiety to a variety of more structurally complex drugs and natural products (Fig. 2, bottom). Remarkably, all of these substrates examined in our work underwent the transformation in good to excellent yields (41–50). The reaction system is capable of distinguishing the activated alkenyl bromides from the unactivated ones,14 providing the site specific transformation. The alkenyl bromide derivatives from pharmaceuticals such as the analgesic-antipyretic bucetin (41) and ibuprofen (42), antianginal drug ranolazine (44), hormone drug epiandrosterone (47), repellent icaridin (48), selective estrogen receptor modulator ospemifene (49) and psychiatric drug perphenazine (50) were amenable to this reaction, demonstrating the viability of this nickel-catalyzed allylation for modifications of bioactive molecules. Furthermore, derivatives from naturally occurring alcohols such as phytol (43), citronellol (45) and perillol (46) were also competent substrates, enabling access to the desired adducts in synthetically useful yields. It is worth noting that many of these substrates exhibit extremely high transformation, up to 89% yield.
Natural products have always been a significant direct or indirect source of anti-tumour drugs. β-Elemene is an anti-tumour natural medicine, which is a sesquiterpene exacted from the rhizome of Traditional Chinese Medicine (TCM) Curcuma wenyujin.21 After years of experimentation and efforts,22 elemene oral emulsion (CFDA number H20010338) and elemene injection (CFDA number H10960114) developed by our research team were approved by the China Food and Drug Administration (CFDA) as broad-spectrum anti-tumour drugs in the 1990s (Fig. 3a). Although β-elemene has achieved good clinical effects and feedback, its structure still has certain defects. For example, β-elemene is a volatile oil that is insoluble in water and can only be formulated in liposome formats, and its anti-tumour activity is moderate. Therefore, it is necessary to carry out purposeful structural modifications to improve its physical and chemical properties and enhance its anti-tumour effect.
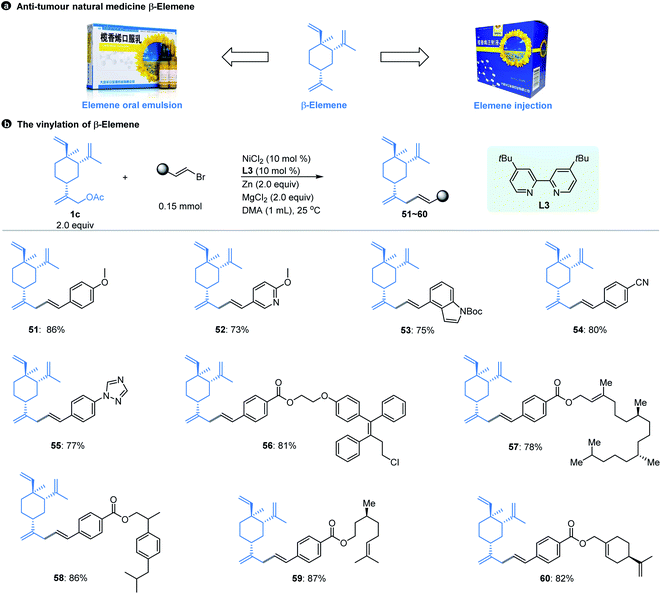 |
| Fig. 3 β-Elemene and its vinyl modification. aThe standard reaction conditions; isolated yields are provided (average of 2 independent runs). | |
However, the majority of the modifications of β-elemene reported previously are limited to the nucleophilic displacement of amino, hydroxyl or thiol groups at the allylic position due to the chemistry feasibility.23 Using the Ni-catalyzed cross-electrophile coupling method developed here, we realize for the first time the construction of a Csp3–Csp2 bond at the allylic position of β-elemene that was achieved by using the substrates of pre-acetylated β-elemene derivatives, which allows the diverse structures of β-elemene analogs to be constructed in a feasible manner. As shown in Fig. 3b, our allylic vinylation method displayed excellent compatibility for various vinyl bromides when coupling with β-elemene derived-acetate 1c. The reactions were also compatible with 1-aryl-conjugated vinyl bromides. The aryl moieties bearing a variety of functional groups in general resulted in good coupling yields (51–55). Moreover, the conversion of 1c with a series of structurally complex drug or natural product conjugated vinyl bromides was efficient as manifested by the examples of 56–60. Notably, our vinylation strategy of β-elemene at site 13 could be a potential solution to enhance its hydrophilicity by introducing the groups such as methoxy (51, 52), amino (53), triazole (55), ester (56–60), etc.
With the β-elemene vinylated compounds in hand, we subsequently investigated the in vitro anti-tumour activities of these compounds towards HCT116 (colon cancer cells) and A549 (lung cancer cells) tumour cell lines.24 After the first preliminary screening at a concentration of 50 μM, compound 55 was selected to further test its IC50 values against these two tumour cell lines. For the HCT116 cell line, compound 55 is over 15-fold more active than β-elemene (IC50: 50.9 μM and 781.9 μM for compound 55 and β-elemene, respectively, Fig. S1–S2†); for the A549 cell line, the activity of compound 55 is about 4-fold greater than that of β-elemene (IC50: 57.2 μM and 227.0 μM for compound 55 and β-elemene, respectively, Fig. S3–S4†). The above results indicate that the anti-proliferative activities of compound 55 are absolutely more potent than those of β-elemene, demonstrating that the introduction of the vinyl motif has successfully enhanced the anti-tumour effect of β-elemene, and our cross-coupling strategy is feasible for the structural modification of β-elemene in further investigation.
Next, we investigated whether the allylation strategy was possible to achieve enantioselectivity for the coupling of vinyl bromide 1a with (E)-4-phenylbut-3-en-2-yl acetate using a chiral tridentate pybox ligand L4.14 Although a moderate yield was obtained, only 24% of enantiomeric excess was observed (Fig. 4). Efforts will be continued in our lab to promote the enantioselectivity of this direct reductive coupling of vinyl bromides with allylic electrophiles.
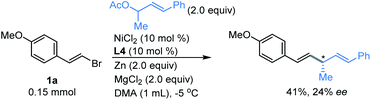 |
| Fig. 4 Asymmetric vinylation of 1,3-disubstituted allyl acetate. | |
Mechanistic consideration
In order to elucidate the underlying mechanism of this reaction, the assembly of an organozinc reagent prepared from (E)-1-(2-bromovinyl)-4-methoxybenzene 1a with allyl acetate 1b was performed in the absence of zinc powder using the standard method to examine whether an in situ Negishi process is possible. However, the corresponding allylation product was not obtained (Fig. 5, eqn (1)).14 In addition, coupling of vinyl bromide 1a with allyl acetate 1b as in Table 1, entry 4, but in the absence of a ligand mainly gave recovered starting materials.14 These results suggest that in situ formation of the organozinc/Negishi process does not seem to occur. To gain insight into the reaction details, a set of control experiments were executed (Fig. 5, eqn (2)). Considering the radical mechanism proposed by Bandar,17a radical scavengers were added into the reaction of 1a and 2a. As a result, the yield of 1 was hardly affected with either TEMPO or BHT, thus precluding the engagement of a SET-initiated radical process.17b Moreover, when the reaction was carried out under aerobic conditions, it did not work (Fig. 5, eqn (3)). These data demonstrated that the reaction was sensitive to the O2.
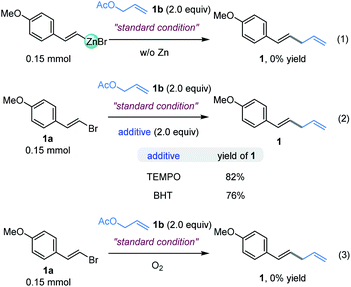 |
| Fig. 5 Mechanistic investigation and competition experiments. | |
On the basis of the results described above and the stoichiometric studies of allylic-Ni(I) with electrophiles reported by Corey, Heghdus and Gong,18 a Ni(I) to Ni(III) process may account for the catalytic pathways in the present study.19 Moreover, similar to the Co-catalyzed reductive allylation in Shu’s previous studies,11 we tentatively propose a catalytic cycle as shown in Scheme 2. First, the oxidative addition of allyl acetate to Ni(0) affords the π-allyl-Ni(II) complex. Then one electron reduction of the π-allyl-Ni(II) generates an allyl-Ni(I) intermediate by Zn.20 This intermediate undergoes the oxidative addition process with alkenyl halide which results in the allyl-Ni(III)-alkenyl species. Subsequent reductive elimination produces the desired product and generates a Ni(I) intermediate, which can be reduced to Ni(0) by Zn.
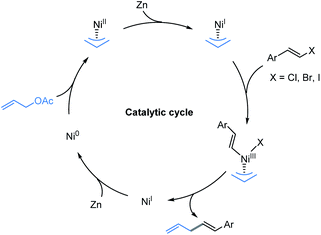 |
| Scheme 2 Proposed catalytic pathway. | |
Conclusions
In conclusion, we have reported an efficient Ni-catalyzed method for the allylation of alkenyl bromides to afford 1,4-dienes. The reaction demonstrates excellent functional group tolerance and a broad substrate scope for a variety of substituted substrates including a series of alkenyl bromide derived natural products and drugs. Significantly, this work provides a convenient tool for modification of bioactive β-elemene by facile introduction of alkenyl subunits, supporting the conclusion that this synthetic method can be a strong impetus for explorations in medicinal chemistry and chemical biology. In addition, although a low ee was observed for the 1,3-disubstituted allylic acetate, it represents the rare asymmetric version of direct reductive coupling of two electrophiles wherein in situ organometallic reagents may not be involved. Efforts to further apply this reaction, the Ni-catalyzed asymmetric cross-electrophile allylation version and further biological evaluations of synthetic products are currently in progress in our laboratory.
Data availability
All experimental data and detailed procedures are available in the ESI.†
Author contributions
Y. Y. designed the research, performed the experiments, analyzed the data, and wrote the paper. X. Q. performed the in vitro anti-tumour activity experiments and synthesized some of the allylic acetates. B. X., Y. L., H. X. and L. Z. synthesized some of the vinyl halides. X.-Y. Y. and T. X. revised the paper.
Conflicts of interest
There are no conflicts to declare.
Acknowledgements
This work was supported by the National Natural Science Foundation of China (82073686, 81730108, and 81973635), Scientific Research Foundation for Scholars of Hangzhou Normal University (2021QDL042 and 2019QDL003), the Ministry of Science and Technology of China (High-end foreign experts program, G20200217005 and G2021017004), Hangzhou City “115” plan to introduce overseas intelligence projects (20200215 and 20210120), and Hangzhou Normal University School of Medicine Teaching Reform Fund (4125b30100112).
Notes and references
- For selected examples of allylic substitution reactions, see:
(a) C. Li, J. Xing, J. Zhao, P. Huynh, W. Zhang, P. Jiang and Y. J. Zhang, Org. Lett., 2012, 14, 390–393 CrossRef CAS PubMed;
(b) H. Ohmiya, Y. Makida, D. Li, M. Tanabe and M. Sawamura, J. Am. Chem. Soc., 2010, 132, 879–889 CrossRef CAS PubMed;
(c) K. H. Shukla and P. DeShong, J. Org. Chem., 2008, 73, 6283–6291 CrossRef CAS PubMed;
(d) R. Riveiros, R. Tato, J. P. Sestelo and L. A. Sarandeses, Eur. J. Org. Chem., 2012, 3018–3023 CrossRef CAS;
(e) A. Krasovskiy, V. Malakhov, A. Gavryushin and P. Knochel, Angew. Chem., Int. Ed., 2006, 45, 6040–6044 CrossRef CAS PubMed.
- For selected reviews:
(a) B. M. Trost and M. L. Crawley, Chem. Rev., 2003, 103, 2921–2944 CrossRef CAS PubMed;
(b) Q. Cheng, H.-F. Tu, C. Zheng, J.-P. Qu, G. Helmchen and S.-L. You, Chem. Rev., 2019, 119, 1855–1969 CrossRef CAS PubMed.
- For selected examples of vinyl electrophiles:
(a) A. L. Krasovskiy, S. Haley, K. Voigtritter and B. H. Lipshutz, Org. Lett., 2014, 16, 4066–4069 CrossRef CAS PubMed;
(b) K. Lee, H. Kim, J. Mo and P. H. Lee, Chem. - Asian J., 2011, 6, 2147–2157 CrossRef CAS PubMed;
(c) Y. Baba, A. Toshimitsu and S. Matsubara, Synlett, 2008, 13, 2061–2063 Search PubMed;
(d) Y. Deng, X.-J. Wei, X. Wang, Y. Sun and T. Noel, Chem. - Eur. J., 2019, 25, 14532–14535 CrossRef CAS PubMed.
- For selected examples of allylic electrophiles:
(a) E. Alacid and C. Najera, J. Org. Chem., 2009, 74, 2321–2327 CrossRef CAS PubMed;
(b) G. W. Kabalka and M. Al-Masum, Org. Lett., 2006, 8, 11–13 CrossRef CAS PubMed;
(c) Y. Nakao, S. Ebata, J. Chen, H. Imanaka and T. Hiyama, Chem. Lett., 2007, 36, 606–607 CrossRef CAS;
(d) Y. Guo and Z. Shen, Org. Biomol. Chem., 2019, 17, 3103–3107 RSC;
(e) R. C. Larock, J. C. Bernhardt and R. J. Driggs, J. Org. Chem., 1978, 156, 45–54 CrossRef CAS.
- T. Naofumi, S. Tetsuo and I. Yoshio, Chem. Commun., 2001, 3, 237–238 Search PubMed.
-
(a) A. H. Cherney, N. T. Kadunce and S. E. Reisman, Chem. Rev., 2015, 115, 9587–9652 CrossRef CAS PubMed;
(b) G. C. Fu, ACS Cent. Sci., 2017, 3, 692–700 CrossRef CAS PubMed.
-
(a) Z. Lu and S. Ma, Angew. Chem., Int. Ed., 2008, 47, 258–297 CrossRef CAS PubMed;
(b) K. Geurts, S. P. Fletcher, A. W. van Zijl, A. J. Minnaard and B. L. Feringa, Pure Appl. Chem., 2008, 80, 1025–1037 CrossRef CAS;
(c) H. Yorimitsu and K. Oshima, Angew. Chem., Int. Ed., 2005, 44, 4435–4439 CrossRef CAS PubMed;
(d) B. M. Trost, J. Org. Chem., 2004, 69, 5813–5837 CrossRef CAS PubMed.
- For selected examples:
(a) Y. Min, G. Ma, X. Wang and H. Gong, Angew. Chem., Int. Ed., 2021, 60, 9947–9952 CrossRef CAS PubMed;
(b) Z. Zhu, Y. Gong, W. Tong, W. Xue and H. Gong, Org. Lett., 2021, 23, 2158–2163 CrossRef CAS PubMed;
(c) J. Wang, M. E. Hoerrner, M. P. Watson and D. J. Weix, Angew. Chem., Int. Ed., 2020, 59, 13484–13489 CrossRef CAS PubMed;
(d) K. Kang, L. Huang and D. J. Weix, J. Am. Chem. Soc., 2020, 142, 10634–10640 CrossRef CAS PubMed;
(e) W. M. Czaplik, M. Mayer and A. J. von Wangelin, Angew. Chem., Int. Ed., 2009, 48, 607–610 CrossRef CAS PubMed;
(f) X. Qian, A. Auffrant, A. Felouat and C. Gosmini, Angew. Chem., Int. Ed., 2011, 50, 10402–10405 CrossRef CAS PubMed;
(g) Y. He, M. Börjesson, H. Song, Y. Xue, D. Zeng, R. Martin and S. Zhu, J. Am. Chem. Soc., 2021, 143, 20064–20070 CrossRef CAS PubMed;
(h) Q. Wang, Q. Sun, Y. Jiang, H. Zhang, L. Yu, C. Tian, G. Chen and M. J. Koh, Nature Synthesis, 2022, 1, 235–244 CrossRef;
(i) Y. Li, Y. Li, L. Peng, D. Wu, L. Zhu and G. Yin, Chem. Sci., 2020, 11, 10461–10464 RSC;
(j) S. Xu, K. Wang and W. Kong, Org. Lett., 2019, 21, 7498–7503 CrossRef CAS PubMed.
- For selected reviews:
(a) J. Liu, Y. Ye, J. L. Sessler and H. Gong, Acc. Chem. Res., 2020, 53, 1833–1845 CrossRef CAS PubMed;
(b) D. J. Weix, Acc. Chem. Res., 2015, 48, 1767–1775 CrossRef CAS PubMed;
(c) C. Zhu, H. Yue, L. Chu and M. Rueping, Chem. Sci., 2020, 11, 4051–4064 RSC;
(d) Y.-N. Li, F. Xiao, Y. Guo and Y.-F. Zeng, Eur. J. Org. Chem., 2021, 1215–1228 CrossRef.
-
(a) P. Gomes, C. Gosmini and J. Périchon, Org. Lett., 2003, 5, 1043–1045 CrossRef CAS PubMed;
(b) L. L. Anka-Lufford, M. R. Prinsell and D. J. Weix, J. Org. Chem., 2012, 77, 9989–10000 CrossRef CAS PubMed;
(c) M. Mayer, W. M. Czaplik and A. J. von Wangelin, Adv. Synth. Catal., 2010, 352, 2147–2152 CrossRef CAS;
(d) A. Rudolph and M. S. Lautens, Angew. Chem., Int. Ed., 2009, 48, 2656–2670 CrossRef CAS PubMed;
(e) X. Hu, Chem. Sci., 2011, 2, 1867–1886 RSC;
(f) X.-G. Jia, P. Guo, J. Duan and X.-Z. Shu, Chem. Sci., 2018, 9, 640–645 RSC.
- W.-Y. Ma, G.-Y. Han, S. Kang, X. Pang, X.-Y. Liu and X.-Z. Shu, J. Am. Chem. Soc., 2021, 143, 15930–15935 CrossRef CAS PubMed.
-
(a) P. Guo, K. Wang, W.-J. Jin, H. Xie, L. Qi, X.-Y. Liu and X.-Z. Shu, J. Am. Chem. Soc., 2021, 143, 513–523 CrossRef CAS PubMed;
(b) Y. Ye, H. Chen, J. L. Sessler and H. Gong, J. Am. Chem. Soc., 2019, 141, 820–824 CrossRef CAS PubMed;
(c) M. Gao, D. Sun and H. Gong, Org. Lett., 2019, 21, 1645–1648 CrossRef CAS PubMed.
- For selected other cross-coupling reactions of vinyl electrophiles:
(a) Y. Ye, J. Liu, B. Xu, S. Jiang, R. Bai, S. Li, T. Xie and X.-Y. Ye, Chem. Sci., 2021, 12, 13209–13215 RSC;
(b) Y. Ye, H. Chen, K. Yao and H. Gong, Org. Lett., 2020, 22, 2070–2075 CrossRef CAS PubMed;
(c) R.-D. He, Y. Bai, G.-Y. Han, Z.-Z. Zhao, X. Pang, X. Pan, X.-Y. Liu and X.-Z. Shu, Angew. Chem., Int. Ed., 2022, 61, e202114556 CAS;
(d) X. Pang, Z.-Z. Zhao, X.-X. Wei, L. Qi, G.-L. Xu, J. Duan, X.-Y. Liu and X.-Z. Shu, J. Am. Chem. Soc., 2021, 143, 4536–4542 CrossRef CAS PubMed.
- See the ESI† for details.
- X. Cui, S. Wang, Y. Zhang, W. Deng, Q. Qian and H. Gong, Org. Biomol. Chem., 2013, 11, 3094–3097 RSC.
-
(a) S. Wang, Q. Qian and H. Gong, Org. Lett., 2012, 14, 3352–3355 CrossRef CAS PubMed;
(b) X. Wang, G. Ma, Y. Peng, C. E. Pitsch, B. J. Moll, T. D. Ly and X. Wang, J. Am. Chem. Soc., 2018, 140, 14490–14497 CrossRef CAS PubMed.
-
(a) C. Luo and J. S. Bandar, J. Am. Chem. Soc., 2019, 141, 14120–14125 CrossRef CAS PubMed;
(b) C. Zhu, M.-M. Sun, K. Chen, H. Liu and C. Feng, Angew. Chem., Int. Ed., 2021, 60, 20237–20242 CrossRef CAS PubMed.
-
(a) L. S. Hegedus and D. H. P. Thompson, J. Am. Chem. Soc., 1985, 107, 5663–5669 CrossRef CAS;
(b) E. J. Corey and M. F. Semmelhack, J. Am. Chem. Soc., 1967, 89, 2755–2757 CrossRef CAS;
(c) H. Chen, X. Jia, Y. Yu, Q. Qian and H. Gong, Angew. Chem., Int. Ed., 2017, 56, 13103–13106 CrossRef CAS PubMed.
- For cross-coupling via the Ni(I)/Ni(III) process, see:
(a) T. T. Tsou and J. K. Kochi, J. Am. Chem. Soc., 1979, 101, 7547–7560 CrossRef CAS;
(b) I. Colon and D. R. Kelsey, J. Org. Chem., 1986, 51, 2627–2637 CrossRef CAS;
(c) C. Amatore and A. Jutand, Organometallics, 1988, 7, 2203–2214 CrossRef CAS.
- S. Ikeda, K. Suzuki and K. Odashima, Chem. Commun., 2006, 457–459 RSC.
- For selected reviews:
(a) B. Zhai, N. Zhang, X. Han, Q. Li, M. Zhang, X. Chen, G. Li, R. Zhang, P. Chen, W. Wang, C. Li, Y. Xiang, S. Liu, T. Duan, J. Lou, T. Xie and X. Sui, Biomed. Pharmacother., 2019, 114, 108812 CrossRef CAS PubMed;
(b) Z. Bai, C. Yao, J. Zhu, Y. Xie, X.-Y. Ye, R. Bai and T. Xie, Molecules, 2021, 26, 1499 CrossRef CAS PubMed.
-
(a) X. Wang, Z. Liu, X. Sui, Q. Wu, J. Wang and C. Xu, Phytomedicine, 2019, 59, 152787 CrossRef CAS PubMed;
(b) H. Cheng, X. Ge, S. Zhuo, Y. Gao, B. Zhu, J. Zhang, W. Shang, D. Xu, W. Ge and L. Shi, Front. Pharmacol., 2018, 9, 1413 CrossRef CAS PubMed;
(c) X. He, X.-T. Zhuo, Y. Gao, R. Bai, X.-Y. Ye and T. Xie, R. Soc. Open Sci., 2020, 7, 200038 CrossRef CAS PubMed.
- For selected examples:
(a) G. Liu, Z. Kong and Y. Shen, Med. Chem. Res., 2013, 22, 3536–3540 CrossRef CAS;
(b) Y. Sun, G. Liu, Y. Zhang, H. Zhu, Y. Ren and Y.-M. Shen, Bioorg. Med. Chem., 2009, 17, 1118–1124 CrossRef CAS PubMed.
- R. Bai, J. Zhu, Z. Bai, Q. Mao, Y. Zhang, Z. Hui, X. Luo, X.-Y. Ye and T. Xie, J. Enzyme Inhib. Med. Chem., 2022, 37, 379–385 CrossRef CAS PubMed.
|
This journal is © The Royal Society of Chemistry 2022 |
Click here to see how this site uses Cookies. View our privacy policy here.