DOI:
10.1039/D2SC02467E
(Edge Article)
Chem. Sci., 2022,
13, 9525-9530
Activatable photothermal agents with target-initiated large spectral separation for highly effective reduction of side effects†
Received
2nd May 2022
, Accepted 5th July 2022
First published on 7th July 2022
Abstract
Photothermal agents (PTAs) with minimized side effects are critical for transforming cancer photothermal therapy (PTT) into clinical applications. However, most currently available PTAs lack true selective activation to reduce side effects because of heavy spectral overlap between photothermal agents and their corresponding products. This study reports the construction of activatable PTAs with target-initiated large spectral separation for highly effective reduction of side effects. Such designed probes involve two H2O2-activatable PTAs, aza-BOD-B1 (single activatable site) and aza-BOD-B2 (multiple activatable site). After interacting with H2O2, aza-BOD-B1 only displays a mild absorption redshift (60 nm) from 750 nm to 810 nm with serious spectral overlap, resulting in a mild photothermal effect on normal tissues upon 808 nm light irradiation. In contrast, aza-BOD-B2 displays a large absorption spectral separation (150 nm) from 660 nm to 810 nm, achieving true selective activation to minimize side effects during PTT of cancer. Besides, in vitro and in vivo investigations demonstrated that aza-BOD-B2 can specifically induce photothermal ablation of cancer cells and tumors while leaving normal sites undamaged, whereas aza-BOD-B1 exhibits undesirable side effects on normal cells. Our study provides a practical solution to the problem of undesired side effects of phototherapy, an advance in precision medicine.
Introduction
Cancer, as one of the most complicated diseases, threatens human life and health seriously.1–3 So far, the therapy methods for cancer mainly include surgery, chemotherapy, radiotherapy, etc. However, invasive surgical treatment is always accompanied by great physical pain, and radiation therapy or chemotherapy is more likely to be associated with fatal side effects. Therefore, there is a considerable demand to develop more efficient strategies to combat cancer.4–9 Photothermal therapy (PTT), a new type of tumor treatment, has attracted immense attention because of its non-invasiveness, low toxicity, real-time control, high tumor specificity, and so on.10–17 PTT treatment utilizes a photothermal reagent to produce heat upon laser irradiation for killing the cancer cells in the exposed area.18–22 Compared with traditional “always-on” photothermal agents, activatable photothermal agents will suppress cancer precisely and minimize side effects on normal tissue. Although it has been reported that various photothermal reagents can be activated by the tumor microenvironment (hypoxia,23–25 lower pH,26–29 overexpression of biological enzymes,30–32 and reactive oxygen species (ROS)33–35),the majority of them have a significant shortcoming: heavy spectral overlap between the photothermal agents and their corresponding products. That is, they are unable to achieve true selective activation and minimize side effects on adjacent normal tissues.
The photothermal agents with a single activatable site cannot change its electronic nature well before and after being activated, and thus the spectral changes are limited. We explored whether it is possible to introduce multiple activation sites into the photothermal agents and then change their structure greatly, causing a large spectral change to achieve truly selective activation. Hydrogen peroxide (H2O2) is an important and the most abundant endogenous reactive oxygen species (ROS) in cells.36,37 Compared with normal cells (the concentration of H2O2 below 5 μM), the level of H2O2 will be elevated abnormally in cancer cells,38,39 and thus, it is a good target for designed activatable photothermal agents. Previously, researchers reported a series of activatable photothermal reagents,40–45 but most of them cannot achieve true selective activation to reduce side effects. So, exploring true selective activatable photothermal reagents with minimal side effects is still quite challenging in this research field. In this contribution, taking H2O2 as an example, we proved that the introduction of multiple activation sites on the photothermal agents is an effective strategy to achieve high efficiency and minimal side effects for photothermal therapy of cancer.
Herein, activatable photothermal agents with target-initiated large spectral separation were reported for highly effective reduction of side effects. Based on the aza-BODIPY backbone (aza-BODIPY-1), near-infrared organic photothermal reagents aza-BOD-B1 (single activatable site) and aza-BOD-B2 (multi-activatable site) were rationally constructed (Scheme 1). The aza-BODIPY dye was used due to its intense adjustable near-infrared absorption, good optical stability, and large molar extinction coefficient.46–49 It's worth noting that aza-BOD-B2 with multi-activatable sites displayed a large absorption redshift (about 150 nm) from 660 nm to 810 nm upon activation by H2O2, affording the two well-separated absorption bands that benefit the true selective activation. However, aza-BOD-B1 with a single activatable site only displayed a mild absorption redshift (about 60 nm) with serious spectral overlap. Correspondingly, under the irradiation of an 808 nm laser, aza-BOD-B1 exhibits a mild photothermal effect, whereas aza-BOD-B2 has almost no photothermal effect. In contrast, the H2O2-activated product aza-BODIPY-1 displays excellent photothermal conversion efficiency. Thus, aza-BOD-B2 can act as a promising candidate for the reduction of side effects efficiently during precise PTT of cancer. In vitro investigations have demonstrated that aza-BOD-B2 is very deadly to H2O2-rich cancer cells (A549 cells) and causes minor harm to normal cells (HEK-293T cells), while aza-BOD-B1 causes significant damage to both types of cell. We further demonstrated that aza-BOD-B2 can realize precise and efficient treatment for H2O2-rich cancer cells and minimize side effects on normal tissue in a mouse model. We envisaged that the multiple-site-activated design strategy for photothermal reagents will facilitate boosting the specificity and precision of cancer photothermal therapy.
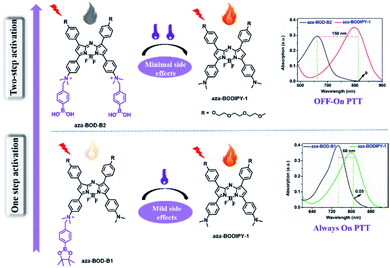 |
| Scheme 1 Rational design of NIR photothermal reagents with H2O2-activated large spectral separation for highly effective reduction of side effects during PTT of cancer. | |
Results and discussion
Synthesis of NIR photothermal regents
Based on an NIR-absorbing aza-BODIPY derivative, two near-infrared H2O2-activated photothermal regents (aza-BOD-B1 and aza-BOD-B2) were constructed by adjusting the number of the H2O2 recognition group. And the introduction of three trimethylene glycol monomethyl ether chains enhanced the water solubility of the two photothermal reagents. The synthesis route is depicted in Scheme S1.† All the chemical structures were identified by 1H NMR, 13C NMR and HRMS spectrometry.
Spectroscopic properties of aza-BOD-B1 and aza-BOD-B2
First, the spectroscopic properties of aza-BOD-B1 and aza-BOD-B2 in response to H2O2 were investigated in a buffer solution (PBS/acetonitrile = 1
:
1; pH = 7.4), respectively. As shown in Fig. 1 and S1,† the maximum absorption wavelength (λmax) of aza-BOD-B1 (single activatable site) is initially at 750 nm, which gradually redshifts to 810 nm upon the addition of H2O2 (300 μM), only displaying a slight absorption peak redshift (about 60 nm). In stark contrast, aza-BOD-B2 (multi-activatable site) exhibits a significant absorption peak redshift (about 150 nm) from 660 nm to 810 nm upon introduction with H2O2 (300 μM). Moreover, the two absorption bands of aza-BOD-B2 were well-separated at 660 nm and 810 nm. Such larger absorption spectrum changes were ascribed to the conversion of aza-BOD-B2 into aza-BODIPY-1, proven by HRMS analysis (Fig. S2†). These results indicate that the construction of photothermal reagents with multi-activatable sites can better change their electronic nature before and after being activated, resulting in larger absorption spectrum changes, which is an effective strategy to solve the problem of spectral overlap. In addition, the two activatable photothermal agents showed good response to H2O2 under weak acid conditions (pH = 6.5–7.4, Fig. S3a and S4a†), indicating their potential application in the general weakly acidic environment of tumors. The absorbance intensity changes at 808 nm exhibited a good linear correlation with increasing H2O2 concentration (0–30 μM), and the limit of detection (LOD) was calculated to be 1.36 μM which indicates high sensitivity of aza-BOD-B2 toward H2O2 (Fig. S5†). Furthermore, except for H2O2 and ONOO−, the other active substances did not cause the obvious absorption spectrum changes of the two compounds (Fig. S3b and S4b†). Importantly, the expression of ONOO− is minimal and negligible compared to H2O2 in live cells,50 Overall, aza-BOD-B1 and aza-BOD-B2 are highly feasible for application in H2O2-activated PTT. In particular, aza-BOD-B2 interaction with H2O2 has produced such a large spectral separation, making it a good candidate for precise treatment of H2O2-rich cancers.
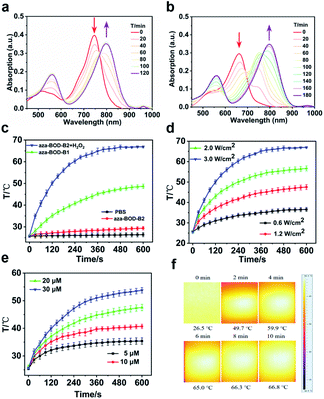 |
| Fig. 1 Time-dependent absorption changes of (a) aza-BOD-B1 (10 μM) and (b) aza-BOD-B2 (10 μM) in the presence of 300 μM H2O2. (c) Under the 808 nm laser irradiation (3.0 W cm−2), the photothermal temperature curves of PBS, aza-BOD-B1 (20 μM), aza-BOD-B2 (20 μM), and aza-BOD-B2 (20 μM) containing 300 μM H2O2. (d) The photothermal temperature change curves of aza-BOD-B2 (20 μM) activated by H2O2 under different power irradiations. (e) Temperature elevation for aza-BOD-B2 at different concentrations in the presence of H2O2 (300 μM) under 808 nm laser irradiation (1.2 W cm−2). (f) Representative IR thermal images of aza-BOD-B2 (20 μM) in the presence of H2O2 (300 μM) under 808 nm laser irradiation (3 W cm−2). Error bars represent the standard error of the mean (n = 3). | |
Photothermal conversion performance of aza-BOD-B1 and aza-BOD-B2
Based on the fact that aza-BOD-B1 and aza-BOD-B2 both showed strong NIR absorption at 808 nm activated by H2O2, an 808 nm laser was chosen for irradiation. We continue to investigate the photothermal conversion ability of aza-BOD-B1 and aza-BOD-B2 in a buffer solution (PBS/acetonitrile = 1
:
1; pH = 7.4). As shown in Fig. 1 and S6,† the temperature changes of aza-BOD-B1 and aza-BOD-B2 in the presence or absence of H2O2 are compared. Even in the absence of H2O2, the temperature of aza-BOD-B1 reached 47 °C within 10 min upon 808 nm laser irradiation (3.0 W cm−2). By prominent contrast, the temperature of aza-BOD-B2 or PBS only exhibited negligible changes under the same conditions (Fig. 1c). The results indicated that aza-BOD-B1 had a mild photothermal effect even in H2O2-deficient noncancerous tissues, whereas aza-BOD-B2 had almost no such undesired photothermal effect. It should be noted that the temperatures of aza-BOD-B1 and aza-BOD-B2 were obviously changed after H2O2 activation, indicating that H2O2 plays an important role in the transformation of aza-BOD-B1 or aza-BOD-B2 into an activatable photothermal agent. Laser power is an important factor that affects the temperature changes of photothermal reagents. As shown in Fig. 1d, the temperature of aza-BOD-B2 (20 μM) gradually increases as the power density increases, up to 66.8 °C. The temperature change process is recorded with an infrared (IR) thermal image camera (Fig. 1f). Next, we investigated the photothermal effects of different concentrations of aza-BOD-B2 in the buffer solution. In the presence of H2O2, as the concentration (5, 10, 20, and 30 μM) of aza-BOD-B2 increased, the temperature reached 35 °C, 40 °C, 45 °C, and 53 °C, respectively, upon 808 nm laser irradiation with a power density of 1.2 W cm−2, indicating that it has a good concentration dependence. In addition, time-dependent photothermal temperature changes of aza-BOD-B1 and aza-BOD-B2 after H2O2 activation under 808 nm laser irradiation (2 W cm−2) showed significant temperature enhancement as the concentration of H2O2 increased (Fig. S7†). The photothermal effects of H2O2-activated aza-BOD-B2 were not diminished even after three heating and cooling cycles, indicating good photothermal stability of aza-BOD-B2 (Fig. S6e†). The H2O2-activated photothermal conversion efficiency (η) of aza-BOD-B2 was determined to be approximately 28.3%. Besides, the two probes exhibited good photostability (Fig. S8†) in buffer solution (PBS/acetonitrile = 1
:
1, pH = 7.4). These favorable results indicate that compared with aza-BOD-B1, aza-BOD-B2 is very promising for H2O2-mediated PTT application in living tumors to reduce side effects effectively.
Photothermal cytotoxicity of aza-BOD-B1 and aza-BOD-B2in vitro
Based on the excellent photothermal conversion ability exhibited by aza-BOD-B1 and aza-BOD-B2 upon interaction with H2O2, their biocompatibility and tumor-specific therapeutic effects were investigated on H2O2-rich lung cancer cells (A549) and H2O2-deficient human embryonic kidney transformed cells (HEK-293T) using the CCK-8 assay. A549 and HEK-293T cells were treated with aza-BOD-B1 and aza-BOD-B2 in different concentrations (0, 5, 10, 20, and 30 μM) for 4 h, respectively. As shown in Fig. 2, the dark toxicity of aza-BOD-B1 and aza-BOD-B2 against A549 cells and HEK-293T cells was evaluated. The cellular activity of both cell lines remained higher than 90% even at high concentrations (30 μM) of the two compounds (Fig. 2a and b), indicating that they have good biocompatibility. However, considerable cytotoxicity was detected when A549 cells were incubated with aza-BOD-B1 or aza-BOD-B2 upon 808 nm laser irradiation. For example, the cell viability was about 40% and 20% after incubation with aza-BOD-B1 (30 μM) and aza-BOD-B2 (30 μM) upon 808 nm laser irradiation with a power density of 1.2 W cm−2 for 8 minutes of irradiation, indicating the high therapeutic efficacy of aza-BOD-B1 and aza-BOD-B2 on cancer cells upon 808 nm light irradiation. What's more, the viability of A549 cells and HEK-293T cells without aza-BOD-B1 and aza-BOD-B2 has not been affected under 808 nm laser irradiation (Fig. 2a and c). In addition, aza-BOD-B2 showed negligible dark cytotoxicity and photocytotoxicity toward normal cells (Fig. 2b and c), suggesting that aza-BOD-B2 was silent in H2O2-deficient cells, which guaranteed minimal side effects on normal tissue. Notably, aza-BOD-B1 was significantly toxic to normal cells under laser irradiation, the viability of cells being less than 50% at a concentration of 30 μM, implying that aza-BOD-B1 still exhibits a mild photothermal effect, which resulted in undesirable side effects on normal tissues inevitably. The inhibitory assay with N-acetylcysteine (NAC) at the cellular level demonstrated that the activation of these two probes was indeed due to the presence of H2O2 (Fig. S9†). These results suggested that aza-BOD-B2 is a promising tool for H2O2-rich cancer photothermal therapy with high precision and minimal side effects.
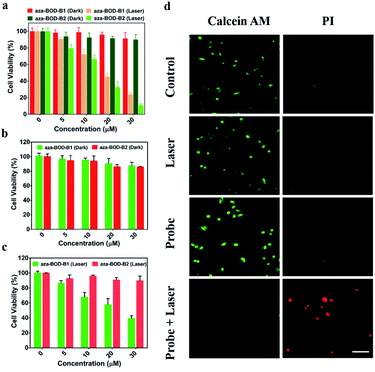 |
| Fig. 2 (a) Viability of A549 cells incubated with aza-BOD-B1 and aza-BOD-B2 in the presence or absence of 808 nm laser irradiation. Viability of HEK-293T cells incubated with aza-BOD-B1 and aza-BOD-B2 in the absence (b) or presence (c) of 808 nm laser irradiation. (d) Confocal fluorescence images of calcein AM/PI stained A549 cells with different treatments (control, laser only, aza-BOD-B2 only, and laser + aza-BOD-B2). Scale bars: 50 μm. The laser power was 1.2 W cm−2. Error bars represent the standard error of the mean (n = 5). | |
We further performed staining experiments with calcein-AM (live cells, green fluorescence) and PI (dead cells, red fluorescence) to evaluate the effective killing cancer efficacy by aza-BOD-B2-mediated photothermal therapy. In the images in Fig. 3d, A549 cells treated with only aza-BOD-B2 or 808 nm laser irradiation showed no significant cell death. The images of cells that were incubated with aza-BOD-B2 followed by irradiation with the 808 nm laser (1.2 W cm−2) showed strong red fluorescence and negligible green fluorescence, implying the superior tumor-killing effect of aza-BOD-B2 under 808 nm laser irradiation.
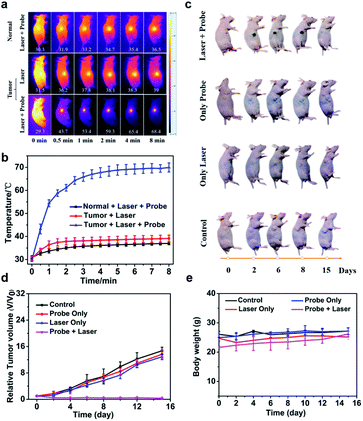 |
| Fig. 3 (a) Infrared thermal images of A549 tumor-bearing mice under continuous NIR laser irradiation with different treatments. Laser irradiation was performed at 4 h post-administration of aza-BOD-B2. (b) Mean temperature as a function of irradiation time. Laser irradiation was performed at 4 h post-administration of aza-BOD-B2. (c) Representative photos of mice in different groups during the aza-BOD-B2-mediated PTT process. (d) Tumor growth curves of mice in different groups by administration of various treatments. (e) Bodyweight changes in mice with various treatments. The laser power was 1.2 W cm−2. Error bars represent the standard error of the mean (n = 5). | |
Photothermal ablation of tumors studies in vivo
Inspired by the superior phototherapeutic results of aza-BOD-B2 on A549 cells in vitro, the capacity of aza-BOD-B2 for photothermal ablation of tumors in vivo was then evaluated using A549 tumor-bearing mice; A549 tumor-bearing mice were randomly divided into 4 groups (5 mice in each group) with different treatments (PBS, aza-BOD-B2, PBS + laser, and aza-BOD-B2 + laser). In the laser-irradiated groups, we used an 808 nm laser (1.2 W cm−2) to irradiate the tumor site directly following intratumor 4 h post-injection of PBS or aza-BOD-B2. In addition, healthy mice (without tumors) treated with both aza-BOD-B2 injection and laser irradiation were also investigated to confirm the inactive feature of aza-BOD-B2 in H2O2-deficient normal tissues. Photothermal imaging of tumor regions in A549 tumor-bearing mice injected with aza-BOD-B2 showed that the tumor temperature rapidly increased to approximately 68.4 °C under the 808 nm laser irradiation for 8 min (Fig. 3a and b). In comparison, there were slight tumor temperature changes in mice treated with only irradiation. Of note, the healthy mice injected with aza-BOD-B2 also showed a slight increase in temperature (about ∼8 °C) upon 808 nm laser irradiation. These results suggested that aza-BOD-B2 can only be specifically activated within tumors to achieve effective photothermal conversion, and thus, aza-BOD-B2 could be a promising tool for efficient therapy of tumors in vivo.
To evaluate the in vivo treatment effect of aza-BOD-B2, the important indexes including mice weight, tumor volume, and the pictures of tumor-bearing mice in different treatment groups at 2, 4, 6, 8, 10, 12, and 15 days after the first administration were recorded. As shown in Fig. 3 and S10,† no obvious therapeutic effect was observed in mice treated with only 808 nm laser irradiation or aza-BOD-B2, indicating that aza-BOD-B2 has negligible dark toxicity. By stark contrast, tumors of mice treated with both aza-BOD-B2 and laser irradiation were almost completely ablated within 15 days (Fig. 3d), suggesting excellent antitumor efficacy of aza-BOD-B2. Notably, no obvious tissue damage was observed when the healthy mice were injected with aza-BOD-B2 upon laser irradiation (Fig. S10†), which further proved that aza-BOD-B2 is highly effective in reducing side effects. Mice in each group with various treatments were dissected after 15 days; their tumors and major organs (the heart, liver, spleen, lung, and kidney) were provided for hematoxylin and eosin (H&E) staining analysis to assess the pathological damage caused by aza-BOD-B2. The pictures of tumor-bearing mice with different treatments more visually showed that tumor inhibition was significantly enhanced in the aza-BOD-B2 + laser group compared to the other treatment groups (in Fig. 3c and S10†). Importantly, H&E staining clearly showed significant necrosis in tumors of mice treated with aza-BOD-B2 and laser irradiation simultaneously, while tumors of mice treated with only aza-BOD-B2 or 808 nm laser irradiation showed no noticeable histopathological damage (Fig. S11†). In addition, the H&E images of the major organs in various treatment groups indicate minimal histopathological damage. These results indicated that aza-BOD-B2 has good biocompatibility and safety for in vivo application. Lastly, during in vivo treatments, a negligible change in mice body weight was observed in all groups (Fig. 3e). These results confirmed that aza-BOD-B2 is a highly efficient H2O2-activated photothermal agent with minimal side effects that can be specifically used for PTT treatment of tumors.
Conclusions
In summary, we developed a photothermal reagent that can achieve true selective activation to reduce side effects for realizing precise PTT therapy of cancer. We have successfully proved that the introduction of multiple activation sites on the photothermal reagent is an effective strategy to significantly eliminate side effects. aza-BOD-B1 with a single activatable site has a poor spectral separation effect after being activated by H2O2 and thus shows a mild photothermal effect under the irradiation of an 808 nm laser in normal tissues, showing undesirable side effects. In sharp contrast, aza-BOD-B2 can be activated sequentially by H2O2 to change its electronic nature well, resulting in two well-separated absorption bands with a large spectral redshift of up to 150 nm. Such significant resolution effectively avoided the problem of spectral overlap and realized true selective activation to reduce side effects upon 808 nm laser irradiation, thus no photothermal effect in normal tissues. In addition, upon activation by H2O2, aza-BOD-B2 exhibited excellent photothermal conversion efficiency under the irradiation of an 808 nm laser, enabling precise PTT treatment in tumor tissues with minimal side effects in normal tissues. In vivo tumor inhibition experiments showed that aza-BOD-B2 could remarkably ablate H2O2-rich tumor tissues after 15 days of treatment with minimal side effects. Our study solved the problems of poor specificity and large side effects of traditional photothermal reagents, opening up a new way for precision medicine and the application of photothermal reagents in clinical practice.
Data availability
All the data supporting this paper could be found in ESI.†
Author contributions
J. Sun and N. Cheng performed the synthesis experiments. N. Cheng, R. Wang, T. Zhu and J. Gao collected the optical data and analyzed the experimental data. J. Sun, X. Dong and C. Dong carried out the cell experiments. J. Sun and K. Yin investigated the tumor inhibition experiments. X. Gu and C. Zhao supervised the work and edited the manuscript.
Conflicts of interest
There are no conflicts to declare.
Acknowledgements
We gratefully acknowledge the financial support by the National Natural Science Foundation of China (21874043, 22077030, and 21977018) and the Shanghai Municipal Science and Technology Major Project (Grant No. 2018SHZDZX03).
Notes and references
- Y. Wang, S. Xu, L. Shi, C. Teh, G. Qi and B. Liu, Angew. Chem., Int. Ed., 2021, 60, 14945–14953 CrossRef CAS PubMed.
- P. Pei, T. Liu, W. Shen, Z. Liu and K. Yang, Mater. Horiz., 2021, 8, 1348–1366 RSC.
- R. Wang, K. Yin, M. Ma, T. Zhu, J. Gao, J. Sun, X. Dong, C. Dong, X. Gu, H. Tian and C. Zhao, CCS Chem., 2022 DOI:10.31635/ccschem.022.202201971.
- S. Goel, D. Ni and W. Cai, ACS Nano, 2017, 11, 5233–5237 CrossRef CAS PubMed.
- P. Zhu, Y. Chen and J. Shi, ACS Nano, 2018, 12, 3780–3795 CrossRef CAS PubMed.
- P. Huang, X. Qian, Y. Chen, L. Yu, H. Lin, L. Wang, Y. Zhu and J. Shi, J. Am. Chem. Soc., 2017, 139, 1275–1284 CrossRef CAS.
- V. Deepagan, D. You, W. Um, H. Ko, S. Kwon, K. Choi, G. Yi, J. Lee, D. Lee, K. Kim, I. Kwon and J. Park, Nano Lett., 2016, 16, 6257–6264 CrossRef CAS PubMed.
- T. Gu, T. Chen, L. Cheng, X. Li, G. Han and Z. Liu, Nano Res., 2020, 13, 2209–2215 CrossRef CAS.
- R. Wang, K. Dong, G. Xu, B. Shi, T. Zhu, P. Shi, Z. Guo, W. Zhu and C. Zhao, Chem. Sci., 2019, 10, 2785 RSC.
- X. Li, J. Lovell, J. Yoon and X. Chen, Nat. Rev. Clin. Oncol., 2020, 17, 657–674 CrossRef PubMed.
- H. Sun, Q. Zhang, J. Li, S. Peng, X. Wang and R. Cai, Nano Today, 2021, 37, 101073 CrossRef CAS.
- D. Li, X. Chen, D. Wang, H. Wu, H. Wen, L. Wang, D. Wang, J. Ji and B. Tang, Biomaterials, 2022, 283, 121476 CrossRef CAS PubMed.
- Y. Zou, W. Liu, W. Sun, J. Du, J. Fan and X. Peng, Adv. Funct. Mater., 2022, 2111853 CrossRef CAS.
- A. Schwartz-Duval, C. Konopka, P. Moitra, A. Daza, I. Srivastava, E. Johnson, T. Kampert, S. Fayn, A. Haran, L. Dobrucki and D. Pan, Nat. Commun., 2020, 11, 4530 CrossRef CAS.
- K. Wang, Y. Xiang, W. Pan, H. Wang, N. Li and B. Tang, Chem. Sci., 2020, 11, 8055 RSC.
- S. He, Y. Jiang, J. Li and K. Pu, Angew. Chem., Int. Ed., 2020, 59, 10633–10638 CrossRef CAS PubMed.
- Y. Wang, G. Xia, M. Tan, M. Wang, Y. Li and H. Wang, Adv. Funct. Mater., 2022, 2113098 CrossRef CAS.
- L. Cheng, X. Wang, F. Gong, T. Liu and Z. Liu, Adv. Mater., 2020, 32, 1902333 CrossRef CAS PubMed.
- S. Gao, G. Wei, S. Zhang, B. Zheng, J. Xu, G. Chen, M. Li, S. Song, W. Fu, Z. Xiao and W. Lu, Nat. Commun., 2019, 10, 2206 CrossRef.
- J. Zhou, L. Rao, G. Yu, T. R. Cook, X. Chen and F. Huang, Chem. Soc. Rev., 2021, 50, 2839–2891 RSC.
- X. Zhao, S. Long, M. Li, J. Cao, Y. Li, L. Guo, W. Sun, J. Du, J. Fan and X. Peng, J. Am. Chem. Soc., 2020, 142, 1510–1517 CrossRef CAS PubMed.
- Y. Xu, S. Wang, Z. Chen, R. Hu, Y. Zhao, K. Wang, J. Qu and L. Liu, Biomaterials, 2021, 276, 121017 CrossRef CAS PubMed.
- D. Zhang, Z. Cai, N. Liao, S. Lan, M. Wu, H. Sun, Z. Wei, J. Li and X. Liu, Chem. Sci., 2018, 9, 7390–7399 RSC.
- H. Wang, K. Xue, Y. Yang, H. Hu, J. Xu and X. Zhang, J. Am. Chem. Soc., 2022, 144, 2360–2367 CrossRef CAS PubMed.
- T. Zhang, X. Hou, Y. Kong, F. Yang, Y. Yue, M. Shah, H. Li, F. Huang, J. Liu and D. Guo, Theranostics, 2022, 12, 396–440 CrossRef CAS PubMed.
- Y. Li, J. Lin, P. Wang, Q. Luo, H. Lin, Y. Zhang, Z. Hou, J. Liu and X. Liu, ACS Nano, 2019, 13, 12912–12928 CrossRef CAS PubMed.
- B. Yang, Z. Dai, G. Zhang, Z. Hu, X. Yao, S. Wang, Q. Liu and X. Zheng, Adv. Healthcare Mater., 2020, 9, 1901634 CrossRef CAS PubMed.
- J. Yang, Y. Chen, Y. Li and X. Yin, ACS Appl. Mater. Interfaces, 2017, 9, 22278–22288 CrossRef CAS PubMed.
- Y. Du, D. Wang, S. Wang, W. Li and J. Suo, RSC Adv., 2021, 11, 6472–6476 RSC.
- J. Li, C. Xie, J. Huang, Y. Jiang, Q. Miao and K. Pu, Angew. Chem., Int. Ed., 2018, 57, 3995–3998 CrossRef CAS PubMed.
- X. Zhen, J. Zhang, J. Huang, C. Xie, Q. Miao and K. Pu, Angew. Chem., Int. Ed., 2018, 57, 7804–7808 CrossRef CAS PubMed.
- J. Zhang, P. Cheng and K. Pu, Bioconjugate Chem., 2019, 30, 2089–2101 CrossRef CAS PubMed.
- Y. Liu, F. Mo, J. Hu, Q. Jiang, X. Wang, Z. Zou, X. Z. Zhang, D. Pang and X. Liu, Chem. Sci., 2021, 12, 10097–10105 RSC.
- Z. Wang, Z. Xu, P. Upputuri, Y. Jiang, J. Lau, M. Pramanik, Z. Wang, X. Paul, K. Pu and B. Xing, ACS Nano, 2019, 13, 5816–5825 CrossRef CAS PubMed.
- L. Feng, R. Zhao, B. Liu, F. He, S. Gai, Y. Chen and P. Yang, ACS Appl. Mater. Interfaces, 2020, 12, 41047–41061 CrossRef CAS PubMed.
- E. Miller, A. Albers, A. Pralle, E. Isacoff and C. Chang, J. Am. Chem. Soc., 2005, 127, 16652 CrossRef CAS PubMed.
- B. Dickinson and C. Chang, J. Am. Chem. Soc., 2008, 130, 9638 CrossRef CAS PubMed.
- T. Szatkowski and C. Nathan, Cancer Res., 1991, 51, 794–798 Search PubMed.
- H. Zhu, J. Li, X. Qi, P. Chen and K. Pu, Nano Lett., 2018, 18, 586–594 CrossRef CAS PubMed.
- Y. Li, J. Lin, P. Wang, Q. Luo, H. Lin, Y. Zhang, Z. Hou, J. Liu and X. Liu, ACS Nano, 2019, 13, 12912–12928 CrossRef CAS PubMed.
- H. Wang, Q. Chen and S. Zhou, Chem. Soc. Rev., 2018, 47, 4198–4232 RSC.
- B. Shi, Q. Yan, J. Tang, K. Xin, J. Zhang, Y. Zhu, G. Xu, R. Wang, J. Chen, W. Gao, T. Zhu, J. Shi, C. Fan, C. Zhao and H. Tian, Nano Lett., 2018, 18, 6411–6416 CrossRef CAS PubMed.
- B. Shi, N. Ren, L. Gu, G. Xu, R. Wang, T. Zhu, Y. Zhu, C. Fan, C. Zhao and H. Tian, Angew. Chem., Int. Ed. Engl., 2019, 58, 16826–16830 CrossRef CAS PubMed.
- G. Xu, W. Guo, X. Gu, Z. Wang, R. Wang, T. Zhu, H. Tian and C. Zhao, CCS Chem., 2020, 2, 527–538 CrossRef CAS.
- X. Zhao, K. Zhao, L. Chen, Y. Liu, J. Liu and X. Yan, Chem. Sci., 2021, 12, 442 RSC.
- M. Li, Y. Xu, M. Zhao, F. Li, W. Feng, T. Feng, S. Liu and Q. Zhao, Inorg. Chem., 2020, 59, 17826–17833 CrossRef CAS PubMed.
- Y. Xu, S. Wang, Z. Chen, R. Hu, S. Li, Y. Zhao, L. Liu and J. Qu, J. Nanobiotechnol., 2021, 19, 37 CrossRef CAS PubMed.
- Z. Shi, X. Han, W. Hu, H. Bai, B. Peng, L. Ji, Q. Fan, L. Li and W. Huang, Chem. Soc. Rev., 2020, 49, 7533–7567 RSC.
- Y. Liu, N. Song, L. Chen, S. Liu and Z. Xie, Chem.–Asian J., 2018, 13, 989–995 CrossRef CAS PubMed.
- S. Parvez, M. J. C. Long, J. R. Poganik and Y. Aye, Chem. Rev., 2018, 118, 8798–8888 CrossRef CAS PubMed.
|
This journal is © The Royal Society of Chemistry 2022 |
Click here to see how this site uses Cookies. View our privacy policy here.