DOI:
10.1039/D2SC04346G
(Edge Article)
Chem. Sci., 2022,
13, 12374-12381
pH-Dependent transport of amino acids across lipid bilayers by simple monotopic anion carriers†
Received
4th August 2022
, Accepted 4th October 2022
First published on 5th October 2022
Abstract
The transport of amino acids across lipid membranes is vital for the proper functioning of every living cell. In spite of that, examples of synthetic transporters that can facilitate amino acid transport are rare. This is mainly because at physiological conditions amino acids predominantly exist as highly polar zwitterions and proper shielding of their charged termini, which is necessary for fast diffusion across lipophilic membranes, requires complex and synthetically challenging heteroditopic receptors. Here we report the first simple monotopic anion receptor, dithioamide 1, that efficiently transports a variety of natural amino acids across lipid bilayers at physiological pH. Mechanistic studies revealed that the receptor rapidly transports deprotonated amino acids, even though at pH 7.4 these forms account for less than 3% of the total amino acid concentration. We also describe a new fluorescent assay for the selective measurement of the transport of deprotonated amino acids into liposomes. The new assay allowed us to study the pH-dependence of amino acid transport and elucidate the mechanism of transport by 1, as well as to explain its exceptionally high activity. With the newly developed assay we screened also four other representative examples of monotopic anion transporters, of which two showed promising activity. Our results imply that heteroditopic receptors are not necessary for achieving high amino acid transport activities and that many of the previously reported anionophores might be active amino acid transporters. Based on these findings, we propose a new strategy for the development of artificial amino acid transporters with improved properties.
Introduction
The transport of amino acids (AAs) across lipid bilayers is vital for the proper functioning of every living cell. However, at physiological pH AAs exist predominantly as highly polar zwitterions, whose spontaneous diffusion through lipid bilayers is intrinsically slow. As a consequence, dozens of specialized transmembrane proteins have evolved to facilitate their transport.1 These highly sophisticated AA transporters play important roles in regulating key physiological functions, such as protein synthesis, metabolism, gene expression, redox balance and signal transduction.2 Genetically conditioned malfunction of natural AA transporters contributes to serious diseases, such as aminoacidurias, diabetes, neurodegenerative disorders, obesity, and cancer.2 Therefore, there is a pressing need to develop synthetic AA transporters which would be able to supplement or substitute the function of defective AA transporters. Such molecules might be expected to display a wide range of biological activities and also find applications in drug delivery,3 metabolism regulation,4 and as next-generation antibiotics.5
Despite the biological significance of amino acids, their transport across lipid bilayers by synthetic transporters has rarely been studied.6 This is in sharp contrast to the tremendous recent progress in the development of artificial carriers and channels for other biologically relevant polar solutes, particularly ions such as Na+, K+, Cl− and HCO3−.7 The reasons behind this lag might be traced to the inherent difficulties of transporting highly polar species containing both positively and negatively charged groups, synthetic challenges associated with the construction of heteroditopic receptors for such zwitterions, and the lack of universal and easy-to-apply assays for AA transport studies in model liposomes.
Recently, Gale and co-workers proposed a novel approach to the transmembrane transport of AAs, wherein an anion receptor binds a carboxylate moiety by an array of hydrogen bonds, whilst a lipophilic aldehyde independently forms a reversible covalent bond with an amino group.8 Here, we demonstrate that simple monotopic anion receptors may act as potent AA carriers at physiological pH, even in the absence of any auxiliary aldehyde. As a case in point, carbazole-based dithioamide 1 is shown to facilitate rapid diffusion of various proteinogenic AAs, including the most hydrophilic ones, across liposomal membranes, despite being unable to bind their ammonium/amino groups. In order to elucidate the mechanism of this surprising phenomenon, we developed a new fluorescent assay which selectively measures the influx of deprotonated AAs into liposomes, while being largely indifferent to their zwitterionic forms. This new tool allowed us to study the pH-dependence of AA transport and explain the surprisingly high activity of 1. Finally, we show that other simple monotopic receptors, such as ureas, thioureas and squaramides, are also able to transport AAs. These findings will significantly advance research relating to the transmembrane transport of AAs and facilitate the search for small, drug-like molecules capable of performing this function.
Results and discussion
Transport of amino acids at physiological pH
We have recently shown that dithioamidocarbazole 1 is an exceptionally active anion carrier capable of transporting a variety of biologically relevant anions, including hydrophilic carboxylates, across lipid bilayers.9 Encouraged by these results, we challenged this receptor with the transport of amino acids.
The ability of the dithioamide 1 and its parent diamide 210 to transport AAs into large unilamellar vesicles (LUVs) was first investigated using an indirect Cu2+–calcein assay developed by Gale and co-workers.8 Following their procedure, LUVs (mean diameter 200 nm) made of POPC/cholesterol (7
:
3), entrapping calcein (0.2 mM), CuSO4 (0.2 mM) and Na2SO4 (100 mM) were prepared and separated from the extravesicular calcein by gel filtration. The vesicles were suspended in an external solution containing AA (24 mM), CuSO4 (0.2 mM) and Na2SO4 (100 mM). Both the internal and external solutions were buffered by HEPES to pH 7.4. Transport was initiated by the addition of 1 or 2 in DMSO to the suspension of vesicles in a spectrofluorometric cuvette. AAs transported into liposomes sequester Cu2+ from the calcein complex, causing recovery of calcein fluorescence.
Using this assay, we studied the transport of all proteinogenic AAs which are both electroneutral (zwitterionic) and sufficiently soluble at physiological pH: glycine, alanine, valine, isoleucine, methionine, phenylalanine, serine, and threonine (Fig. 1). We found that all of them were transported by dithioamide 1, while the diamide analogue 2 showed no activity (Fig. 2).‡
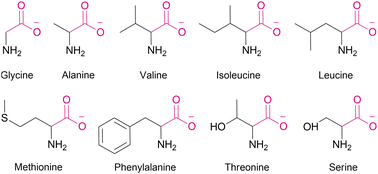 |
| Fig. 1 Anionic forms of amino acids studied in this work. | |
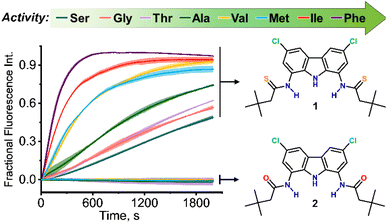 |
| Fig. 2 Amino acids transport kinetics measured by the Cu2+−calcein assay. POPC : cholesterol (7 : 3) LUVs loaded with CuSO4 (0.2 mM), calcein (0.2 mM) and Na2SO4 (100 mM), pH 7.4, were suspended in an external solution containing amino acid (24 mM), CuSO4 (0.2 mM) and Na2SO4, pH 7.4. Fluorescence intensity (λex = 495 nm, λem = 515 nm) was recorded after addition of 1 or 2 (1 mol% with respect to lipids). | |
As expected, the transport activity of 1 increases with increasing lipophilicity of AAs:11 Ser < Gly ∼ Thr < Ala < Met ∼ Val < Ile < Phe but, notably, even the most polar AA in the series, serine, is substantially transported into the vesicles within 30 min. This is particularly remarkable in view of the very simple, monotopic structure of transporter 1. In contrast, most of the literature examples of synthetic AA transporters are either large, channel forming molecules6c–f or elaborate carriers consisting of two separate domains for the recognition of the anionic and the cationic termini.6g,12 The monotopic squaramide receptor 5 (Fig. 8), used in conjunction with lipophilic aldehydes by Gale and co-workers,8 exhibited very low activity in the absence of the auxiliary aldehydes. Thus, to the best of our knowledge, 1 is the first monotopic receptor that is highly active in carrying unmodified AAs through a lipid bilayer at physiological pH.
Amino acid transport at basic pH. Development of SPQ assay
Since the highly hydrophilic, positively charged ammonium group hampers the passage of [1 ⊃ AA] through the lipid membrane, we hypothesized that 1 transports AAs in their deprotonated forms, rather than as zwitterions, despite the latter largely dominate (>97%) at physiological pH. If this is the case, AA transport should be limited not only by the low concentration of deprotonated AAs, but also by the available mechanisms of charge compensation, which in the sulfate medium are practically restricted to H+/Na+ symport or OH− antiport. In consequence, the AA transport should be much faster at high pH and in the nitrate medium. However, the Cu2+–calcein assay is not suitable for the transport studies in basic conditions or in the nitrate solution. Moreover, other literature assays, based on NMR or fluorescent labelling, are not suitable for measuring fast transport kinetics. Therefore, we developed a new fluorescent assay, suitable for studying the kinetics of AA transport in the nitrate medium and at high pH. Previously, we found that a popular anion sensitive fluorescent probe, lucigenin, reacts with AAs under basic conditions, and is therefore unsuitable for this purpose.9 Systematic screening of other fluorescent probes revealed that 6-methoxy-N-(3-sulfopropyl)quinolinium (SPQ),13 which has previously been used for chloride transport studies in vesicles and cells, is strongly quenched by deprotonated AAs (Fig. S3.3.1†). Interestingly, the fluorescence of SPQ is almost indifferent to the neutral, zwitterionic forms of AAs, with the exception of methionine, cysteine, and tryptophan (Fig. S3.4.1†). This suggests that the free –NH2 groups of AAs as well as –SH, –SCH3, and indole moieties are responsible for the efficient fluorescence quenching.§ Separate titration with NaOH revealed (Fig. 4a) that SPQ is only slightly quenched by hydroxide ions – less than 10% of the initial emission at pH 10.7, the highest pH used in our AA transport studies. It is, therefore, a suitable probe for real-time monitoring of the transport of deprotonated AAs in liposomal assays.
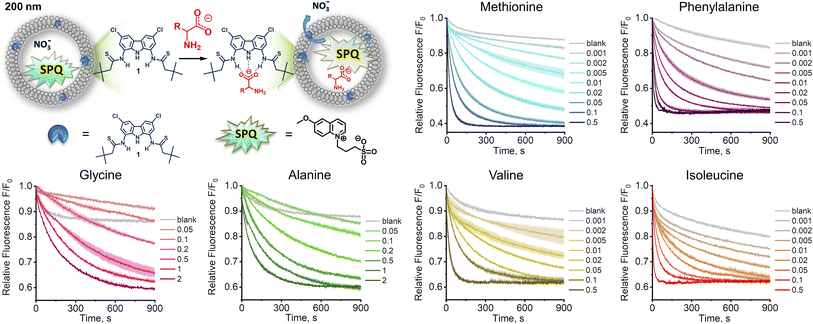 |
| Fig. 3 The kinetics of transport of deprotonated amino acids measured by SPQ assay. Sodium salts of amino acids were added to a final concentration of 25 mM to the suspension of LUVs (POPC : cholesterol 7 : 3 + 0–2 mol% 1) encapsulating SPQ (1 mM) and NaNO3 (225 mM) in aqueous NaNO3 (225 mM). λex = 344 nm, λem = 440 nm. Deviations are shown as shaded areas. | |
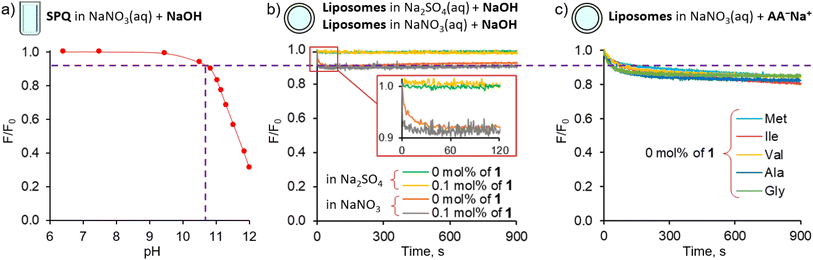 |
| Fig. 4 Effect of pH on SPQ fluorescence in solution and in LUVs: (a) effect of pH on the fluorescence of aqueous solution of SPQ (10−5 M in 225 mM NaNO3); (b) fluorescence changes of SPQ encapsulated in POPC/cholesterol LUVs, having 0 or 0.1 mol% of the transporter 1 in their membrane, upon basification to pH 10.7 by a pulse of NaOH; (c) fluorescence changes of SPQ encapsulated in POPC/cholesterol LUVs, having 0 mol% of the transporter 1 in their membrane, upon addition of sodium salts of various amino acids. | |
In a typical experiment involving SPQ, LUVs (mean diameter 200 nm) were grown from a lipid mixture containing POPC/cholesterol 7
:
3 and transporter 1 (0.1–1 mol%) in the presence of SPQ (1 mM) and NaNO3 (225 mM). Unencapsulated SPQ was separated from the vesicles by gel filtration. Liposomes were then transferred to a spectrofluorometer and a solution of a sodium salt of AA (>95% of the anionic form) was added to obtain final concentration of 25 mM (final pH ≈ 10.7). The addition of AA salts resulted in an immediate drop of fluorescence intensity due to quenching of the residual unencapsulated SPQ (removed in data processing), followed by a gradual decrease of fluorescence due to the transport-mediated quenching of the internal SPQ by deprotonated AAs.
In accord with our hypothesis, the transport of AAs under basic conditions was much faster than at physiological pH. When we used the same amount of transporter as before (1 mol% with respect to lipids), the kinetics was too fast to be measured reliably. After lowering the membrane concentration of 1 to 0.5%, the transport of the most lipophilic AAs, Phe, Ile, Met, and Val, was completed in less than 100 s, while the transport of the more hydrophilic Gly and Ala did not reach plateau within 900 s (Fig. 3).
To quantify the transport rates of the AAs, we fitted the experimental fluorescence decay curves with exponential functions and compared their half-time values (see ESI† for details). This led to the following selectivity order: Phe (t1/2 = 22 s) > Ile (t1/2 = 42 s) > Met (t1/2 = 92 s) > Val (t1/2 = 112 s) ≫ Ala, Gly. The same selectivity order was obtained by measuring EC50 values at 270 s: Phe (0.0082 mol%) > Ile (0.014 mol%) > Met (0.041 mol%) > Val (0.057 mol%) > Ala (0.57 mol%) > Gly (0.79 mol%). Interestingly, the selectivity order measured at pH 10.7 for fully deprotonated amino acids parallels the selectivity trend obtained at pH 7.4 using the calcein–Cu2+ assay. This is in accord with our hypothesis that under physiological pH AAs are also transported in their deprotonated forms.
Control experiments using vesicles with no transporter embedded in the membrane revealed only a slight decrease of fluorescence intensity (<15%) during the first 200 s, followed by very slow non-facilitated diffusion in the case of the more lipophilic AAs (Val, Ile, Met, Phe). This initial drop of SPQ fluorescence was found to be caused by pH equilibration: a similar response was observed upon addition of NaOH to the suspension of vesicles instead of AA salts (Fig. 4b). A separate experiment with the pH sensitive probe, HPTS, confirms that the pH equilibration is complete within few minutes in the NaNO3 environment, even if there is no transporter in the membrane (ESI, Section 5†). We conclude therefore, that the non-facilitated diffusion of AAs anions is negligibly slow in the absence of transporters in the membrane.
The HPTS experiments also revealed that in the NaNO3 environment 1 equalizes the pH on both sides of the membrane almost immediately (within the first 1–2 s) after the addition of base (ESI,† Figure S5.2.1). Thus, in AA− transport experiments this pH equilibration contributes to the vertical drop of fluorescence typically observed in fluorescent assays immediately after the addition of anions and is subtracted from the kinetic data in the course of standard data processing. In contrast, in the sulfate environment the pH gradient persists even in the presence of the transporter (Fig. 4b, see also ESI†). We presume therefore that the pH equilibration takes place via the net OH−/NO3− antiport or H+/NO3− symport, mediated either by carrier deprotonation or by the flip-flop of fatty acid impurities present in the lipid membrane.14
Mechanism of amino acids transport at basic pH
Important insights into the mechanism of AA− transport by 1 was obtained from another control experiment, in which lipophilic NO3− was replaced by hydrophilic SO42− in both the internal and external medium (Fig. 5). No significant transport was detected under these conditions (Fig. 5b), due to the inability of the extremely hydrophilic sulfates to pass through the lipid bilayer and balance the charge of the incoming AA anions. This observation rules out any significant contribution from the symport of AA− with H+ or Na+ and from the antiport of AA− with OH− under these conditions, leaving the AA−/NO3− exchange as the only plausible mechanism of AA transport at high pH.
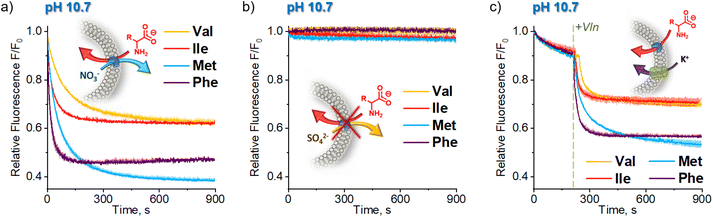 |
| Fig. 5 Comparison of deprotonated AA (AA−) transport kinetics in (a) sodium nitrate; (b) sodium sulfate and (c) potassium sulfate medium. Sodium salts of AAs were added to the suspension of LUVs (POPC : cholesterol 7 : 3 + 0.1 mol% 1) encapsulating SPQ (1 mM, λex = 344 nm, λem = 440 nm) and NaNO3 (225 mM), Na2SO4 (225 mM) or K2SO4 (225 mM) in aqueous NaNO3 (225 mM), Na2SO4 (225 mM) or K2SO4 (225 mM), respectively. Deviations are shown as shaded areas. | |
The transport of AA− in the sulfate medium can be switched ON by enabling any effective mechanism of charge compensation. For example, the addition of potent K+ uniporter, valinomycin, initiates fast AA− transport in vesicles containing 1 in their membrane, suspended in aqueous K2SO4 (Fig. 5c).
Insights into the transport mechanism from pH dependent transport experiments
At first sight, the results obtained in the sulfate medium at pH 10.7 (SPQ assay) are difficult to reconcile with the results obtained in the same medium at pH 7.4 (Cu2+–calcein assay). How is it possible that swift transport is observed at pH 7.4, where the mole fraction of AA− is below 3%, yet no transport can be detected at pH 10.7, where the mole fraction of AA− is ca. 30 times higher? To shed some light on this issue we studied the pH dependence of the AA transport kinetics in both sulfate and nitrate medium.
Unfortunately, neither the Cu2+–calcein nor the SPQ assay is suitable for transport studies at intermediate pH values. In most cases, zwitterionic AAs do not quench SPQ fluorescence and hence the SPQ assay only detects the deprotonated fraction of AAs. However, methionine is unique in that it quenches the SPQ fluorescence in both its anionic and zwitterionic forms to a similar extent (Fig. S3.4.2†). This allows transport measurements at various pH to be compared under otherwise identical conditions.
At pH 5.8, in which methionine exists solely (>99.9%) in its charge neutral, zwitterionic form, only slow, non-facilitated diffusion was observed in the presence of 1, meaning that the receptor is not able to transport methionine in its zwitterionic form (Fig. 6). However, gradual deprotonation of methionine at higher pHs greatly accelerates the facilitated transport, until saturation at pH ca. 10.7, where virtually all methionine is deprotonated. This strongly suggests that 1 transports only the anionic form of the AA.
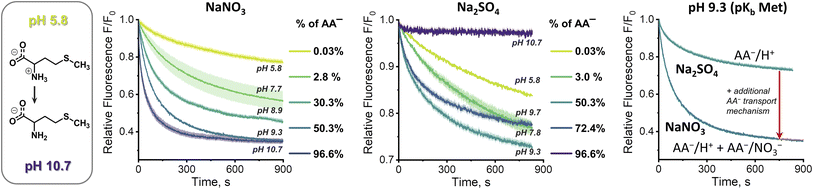 |
| Fig. 6 Methionine transport kinetics measured by the SPQ assay. Methionine solutions at different pH were added to a final concentration of 25 mM to the suspension of LUVs (POPC : cholesterol 7 : 3 + 0.1 mol% 1) encapsulating SPQ (1 mM) and NaNO3 (225 mM). λex = 344 nm, λem = 440 nm. Deviations are shown as shaded areas. | |
Analogous measurements were repeated in the sulfate environment, in which the only plausible mechanism of AA− transport is symport with H+ or antiport with OH− (Fig. 6). At pH 5.8, no difference was noted between the results obtained with and without the transporter, as previously. However, at pH 7.8 the facilitated transport of methionine was already clearly visible. Further basification increased the transport rate, but, unlike in the nitrate medium, it reached maximum at pH 9.3. Increase of pH beyond this point decreased the transport rate, until it stopped completely at pH 10.7. Apparently, although the concentration of AA− increases with rising pH, the co-transport of H+ (or OH−) against the pH gradient slows down and, in the absence of other mechanisms of membrane depolarization, this eventually halts the transport completely.
This peculiar pH dependence of the AA transport rate explains the apparent contradiction highlighted above. In fact, when the methionine transport was measured under comparable conditions (pH 7.4, sulfate medium) by both the Cu2+–calcein and the SPQ assays, the results were almost identical (Fig. S4.11.2b†).
13C NMR assay
To afford additional, more direct evidence for amino acid transport by 1, a 13C NMR assay was used.6g Isotopically enriched glycine, alanine or methionine was added to the suspension of LUVs (mean diameter 1 μm), made of POPC and cholesterol 7
:
3, followed by MeOH solution of 1 (or MeOH alone as control) to initiate the AA* influx (Fig. 7). After 1 h, an aqueous solution of paramagnetic Mn2+ was added to selectively broaden the 13C NMR signals of extravesicular AA* and 13C NMR spectrum of the vesicle suspension was measured. For liposomes without transporter, slow diffusion of methionine was observed, as reflected by a small signal from the intravesicular 13C(1)-L-Met, resonating at δ = 174.3 ppm. For the liposomes containing 1 mol% of 1, a significantly enhanced 13C(1)-L-Met signal was observed after the addition of Mn2+ (Fig. 7d). In case of 13C(1)-Gly and 13C(1)-Ala, small signals of intravesicular AAs were only observed in the presence of 1, in accord with the results from fluorescence assays (see Fig. 7d for Ala and ESI,† Section 6.2, for Gly). Similar experiments were also conducted with Eu3+ instead of Mn2+, which shifts the signal of extravesicular *AAs (ESI, Section 6.2†).
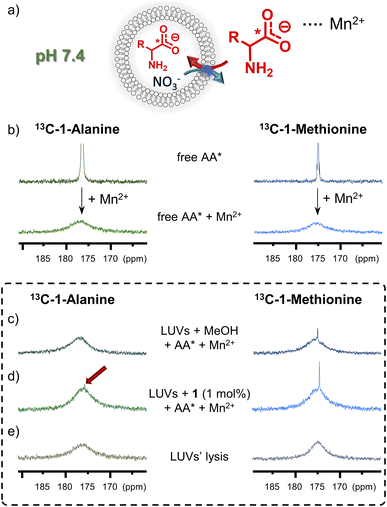 |
| Fig. 7 (a) Schematic representation of the 13C NMR assay for AA* transport. (b) 13C NMR spectra of AA* in 9 : 1 H2O/D2O before and after the addition of MnSO4. Box: 13C NMR spectra of AA* mixed with POPC/cholesterol 7 : 3 LUVs in 9 : 1 (v/v) H2O/D2O 60 min after the addition of (c) MeOH (blank) or (d) MeOH solution of 1 (1 mol%), upon addition of MnSO4, (e) spectra recorded after addition of Triton-X. | |
Amino acid transport by model urea, thiourea and squaramide receptors
The discovery of efficient AA transport by simple monotopic receptor 1 encouraged us to investigate other monotopic anion receptors as potential AA carriers. Four representative urea, thiourea and squaramide receptors 3–6 were selected from the literature based on their structural simplicity, carboxylate affinity and outstanding anionophoric activity (Fig. 8). Their AA transport ability was investigated using the SPQ assay, with sodium salt of methionine as a model AA.
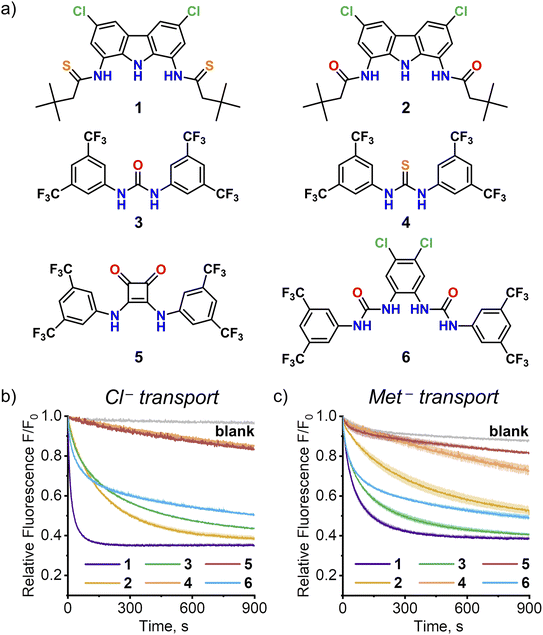 |
| Fig. 8 (a) Structures of synthetic anion transporters selected for comparison with 1. The kinetics of transport of (b) Cl− (25 mM) or c) Met− (25 mM) by transporters 1–6 (0.1 mol%) measured using SPQ assay at pH 10.6 in aqueous NaNO3. Deviations are shown as shaded areas. | |
As shown in Fig. 8, two of these four receptors, urea 3
15 and bis(urea) 6
16, turned out to be efficient AA transporters. Squaramide 5
17 displayed no activity, in accord with the previous studies by Gale and co-workers.8 Surprisingly, thiourea 4 exhibited much lower activity than its urea analog 3, unlike in the chloride/nitrate exchange studied previously.15a Additional experiments revealed, that this is due to deprotonation of 4 at the basic pH employed in this experiment (compare the transport of Met− and Cl− at pH 10.6 in Fig. 8; see Section 4.12 of ESI† for details).
Although none of the four receptors 3–6 was as active as 1, the results clearly show that high AA transport ability of 1 is not unique among monotopic anion carriers. Systematic screening of such receptors using the versatile SPQ assay developed in this work is likely to identify even more potent AA transporters. It is also worth highlighting that the ability of anionophores to transport AAs across lipophilic membranes might be important for their biological activity.
Conclusions
In conclusion, we have shown for the first time that simple monotopic anion receptors can efficiently transport AAs across lipid bilayers at physiological pH. In particular, carbazole-based dithioamide 1 was shown to facilitate rapid diffusion of various proteinogenic AAs, including the most hydrophilic ones, across liposomal membranes, despite being unable to bind their ammonium groups. This unexpected performance was rationalised by in-depth mechanistic studies which showed that 1 transports AAs in their deprotonated, anionic form, even though at neutral pH this form consist of less than 3% of the total AA concentration. This demonstrates that the high activity of small molecule anionophores can make up for the unfavourable speciation of AAs at physiological pH, leading to the remarkable overall transport rates. Accordingly, screening of four representative chloride carriers selected from literature revealed that two of them are also active AA transporters. Systematic screening of other known anionophores using versatile SPQ assay developed in this work is likely to yield more of potent AA transporters as well as valuable information pertaining to their biological activity.
Based on these findings we propose a new strategy for the development of artificial AA transporters with improved activity. Traditionally, complex heteroditopic receptors have been designed to shield both carboxylate and ammonium AAs termini. Our approach relies on screening comparatively simple monotopic receptors. In addition to easier synthesis, their inherent advantage is smaller size, which facilitates diffusion across the lipid membrane. It is noteworthy that these two classes of transporters should, in principle, show opposite pH dependence: while the transport rate by monotopic carboxylate receptors should be proportional to the concentration of deprotonated AA, i.e. increase with pH, the opposite might be expected from heteroditopic receptors, which bind zwitterionic forms of AAs. Given that different tissues, cells and organelles have different pH values and pH gradients, the choice of particular strategy may depend on targeted biological application. We believe that the findings described in this paper will significantly facilitate the search for small, drug-like AA transporters with interesting biological activity.
Data availability
Data supporting this research work are available in the manuscript and in the ESI.†
Author contributions
K. M.-J. contributed to the conceptualization, carried out the synthesis of transporters 1 and 2, performed SPQ quenching and AAs transport experiments, collected and analysed data, and contributed to manuscript writing, illustrating and editing. K. M. B. contributed to the conceptualization and discussion, manuscript writing and editing. B. Z. synthesised receptors 3–6 and performed some spectroscopic studies. M. J. C. contributed to the conceptualization, discussion, funding acquisition, supervision, and manuscript writing and editing.
Conflicts of interest
There are no conflicts to declare.
Acknowledgements
This work was supported by the National Science Centre, Poland (OPUS grant 2018/31/B/ST5/02085 to M. J. C.) This study was carried out at the Biological and Chemical Research Centre, University of Warsaw, established within a project co-financed by the European Union through the European Regional Development Fund under the Operational Programme Innovative Economy 2007–2013.
Notes and references
-
(a) M. H. Saier Jr, Microbiology, 2000, 146, 1775–1795 CrossRef PubMed;
(b) W. Saurin, M. Hofnung and E. Dassa, J. Mol. Evol., 1999, 48, 22–41 CrossRef CAS.
- P. Kandasamy, G. Gyimesi, Y. Kanai and M. A. Hediger, Trends Biochem. Sci., 2018, 43, 752–789 CrossRef CAS.
- Many drug molecules contain both COOH and NH2 groups in their structure; these include anti-cancer drugs Melphalan, Methotrexate and Pemetrexed. All these molecules are zwitterionic at physiological conditions and need transporters to effectively pass through biological membranes..
- Y. Cha, E.-S. Kim and J. Koo, Int. J. Mol. Sci., 2018, 19, 907–924 CrossRef.
-
(a) P. D. Dobson and D. B. Kell, Nat. Rev. Drug Discovery, 2008, 7, 205–220 CrossRef CAS PubMed;
(b) I. Alfonso and R. Quesada, Chem. Sci., 2013, 4, 3009–3019 RSC;
(c) A. I. Share, K. Patel, C. Nativi, E. J. Cho, O. Francesconi, N. Busschaert, P. A. Gale, S. Roelens and J. L. Sessler, Chem. Commun., 2016, 52, 7560–7563 RSC;
(d) S.-H. Park, S.-H. Park, E. N. W. Howe, J. Y. Hyun, L.-J. Chen, I. Huang, G. Vargas-Zuñiga, N. Busschaert, P. A. Gale, J. L. Sessler and I. Shin, Chem, 2019, 5, 2079–2098 CrossRef CAS PubMed.
-
(a) L. E. Bickerton, T. G. Johnson, A. Kerckhoffs and M. J. Langton, Chem. Sci., 2021, 12, 11252–11274 RSC;
(b) W. Stillwell, Biosystems, 1976, 8, 111–117 CrossRef CAS;
(c) J. Sunamoto, K. Iwamoto, Y. Mohri and T. Kominato, J. Am. Chem. Soc., 1982, 104, 5502–5504 CrossRef CAS;
(d) J. Sánchez-Quesada, H. Sun Kim and M. R. Ghadiri, Angew. Chem., Int. Ed., 2001, 40, 2503–2506 CrossRef;
(e) L. Chen, W. Si, L. Zhang, G. Tang, Z.-T. Li and J.-L. Hou, J. Am. Chem. Soc., 2013, 135, 2152–2155 CrossRef CAS PubMed;
(f) Y. Li, J. Dong, W. Gong, X. Tang, Y. Liu, Y. Cui and Y. Liu, J. Am. Chem. Soc., 2021, 143, 20939–20951 CrossRef CAS;
(g) L. Martinez-Crespo, J. Liang Sun-Wang, A. Felipe Sierra, G. Aragay, E. Errasti-Murugarren, P. Bartoccioni, M. Palacin and P. Ballester, Chem, 2020, 6, 3054–3070 CrossRef.
-
(a) F. de Riccardis, I. Izzo, D. Montesarchio and P. Tecilla, Acc. Chem. Res., 2013, 46, 2781–2790 CrossRef PubMed;
(b) P. A. Gale, R. Perez-Tomas and R. Quesada, Acc. Chem. Res., 2013, 46, 2801–2813 CrossRef PubMed;
(c) P. A. Gale, J. T. Davis and R. Quesada, Chem. Soc. Rev., 2017, 46, 2497–2519 RSC;
(d) X. Wu, E. N. W. Howe and P. A. Gale, Acc. Chem. Res., 2018, 51, 1870–1879 CrossRef PubMed;
(e) X. Wu, A. M. Gilchrist and P. A. Gale, Chem, 2020, 6, 1296–1309 CrossRef;
(f) H. Valkenier, L. W. Judd, H. Li, S. Hussain, D. N. Sheppard and A. P. Davis, J. Am. Chem. Soc., 2014, 136, 12507–12512 CrossRef.
- X. Wu, N. Busschaert, N. J. Wells, Y.-B. Jiang and P. A. Gale, J. Am. Chem. Soc., 2015, 137, 1476–1484 CrossRef CAS PubMed.
-
(a) K. M. Bąk, B. van Kolck, K. Maslowska-Jarzyna, P. Papadopoulou, A. Kros and M. J. Chmielewski, Chem. Commun., 2020, 56, 4910–4913 RSC;
(b) R. Pomorski, M. García-Valverde, R. Quesada and M. J. Chmielewski, RSC Adv., 2021, 11, 12249–12253 RSC.
- K. M. Bąk, K. Chabuda, H. Montes, R. Quesada and M. J. Chmielewski, Org. Biomol. Chem., 2018, 16, 5188–5196 RSC.
-
(a) A. C. Chakrabarti and D. W. Deamer, Biochim. Biophys. Acta, 1992, 1111, 171–177 CrossRef CAS;
(b) A. C. Chakrabarti, Amino Acids, 1994, 6, 213–229 CrossRef CAS.
-
(a) P. Breccia, M. Van Gool, R. Pérez-Fernández, S. Martín-Santamaría, F. Gago, P. Prados and J. de Mendoza, J. Am. Chem. Soc., 2003, 125, 8270–8284 CrossRef CAS;
(b) J. L. Sessler and A. Andrievsky, Chem. - Eur. J., 1998, 4, 159–167 CrossRef;
(c) M. T. Reetz, J. Huff, J. Rudolph, K. Toellner, A. Deege and R. Goddard, J. Am. Chem. Soc., 1994, 116, 11588–11589 CrossRef;
(d) J. Rebek, B. Askew, D. Nemeth and K. Parris, J. Am. Chem. Soc., 1987, 109, 2432–2434 CrossRef.
- A. S. Verkman, Am. J. Physiol., 1990, 259, C375–C388 CrossRef.
- X. Wu and P. A. Gale, J. Am. Chem. Soc., 2016, 138, 16508–16514 CrossRef PubMed.
-
(a) N. Busschaert, I. L. Kirby, S. Young, S. J. Coles, P. N. Horton, M. E. Light and P. A. Gale, Angew. Chem., Int. Ed., 2012, 51, 4426–4430 CrossRef;
(b) X. Wu, L. W. Judd, E. N. W. Howe, A. M. Withecombe, V. Soto-Cerrato, H. Li, N. Busschaert, H. Valkenier, R. Pérez-Tomás, D. N. Sheppard, Y.-B. Jiang, A. P. Davis and P. A. Gale, Chem, 2016, 1, 127–146 CrossRef.
- C. M. Dias, H. Li, H. Valkenier, L. E. Karagiannidis, P. A. Gale, D. N. Sheppard and A. P. Davis, Org. Biomol. Chem., 2018, 16, 1083–1087 RSC.
-
(a) A. Rostami, A. Colin, X. Y. Li, M. G. Chudzinski, A. J. Lough and M. S. Taylor, J. Org. Chem., 2010, 75, 3983–3992 CrossRef PubMed;
(b) N. Busschaert, R. B. P. Elmes, D. D. Czech, X. Wu, I. L. Kirby, E. M. Peck, K. D. Hendzel, S. K. Shaw, B. Chan, B. D. Smith, K. A. Jolliffe and P. A. Gale, Chem. Sci., 2014, 5, 3617–3626 RSC.
-
(a) S. Jayaraman and A. S. Verkman, Biophys. Chem., 2000, 85, 49–57 CrossRef;
(b) S. Jayaraman, J. Biwersi and A. S. Verkman, Am. J. Physiol., 1999, 276, C747–C757 CrossRef PubMed.
Footnotes |
† Electronic supplementary information (ESI) available: Experimental details, data fitting, additional discussion and supplementary figures. See DOI: https://doi.org/10.1039/d2sc04346g |
‡ As will be shown later, the complete lack of activity in case of diamide 2 is not due to inefficient post-incorporation into the lipid bilayer, but rather due to the combination of intrinsically low activity and very low concentration of deprotonated amino acids at pH 7.4, which together slow down the transport below the detection limit of the calcein assay (see Fig. 8 and the accompanying discussion; see also chapters 4.4 and 4.11 of the ESI†). |
§ The quenching of SPQ emission is mediated through a non-emissive excited charge transfer complex and subsequent electron transfer.18 As a result, quenching efficiencies correlate well with redox properties of the quencher. Amino groups, thiols, organic sulphides, and indole are known electron donors. |
|
This journal is © The Royal Society of Chemistry 2022 |
Click here to see how this site uses Cookies. View our privacy policy here.