DOI:
10.1039/D2SC04350E
(Edge Article)
Chem. Sci., 2022,
13, 11405-11410
Access to dialkylated allylic stereogenic centers by Ni-catalysed enantioselective hydrovinylation of unactivated alkenes†
Received
5th August 2022
, Accepted 12th September 2022
First published on 13th September 2022
Abstract
Tertiary dialkylated allylic stereogenic centers are widespread substructures in bioactive molecules and natural products. However, enantioselective access to dialkyl substituted allylic motifs remains a long-term challenge. Herein, a straightforward protocol to build allylic dialkylated stereogenic centers enabled by nickel-catalysed regio- and enantioselective hydrovinylation of isolated unactivated alkenes facilitated by a weakly coordinating group with vinyl bromides was developed, affording dialkylated allylic species in good yields with excellent enantioselectivities. The reaction distinguishes distinct alkenes and works for both terminal and internal aliphatic alkenes. The reaction proceeds under mild conditions and tolerates a wide range of functional groups.
Introduction
Tertiary allylic carbons bearing two alkyl substituents are widely found in natural products and other bioactive molecules.1 Traditional methods to access substituted allylic carbon centers heavily rely on transition-metal-catalysed asymmetric allylic alkylation (AAA) reactions. However, it remains challenging to access dialkyl substituted allylic tertiary carbon centers due to the difficulties in differentiating two alkyl groups similar in steric and electronic properties. Over the past few decades, transition-metal catalysed asymmetric cross-coupling enabled C–C bond-forming processes have proved to be a powerful tool to construct saturated stereogenic carbon centers.2 In particular, transition-metal catalysed asymmetric cross-couplings of vinyl electrophiles with alkyl electrophiles are a straightforward and complementary way to build allylic stereogenic carbon centers. While significant progress has been achieved regarding transition-metal-catalysed asymmetric synthesis of the Csp2–Csp3 bond, the available methods only allow access to only allylic structures next to aryl,3 nitrile,4 amides,5 or esters.6 No successful examples are known to construct dialkylated allylic motifs (Scheme 1a). Recently, alkenes have been employed as surrogates for alkyl nucleophiles in the presence of metal hydride species.7,8 Accordingly, metal-catalysed asymmetric Csp2–Csp3 coupling using alkenes as alkyl nucleophiles with metal hydride via hydrofunctionalizations has been established. However, the use of this strategy to build allylic stereogenic centers is challenging due to the competitive reactivity between alkenes with vinyl electrophiles. In 2021, Buchwald reported the first example of Pd/Cu co-catalysed enantioselective hydrovinylation of styrenes with vinyl triflates to deliver allylic benzylic stereogenic centers (Scheme 1b).9 In the same year, Zhu and Gong developed the Ni-catalysed enantioselective hydrovinylation of styrenes to form the same type of stereogenic carbon center.10 However, enantioselective access to dialkyl substituted allylic stereogenic centers via hydrovinylation of unactivated alkenes remains underexplored partially due to the low reactivity of unactivated alkenes and associated poor selectivity issues. Recently, Giri disclosed a Ni-catalysed regioselective alkenylarylation of γ,δ-alkenyl ketones via carbonyl coordination.11 Engle reported a directed racemic hydroarylation and hydroalkenylation of unactivated alkenes bearing a remote coordination group.12 Herein, we report a general protocol to construct tertiary allylic stereogenic centers with two alkyl substituents enabled by Ni-catalysed regio- and enantioselective hydrovinylation of unactivated aliphatic alkenes with vinyl halides (Scheme 1c). The strategy allows for the chemoselective hydrofunctionalizations between two alkenes facilitated by a weak coordinating group to furnish substituted tertiary carbon centers with good functional group tolerance.
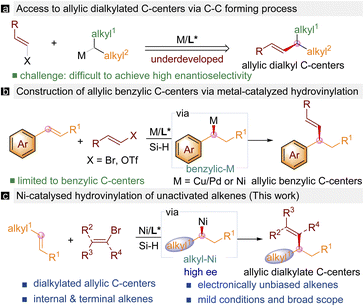 |
| Scheme 1 Impetus for the development of dialkylated allylic stereogenic centers. | |
Results and discussion
We started to test the feasibility of the transformation using internal aliphatic alkene 1a and (E)-1-(2-bromovinyl)-4-methoxybenzene 2a as the prototype substrates (Table 1). After evaluation of a wide range of reaction parameters, the reaction using Ni(COD)2 (10 mol%) as a precatalyst, the bisoxazolidine-based ligand (L1, 12 mol%) as an anchoring ligand, (MeO)3SiH (3.0 equiv.) as a hydride source, potassium phosphate monohydrate (2 equiv.) as a base in the mixture of dioxane and DME (3
:
1, 0.05 M) at 0 °C delivered the desired product 3a with optimal results (79% isolated yield and 92% ee, rr = 10
:
1, Table 1, entry 1).13
Table 1 Condition optimization of Ni-catalysed hydrovinylation of unactivated alkenes
Other bidentate chiral ligands could catalyse the desired transformation, albeit in lower yields and levels of enantioselectivity (Table 1, entries 2–4). Replacing vinyl bromides with vinyl chlorides or iodides dramatically decreases the efficiency of the hydrovinylation reaction (Table 1, entries 5 and 6). The other nickel source could be used as a precatalyst for the reaction, delivering 3a with good efficiency (Table 1, entries 7–9). The use of dioxane or dimethyl ether (DME) as the sole solvent substantially diminishes the yield and enantioselectivity of the product (Table 1, entries 10 and 11). Inorganic bases, such as cesium carbonate, potassium carbonate, and sodium carbonate, could mediate the reaction to give 3a in 30–55% yields with comparable enantioselectivity (Table 1, entries 12–14). Carrying out the reaction at room temperature delivered the hydrovinylation product 3a in 85% yield with 83% ee (Table 1, entry 15). In addition, decreasing the stoichiometry of 2a diminished the efficiency of the reaction (Table 1, entry 16).
With the optimized conditions in hand, we turned to investigate the scope of this protocol to construct dialkylated allylic stereogenic centers (Table 2). First, the scope of the unactivated aliphatic alkenes was tested. Various 1-monosubstituted and 1,2-disubstituted aliphatic alkenes with a range of functional groups could be coupled with vinyl bromides to afford corresponding formal allylic alkylated products (3a–3o) in good to excellent yields with good levels of regio- and enantioselectivity. Electron-withdrawing and electron-donating group substituted aniline-derived amides were well-tolerated in the reaction, delivering dialkylated allylic stereogenic centers in 71–79% yields with 90–92% ee (3a–3d). Notably, ketone could be tolerated under the reductive conditions, delivering 3b in 71% yield with 90% ee. Heteroaromatic amine-based alkene was transformed into 3e in 70% yield with 92% ee. E-internal alkenes with different functional groups, such as aryls and esters, are compatible in the reaction (3f–3j), giving the desired products in 54–78% yields with 88–91% ee. Notably, Z-internal alkenes are also compatible in this reaction to furnish 3h in 55% yield with 88% ee. Terminal aliphatic alkenes are also good substrates for this reaction (3k and 3l). Interestingly, allylic branched alkene undergoes the desired transformation to afford dialkylated allylic product 3l bearing two continuous saturated stereogenic centers in 72% yield (dr = 1.05
:
1) with 89% and 87% ee. Both terminal and internal allylic N-acyl amines could be involved in the reaction, giving β-vinyl chiral amines (3m–3o) in 60–78% yields with 85–92% ee.
For standard conditions, see Table 1. rr = ratio of β and γ selective product. rr was determined by GC. Enantiomeric excess was determined by chiral HPLC analysis using a chiral stationary phase.
NiBr2·glyme (10 mol%), NaI (1.0 equiv.), and dioxane (0.05 M) were used.
1 : 2 = 2.5 : 1, NiBr2·glyme (10 mol%), NaI (0.2 equiv.), and dioxane (0.05 M) were used.
1 : 2 = 2 : 1, NiBr2·glyme (10 mol%), NaI (0.5 equiv.), KCl (0.5 equiv.), CsF (2.0 equiv.), HBpin (3.0 equiv.), and dioxane (0.05 M) were used.
|
|
Next, the scope of vinyl bromides was examined. E-styryl bromides are well-tolerated in the reaction, affording corresponding E-styryl dialkylated compounds (4a–4r) in good yields with good levels of regio- and enantioselectivity. Styrene derivatives with para-substitution patterns are all well compatible under the reaction conditions. Electron-donating group substituted aryl vinyl bromides were transformed into 4b–4e in 50–75% yields with 86–97% ee. Meanwhile, electron-withdrawing group substituted aryl vinyl bromides were successfully involved in the reaction to give the desired products (4f–4h) in 55–60% yields with 85–88% ee. meta- and ortho-substituted aryl vinyl bromides with electron-donating and electron-withdrawing groups underwent the regio- and enantioselective hydrovinylation reaction to give 4i–4l in 52–70% yields with 89–93% ee. It is noteworthy that chloro- (4f), -Bpin (4g), and bromo- (4j) were compatible under the reaction conditions, leaving chemical handles for further elaborations.
Moreover, multi-substituted styryl bromides could be converted to desired products (4m–4o) in 74–80% yields with 85–87% ee. Heteroaryl vinyl bromides, including carbazole, thiophene, and pyridine, were converted to 4p–4r in 56–65% yields with 89–91% ee. The absolute configuration of the products is confirmed to be S unambiguously by X-ray diffraction analysis of 4q. Moreover, Z-alkenyl bromide could be involved in the reaction, delivering the desired product 4s in 51% yield with 93% ee. Impressively, 1,1-disubstituted and 1,1,2-trisubstituted vinyl bromides proved successful in the reaction, giving sterically demanding dialkylated allylic products (4t and 4u) in synthetic useful yields with 93–94% ee, which are inaccessible otherwise. In addition, aliphatic vinyl bromides could be used in the reaction to form desired product 4v in 42% yield with 87% ee.
Next, the reaction proceeded smoothly on the 2.0 mmol scale under standard conditions, giving 3a in 73% yield with 90% ee, which showcased the potential of this protocol for the application of large-scale synthesis (Scheme 2a). To probe the reaction mechanism of this reaction, a series of control experiments were conducted. First, the reaction of 1-substituted aliphatic alkene 1k with 2a was carried out at room temperature using a deuterated silane (Ph2SiD2). Desired product 5 was obtained in 79% yield based on the conversion of 1k with 80% ee (Scheme 2b). Under identical conditions, the reaction of 1,2-disubstituted aliphatic alkene 1a with 2a delivered the desired product 6 based on the conversion of 1a with 90% ee (Scheme 2c). In both cases, deuterium was exclusively delivered to γ-position of the amide group, indicating that the hydrometalation of Ni–H with alkenes is irreversible or the following Csp3–Csp2 bond-forming step is much faster. Furthermore, the reaction of corresponding α,β-unsaturated amide 1a′ successfully led to desired hydrovinylation product 3k in 35% yield with opposite absolute configuration (80% ee), excluding the isomerized alkene 1a′ as an intermediate for the reaction (Scheme 3d).
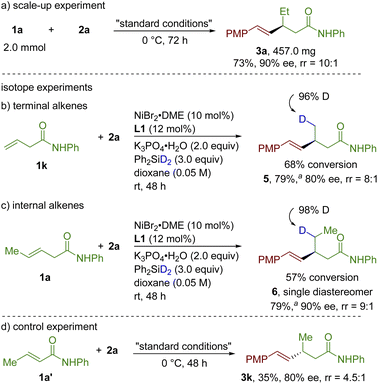 |
| Scheme 2 Scale-up experiment and mechanistic experiments. a Yield was calculated based on the recovery of alkenes. | |
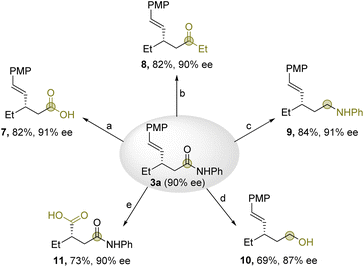 |
| Scheme 3 Synthetic applications of 3a. Reaction conditions: (a) (1) (Boc)2O, Et3N, DMAP, DCM, and 0 °C to room temperature; (2) LiOH, H2O2, THF/H2O = 3 : 1, and 0 °C to room temperature. (b) (1) Tf2O, 2-F-pyridine, DCM, and −78 °C to 0 °C; (2) EtMgBr and 0 °C. (c) LAH, THF, and 0 °C to 85 °C. (d) (1) NaH, BnBr, DMF, and 0 °C to room temperature; (2) LAH, THF, and 0 °C to 85 °C. (e) RuCl3, NaIO4, CCl4/CH3CN = 1 : 1, and rt. | |
Furthermore, transformations of the allylic dialkylated products were examined using 3a to further demonstrate the synthetic potential of this reaction (Scheme 3). First, the amide moiety could be transformed into diverse functional groups (7–10) without erasing the levels of enantioselectivity. Accordingly, β-vinyl acids and ketones as well as γ-vinyl amines and alcohols were obtained in good yields (69–84%) with good levels of enantioselectivity (87–91%). Moreover, α-alkylated carboxylic acid bearing acidic α-proton (11) was obtained in 73% yield with 90% ee by C
C cleavage.
Finally, a plausible mechanism for this reaction is proposed in Scheme 4.8–10 First, Ni(I)–H(A) was formed from the reaction of Ni(I)–X with silane with the assistance of the base. A coordinated with first alkenes 1 to undergo regioselective migratory insertion to generate secondary alkyl nickel species B, which could undergo oxidative addition with vinyl bromides 2 to form intermediate C. The final dialkylated allylic product was obtained via reductive elimination of C along the formation of Ni(I) to finish the catalytic cycle.
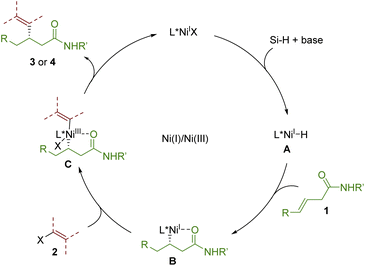 |
| Scheme 4 Proposed mechanism for the reaction. | |
Conclusions
In summary, a straightforward protocol to access dialkylated allylic stereogenic centers has been developed by nickel catalysed enantioselective hydrovinylation of unactivated aliphatic alkenes with vinyl bromides facilitated by a directing group. The reaction proceeded under mild conditions with great chemo- and regioselectivities over two distinct alkenes to afford formal allylic dialkylated products with excellent levels of enantioselectivity for the first time. The reaction tolerates diverse substitution patterns and a wide range of functional groups.
Data availability
Experimental data have been provided in the ESI.†
Author contributions
J. X. Z. discovered the project. W. S. conceived and directed the project. J. X. Z. and P. F. Y. carried out the experiments and collected the data. WS wrote the manuscript with contributions from all authors.
Conflicts of interest
There are no conflicts to declare.
Acknowledgements
Financial support from the NSFC (22171127 and 21971101), Guangdong Basic and Applied Basic Research Foundation (2022A1515011806), Department of Education of Guangdong Province (2021KTSCX106 and 2022JGXM054), The Stable Support Plan Program of Shenzhen Natural Science Fund (No. 20200925152608001), Thousand Talents Program for Young Scholars, the Pearl River Talent Recruitment Program (2019QN01Y261), and Guangdong Provincial Key Laboratory of Catalysis (No. 2020B121201002) are sincerely acknowledged. We acknowledge the assistance of SUSTech Core Research Facilities. We thank Dr Xiaoyong Chang (SUSTech) for X-ray crystallographic analysis and Dr Wen-Tao Zhao (SUSTech) for reproducing the results of 3d, 3j, 4f and 4o.
Notes and references
-
(a) R. Sieben, U. Philippi and E. Eich, J. Nat. Prod., 1984, 47, 433–438 CrossRef CAS;
(b) B. M. Trost and M. L. Crawley, Chem. Rev., 2003, 103, 2921–2944 CrossRef CAS PubMed;
(c) S. Berényi, C. Csutorás and A. Sipos, Curr. Med. Chem., 2009, 16, 3215–3242 CrossRef PubMed;
(d) M. A. Versiani, T. Diyabalanage, R. Ratnayake, C. J. Henrich, S. E. Bates, J. B. McMahon and K. R. Gustafson, J. Nat. Prod., 2011, 74, 262–266 CrossRef CAS PubMed;
(e) F. Galli, F. Mazzini, L. Bamonti, L. Gille, S. Böhmdorfer, M. Piroddi, T. Netscher, F. J. Kelly and T. Rosenau, Bioorg. Med. Chem., 2011, 19, 6483–6491 CrossRef CAS PubMed;
(f) Z. Li, J. Cheng, X. Yang, H. Liu, X. Xu, L. Ma, S. Shang and Z. Song, Int. J. Biol. Macromol., 2020, 150, 1–8 CrossRef CAS PubMed;
(g) J. Long, W. Ji, D. Zhang, Y. Zhu and Y. Bi, Front. Pharmacol., 2021, 12 DOI:10.3389/fphar.2021.759220;
(h) P. Yi, B. Li, S. Zafar, S. Ali, W. B. Sheng, Y. Mao, F. Zhou, W. M. Chen, Y. R. Tang, C. Y. Peng, M. I. Choudhary, A. Rahman and W. Wang, Nat. Prod. Res., 2021 DOI:10.1080/14786419.2021.2012669.
-
(a) A. Rudolph and M. Lautens, Angew. Chem., Int. Ed., 2009, 48, 2656–2670 CrossRef CAS PubMed;
(b) F. S. Han, Chem. Soc. Rev., 2013, 42, 5270–5298 RSC;
(c) E. C. Swift and E. R. Jarvo, Tetrahedron, 2013, 69, 5799–5817 CrossRef CAS PubMed;
(d) A. H. Cherney, N. T. Kadunce and S. E. Reisman, Chem. Rev., 2015, 115, 9587–9652 CrossRef CAS;
(e) G. C. Fu, ACS Cent. Sci., 2017, 3, 692–700 CrossRef CAS PubMed;
(f) X. Qi and T. Diao, ACS Catal., 2020, 10, 8542–8556 CrossRef CAS PubMed;
(g) K. E. Poremba, S. E. Dibrell and S. E. Reisman, ACS Catal., 2020, 10, 8237–8246 CrossRef CAS PubMed;
(h) K. Juhász, Á. Magyar and Z. Hell, Synthesis, 2021, 53, 983–1002 CrossRef.
-
(a) A. H. Cherney and S. E. Reisman, J. Am. Chem. Soc., 2014, 136, 14365–14368 CrossRef CAS PubMed;
(b) N. Suzuki, J. L. Hofstra, K. E. Poremba and S. E. Reisman, Org. Lett., 2017, 19, 2150–2153 CrossRef CAS PubMed;
(c) J. L. Hofstra, A. H. Cherney, C. M. Ordner and S. E. Reisman, J. Am. Chem. Soc., 2018, 140, 139–142 CrossRef CAS PubMed;
(d) T. J. Delano and S. E. Reisman, ACS Catal., 2019, 9, 6751–6754 CrossRef CAS PubMed;
(e) Y. Li, Z. Ding, A. Lei and W. Kong, Org. Chem. Front., 2019, 6, 3305–3309 RSC;
(f) Z.-X. Tian, J.-B. Qiao, G.-L. Xu, X. Pang, L. Qi, W.-Y. Ma, Z.-Z. Zhao, J. Duan, Y.-F. Du, P. Su, X.-Y. Liu and X.-Z. Shu, J. Am. Chem. Soc., 2019, 141, 7637–7643 CrossRef CAS PubMed.
- J. Choi and G. C. Fu, J. Am. Chem. Soc., 2012, 134, 9102–9105 CrossRef CAS PubMed.
-
(a) J. Choi, P. Martín-Gago and G. C. Fu, J. Am. Chem. Soc., 2014, 136, 12161–12165 CrossRef CAS;
(b) Z. Wang, Z.-P. Yang and G. C. Fu, Nat. Chem., 2021, 13, 236–242 CrossRef CAS PubMed.
- X. Dai, N. A. Strotman and G. C. Fu, J. Am. Chem. Soc., 2008, 130, 3302–3303 CrossRef CAS PubMed.
- For reviews, see:
(a) J. Chen and Z. Lu, Org. Chem. Front., 2018, 5, 260–272 RSC;
(b) R. Blieck, M. Taillefer and F. Monnier, Chem. Rev., 2020, 120, 13545–13598 CrossRef CAS PubMed;
(c) X.-X. Wang, X. Lu, Y. Li, J.-W. Wang and Y. Fu, Sci. China: Chem., 2020, 63, 1586–1600 CrossRef CAS;
(d) Y. He, J. Chen, X. Jiang and S. Zhu, Chin. J. Chem., 2022, 40, 651–661 CrossRef CAS.
-
(a) X. Lu, B. Xiao, L. Liu and Y. Fu, Chem.–Eur. J., 2016, 22, 11161–11164 CrossRef CAS;
(b) X. Lu, B. Xiao, Z. Zhang, T. Gong, W. Su, J. Yi, Y. Fu and L. Liu, Nat. Commun., 2016, 7, 11129 CrossRef PubMed;
(c) Y. He, Y. Cai and S. Zhu, J. Am. Chem. Soc., 2017, 139, 1061–1064 CrossRef CAS;
(d) F. Zhou, J. Zhu, Y. Zhang and S. Zhu, Angew. Chem., Int. Ed., 2018, 57, 4058–4062 CrossRef CAS PubMed;
(e) Z. Wang, H. Yin and G. C. Fu, Nature, 2018, 563, 379–383 CrossRef CAS PubMed;
(f) J. Xiao, Y. He, F. Ye and S. Zhu, Chem, 2018, 4, 1645–1657 CrossRef CAS;
(g) S. Z. Sun, M. Börjesson, R. Martin-Montero and R. Martin, J. Am. Chem. Soc., 2018, 140, 12765–12769 CrossRef CAS PubMed;
(h) F. Zhou, Y. Zhang, X. Xu and S. Zhu, Angew. Chem., Int. Ed., 2019, 58, 1754–1758 CrossRef CAS PubMed;
(i) S. Bera and X. Hu, Angew. Chem., Int. Ed., 2019, 58, 13854–13859 CrossRef CAS PubMed;
(j) D. Qian and X. Hu, Angew. Chem., Int. Ed., 2019, 58, 18519–18523 CrossRef CAS PubMed;
(k) S. Z. Sun, C. Romano and R. Martin, J. Am. Chem. Soc., 2019, 141, 16197–16201 CrossRef CAS;
(l) Z. P. Yang and G. C. Fu, J. Am. Chem. Soc., 2020, 142, 5870–5875 CrossRef CAS PubMed;
(m) Y. He, C. Liu, L. Yu and S. Zhu, Angew. Chem., Int. Ed., 2020, 59, 21530–21534 CrossRef CAS PubMed;
(n) S.-J. He, J.-W. Wang, Y. Li, Z.-Y. Xu, X.-X. Wang, X. Lu and Y. Fu, J. Am. Chem. Soc., 2020, 142, 214–221 CrossRef CAS PubMed;
(o) X. Jiang, B. Han, Y. Xue, M. Duan, Z. Gui, Y. Wang and S. Zhu, Nat. Commun., 2021, 12, 3792 CrossRef CAS PubMed;
(p) S. Wang, J.-X. Zhang, T.-Y. Zhang, H. Meng, B.-H. Chen and W. Shu, Nat. Commun., 2021, 12, 2771 CrossRef CAS PubMed;
(q) J. Chen and S. Zhu, J. Am. Chem. Soc., 2021, 143, 14089–14096 CrossRef CAS PubMed;
(r) L. Shi, L.-L. Xing, W.-B. Hu and W. Shu, Angew. Chem., Int. Ed., 2021, 60, 1599–1604 CrossRef CAS PubMed;
(s) S. Cuesta-Galisteo, J. Schörgenhumer, X. Wei, E. Merino and C. Nevado, Angew. Chem., Int. Ed., 2021, 60, 1605–1609 CrossRef CAS PubMed;
(t) L. Meng, J. Yang, M. Duan, Y. Wang and S. Zhu, Angew. Chem., Int. Ed., 2021, 60, 23584–23589 CrossRef CAS PubMed;
(u) Y. Zhang, J. Ma, J. Chen, L. Meng, Y. Liang and S. Zhu, Chem, 2021, 7, 3171–3188 CrossRef CAS;
(v) D. Qian, S. Bera and X. Hu, J. Am. Chem. Soc., 2021, 143, 1959–1967 CrossRef CAS PubMed;
(w) S. Bera, R. Mao and X. Hu, Nat. Chem., 2021, 13, 270–277 CrossRef CAS PubMed;
(x) Y. He, H. Song, J. Chen and S. Zhu, Nat. Commun., 2021, 12, 638 CrossRef CAS PubMed;
(y) J. W. Wang, Y. Li, W. Nie, Z. Chang, Z. A. Yu, Y. F. Zhao, X. Lu and Y. Fu, Nat. Commun., 2021, 12, 1313 CrossRef CAS PubMed;
(z) P.-F. Yang, J.-X. Liang, H.-T. Zhao and W. Shu, ACS Catal., 2022, 12, 9638–9645 CrossRef CAS;
(a
a) P.-F. Yang, L. Zhu, J.-X. Liang, H.-T. Zhao, J.-X. Zhang, X.-W. Zeng, Q. Ouyang and W. Shu, ACS Catal., 2022, 12, 5795–5805 CrossRef CAS.
- A. W. Schuppe, J. L. Knippel, G. M. Borrajo-Calleja and S. L. Buchwald, J. Am. Chem. Soc., 2021, 143, 5330–5335 CrossRef CAS.
- J. Liu, H. Gong and S. Zhu, Angew. Chem., Int. Ed., 2021, 60, 4060–4064 CrossRef CAS.
- R. K. Dhungana, V. Aryal, D. Niroula, R. R. Sapkota, M. G. Lakomy and R. Giri, Angew. Chem., Int. Ed., 2021, 60, 19092–19096 CrossRef CAS PubMed.
-
(a) R. Matsuura, T. C. Jankins, D. E. Hill, K. S. Yang, G. M. Gallego, S. Yang, M. He, F. Wang, R. P. Marsters, I. McAlpine and K. M. Engle, Chem. Sci., 2018, 9, 8363–8368 RSC;
(b) Z. Q. Li, O. Apolinar, R. Deng and K. M. Engle, Chem. Sci., 2021, 12, 11038–11044 RSC.
- For more details on the condition optimization, see the ESI†.
Footnotes |
† Electronic supplementary information (ESI) available. CCDC 2131923. For ESI and crystallographic data in CIF or other electronic format see https://doi.org/10.1039/d2sc04350e |
‡ J. X. Z. and P. F. Y. contributed equally to this work. |
|
This journal is © The Royal Society of Chemistry 2022 |
Click here to see how this site uses Cookies. View our privacy policy here.