DOI:
10.1039/D2BM00836J
(Paper)
Biomater. Sci., 2023,
11, 181-194
Local epidermal growth factor delivery using nanopillared chitosan–gelatin films for melanogenesis and wound healing†
Received
26th May 2022
, Accepted 6th November 2022
First published on 16th November 2022
Abstract
Epidermal growth factor (EGF) is required for various regulations of skin tissue including wound healing; however, it has limited stability due to the physicochemical conditions of the wound milieu. The lack of functional EGF within the wound can cause permanent tissue defects and therefore, current wound patch designs involve EGF-releasing components. Consequently, the focus of such systems is to improve the wound healing mechanism, with minimal attention on melanogenesis of the scar tissue. The present study investigates in vitro/in vivo wound healing and melanogenesis potential of the EGF-doped films comprised of arrays of chitosan:gelatin nanopillars (nano C:G films) prepared by using nanoporous anodic alumina molds. The potential of EGF-doped films in wound healing was examined with individual and coculture systems of fibroblasts and melanocytes to mimic the wound conditions. The outcomes demonstrated that compared to the control groups, the combination of EGF doping and nanotopography consistently provided the highest levels of melanogenic activity-related genes, melanin contents as well as EGFR expressions for both melanocyte-only and coculture setups. Proteomic, genomic and histological analysis of the excisional wound model further demonstrated that if EGF was present within the nanostructured films, the performance of these substrates in terms of wound closure, collagen thickness as well as melanin deposition was considerably improved. Furthermore, when compared with the control saline treatment and healthy mice groups, significant differences for such parameters were obtained for the nano C:G films, irrespective of their EGF contents. Overall, the results indicate that EGF-doped nano C:G films are good candidates as wound patches that not only provide desirable healing characteristics but also cause improved melanogenic outputs.
1. Introduction
Skin is the largest organ of the human integumentary system which functions as a physical barrier from external damage and regulates body temperature, peripheral circulation and fluid balance via hair and sweat formations.1 However, chronic or acute injuries can easily deform the multi-layered structure of skin causing complications such as infections, melanoma or scar tissue formation. Therefore, the treatment of such injuries requires the application of artificial skin barriers that can support cellular activity in a moist environment, allow transport of gaseous species, but at the same time, prevent pathogenic transmission.2
Wound healing mechanism comprises four steps: hemostasis, inflammation, angiogenesis and re-epithelization. Melanogenesis also plays a crucial role in the generation of healthy skin tissue,3 as melanocytes have melanosomes to produce melanin pigment, the main source of skin coloration. Lack of melanin may cause the formation of various cancer types and serious sunburns.4 Therefore, the design of biodegradable skin barriers that can control healing and melanocyte activity is a critical requirement for successful skin tissue engineering applications. However, melanogenesis and wound healing strategies are rarely addressed together and only few studies have been reported.4–6 For example, Shi et al. proposed the use of tyrosinase-doped gelatin methacrylate (GelMA)-collagen mixture to test melanocyte activity besides the behavior of other types of skin cells.5 The results showed that tyrosinase-doped films have a potential to speed up the wound healing process and melanocyte activity.
Recent developments in skin tissue engineering also dictate the importance of mimicking the nanoscale topography of the extracellular matrix (ECM) of the injured tissue to attain the desired biological performance involving enhanced melanogenesis.7,8 Like many other tissues, skin ECM is also primarily composed of nanoscale components including the collagen fibers (50–500 nm in diameter and parallel to each other, similar to the nanopillar arrays fabricated in this study) and aggrecan structures, which contain hierarchical glycosaminoglycan branches with contour lengths of about 400 nm. Electrospinning, lithography, anodization, or molding-based methods are some common approaches to fabricate biomaterials with such nanosized topographic features.9 However, high fabrication costs, low resultant yields and lack of reproducibility make these methods unfavorable compared to drop-casting strategies.10
Notably, nanoporous anodic aluminum oxide molds (AAMs), which can be easily customized for physical properties such as porosity, pore diameter and pore depth, provide unique opportunities for the facile fabrication of large-area nanopatterned polymer surfaces via drop-casting.11 For instance, we have recently reported the formation of multi-branched polycarbonate nanostructures, simply by changing the electrolyte type and anodization voltage values in the general AAM fabrication protocol.12
Additionally, these topographical factors can be supported by biochemical cues through loading the constituent nanostructures with specific biological molecules such as growth factors, peptides, or genetic materials to improve wound healing and melanogenic activities. For example, epidermal growth factor (EGF) plays a crucial role in the metabolism of skin cells, including keratinocytes, melanocytes and fibroblasts and several skin barrier platforms with nanoscale topographic features were loaded with EGF where successful improvement of the overall healing process was reported via controlled EGF release.6,13–15
The majority of such platforms are fabricated by using natural polymer mixtures due mainly to chemical similarity with native ECM environment, biocompatibility, low cost, large-scale fabrication and potential bactericidal activity.16,17 For instance, chitosan–gelatin mixtures have been recommended as skin barriers in many studies.18,19 Gelatin, in particular, promotes the mechanical stability of scaffolds by electrostatically interacting with chitosan under acidic pH settings20,21 and creates an adhesive environment for cells due to an abundance of RGD sequences. Gelatin also prevents proteolysis of many growth factors during the wound healing process.22 On the other hand, it has already been well-documented that chitosan is a good candidate for generating bactericidal, biocompatible and bioadhesive skin barriers and is widely used in commercial wound dressing applications.23 To improve its mechanical properties, chitosan is commonly mixed with gelatin.20,24 This combination not only provides a more durable biomatrix, but it can also allow controlled release of biological factors, as well as presents a safeguard from wound environment to molecules like EGF that has reported sensitivity to wound-milieu.22
We recently fabricated chitosan:gelatin films (nano C:G films) decorated with arrays of nanopillars by using AAMs in a drop casting setup and presented their antimicrobial activity and osteogenesis potential in vitro conditions for stem cells and mature osteoblasts.25 In here, these nanostructured films were doped with EGF and were used to regenerate healthy skin tissue in vivo using an acute excisional wound healing model. To the best of our knowledge, there is no literature about the use of EGF-loaded bionano-polymeric films for melanogenic applications. We tested the effects of the nanotopography, the amount of EGF release on the wound healing process and melanogenic activity-related cellular processes both in vivo and in vitro. Expression levels of genes, including tyrosinase (Try), tyrosinase-related protein-1 (TRP-1) and tyrosinase-related protein-2 (TRP-2), were examined in response to changing topography and EGF release profile. The results suggested that the nano C:G films can be alternative bifunctional barriers that can enhance wound healing as well as the melanogenic activity around the wound.
2. Experimental section
2.1. Fabrication of EGF-doped C:G nano and flat films
The drop casting strategy was utilized to fabricate nano C:G films via nanoporous AAO molds prepared by the two-step anodization protocol followed by a hydrophobic silane (octadecyltrimethoxysilane, Alfa Aesar, L06366) treatment. The details of AAO fabrication and mold modification are described elsewhere in the literature.10 To fabricate the flat polymer controls, a similar silane treatment was followed using a Si wafer.
In order to obtain EGF-doped films, an EGF (GenScript, Z02691) solution with 254 ng ml−1 final EGF concentration was prepared in chitosan–gelatin mixture. This C:G mixture (1.25/0.25 w/w; medium molecular weight chitosan; 190–310 kDa; 75–85% deacetylation degree; Sigma-Aldrich; skin-derived bovine gelatin, Helavet Ind.) was prepared in a 0.3% (v/v) acetic acid solution. To increase the stability of the film, poly(ethylene glycol) diglycidyl ether (PEGDE), a well-known biocompatible cross-linker, was also added to the C:G solution (0.3% (v/v)) at pH 7.5.25 The mold surface area and utilized mixture volume calculations showed that after the drop-casting and solvent evaporation, the EGF amount present in each 6 mm punch-sized (28.2 mm2 surface area) C:G film is 14.5 ng. EGF-dropped films were prepared by directly dropping the EGF solution on the films’ surface. The amount of EGF on dropped films was kept same as EGF-doped films.
2.2. Morphological evaluation of the films
Morphology of the AAO molds and nano C:G films were determined using SEM (Quanta 200, FEI, 177 Hillsboro, OR, USA, 10 mm working distance, 5 kV acceleration 178 voltage). The samples were coated with 10 nm Pd–Au using a precision coating system (GATAN) and were then scanned using SEM. ImageJ software was used to determine the size of the structures.
2.3.
In vitro EGF release assay
After the formation of EGF-doped nano and flat C:G films, the films were placed into 96-well plates and the release profile was followed in phosphate buffer (10 mM) solution at 37 °C, 5% CO2 humidity and pH 7.5. The supernatants were then centrifuged at 1kg (4 °C) and the amount of EGF released into the solution was detected following the manufacturer's instructions for ELISA (Thermo Scientific, KHG0061). The EGF amount was expressed as ng ml−1.
2.4. Cell culture conditions and viability assay
NIH-3T3 and B16-F10 (American Type Culture Collection; VA) were cultured in DMEM supplemented with 20% FBS and 1% antibiotics (penicillin/streptomycin) and maintained at 37 °C and in 5% CO2 humidified incubator. The experiments were conducted using cell passages 10–20.
MTT viability assay (Thermo Scientific, M6494) was conducted to assess the cell viability of melanocytes, fibroblasts and cocultured cells grown on the C:G films following the manufacturer's instructions. Briefly, the cells grown on films for 72 h were rinsed with PBS after incubation and MTT reagent was added to the cells. Then the cells were incubated at 37 °C and in 5% CO2 humidified incubator. Then the mediums were replaced with DMSO and the absorbance of the dissolved tetrazolium salt was read out at 540 nm. The viability assay details are presented in this manuscript's ESI† section.
2.5. Quantification of total EGFR and fibronectin content
The cells (104 cells per well) were added onto the C:G films in a 96-well plate and were incubated for 72 h. The cells grown on TCP plates were used as the control group. After the end of the incubation period, the cells/films were rinsed with phosphate-buffered saline (PBS) two times and then the plates were incubated with RIPA lysis buffer (Thermo Scientific, 89901) on ice for 15 min. The solutions were then transferred to microcentrifuge tubes and samples were centrifuged at 10kg for 30 min to separate the protein solution from the cell mixtures. The total protein amount from sample to sample was normalized via micro-BCA analysis (Thermo Scientific, 23235). The EGFR content was then quantified in the collected supernatants using ELISA (BosterBio, EK1433) following the manufacturer's instructions. The results were expressed as μg mg−1 protein. All the cell experiments were conducted with 3 independent experiments per group (n = 3). The fibronectin content was quantified in the supernatants using ELISA (BosterBio, EK0351) following manufacturer's instructions. The results were expressed as μg mg−1 protein. The details of in vitro and in vivo fibronectin quantification experiments are presented in the ESI† section.
2.6. Cell imaging
To visualize the growth of melanocytes in a coculture system with fibroblasts, 104 melanocytes were added into the phenol red-free medium and the medium was supplemented with 5 μl ml−1 red-colored cytoplasmic membrane dye (CellBrite™, 30023). The cells were then incubated for 30 min at 37 °C in dark and centrifuged at 8000 rpm for 5 min at room temperature. The collected cell pellets were rinsed two times with DMEM media. Then the pellets were resuspended in DMEM media and were mixed with fibroblasts in a 5
:
1 ratio. The cells were then added onto C:G films and the cells were incubated for 72 h before taking images using an optical microscope (Keyence, Japan).
To visualize both fibroblasts and melanocytes in the films, fibroblasts were stained with red colored cytoplasmic membrane dye (CellBrite™, 30023) and melanocytes were stained with yellow-orange colored cytoplasmic membrane dye (CellBrite™, 30022). After staining, both cells were added to the films at a 5
:
1 ratio and were incubated for 72 h. Post-incubation, the films (with cells) were coated with mounting medium (VECTASHIELD® Antifade Mounting Medium with DAPI), where DAPI stained nucleus. The confocal fluorescence images were taken using Zeiss Axio Observer Z1 inverted microscope and were analyzed using ImageJ software.
2.7. Total RNA isolation and qPCR analysis
Monocultured melanocytes were seeded on C:G films at a density of 2 × 105 cells per well of a 12-well plate. On day 3 post-seeding, the cells were dislodged using trypsin-EDTA solution (Sigma Aldrich, T4049), centrifuged at 1kg for 5 min and the collected cell pellets were washed 3× with PBS. Total RNA was isolated using Quick-RNA™ isolation kit as per the manufacturer's instructions (Zymo Research, CA). The concentration of RNA was measured using Nanodrop 2000 (Thermo Scientific). RNA (1 μg) was reversely transcribed into cDNA using cDNA synthesis kit (Applied Biosystems) following the manufacturer's protocol. The expression of tyrosinase, TRP-1 and TRP-2 mRNA was quantified by 7300 Real-Time PCR System from Applied Biosystems using the cycle program for SYBR® green reaction. GAPDH was used as a housekeeping gene. The comparative Ct method was used to analyze expression of mRNA. The following primers were used for qPCR studies of monocultured melanocytes:26 tyrosinase: (F: 5′-CTCTGGGCTTAGCAGTAGGC-3′; R: 5′-GCAAGCTGTGGTAGTCGTCT-3′), TRP-1 (F: 5′-GCTGCAGGAGCCTTCTTTCTC-3′; R: 5′-AAGACGCTGCACTGCTGGTCT-3′), TRP-2 (F: 5′-TGGAGTACGTCAAGCAGGAG-3′; R: 5′-ATTCGGTTGTGACCAATGGGT-3′) and GAPDH (F: 5′-GGTCCTCAGTGTAGCCCAAG-3′; R: 5′-AATGTGTCCGTCGTGGATCT-3′).
2.8. Total melanin quantification
For melanin quantification, synthetic melanin was purchased from Sigma Aldrich and the standard curve was prepared using the melanin solution. For melanin quantification, all possible sources were used including intra-cellular melanin, melanin released into the cell media and melanin extracted from films. Firstly, intra-cellular melanin was extracted from the cells using 1 N NaOH + 10% dimethyl sulfoxide solution. The samples were centrifuged at 10kg for 15 min and supernatants were read at 405 nm. Secondly, melanin released into the cell media was detected by directly reading the collected solution at 405 nm. Lastly, the amount of residual melanin in the films was detected by incubating the films in 1 M acetic acid solution for 3 days in dark. After 3 days, the solutions were read at 405 nm for melanin detection. Finally, for total melanin amount, above mentioned three groups were added together. Melanin content was expressed as μg mg−1 protein.
2.9. Full-thickness in vivo wound model
All animal procedures were performed following the Guidelines for Care and Use of Laboratory Animals of Northeastern University and approved by the Institutional Animal Care and Use Committee (ICAUC; protocol 17-0516R). C57BL/6 male mice (8 to 10-week-old, average wt 30 g) were procured from Charles River (MA, USA). Animals were kept for 48 h before the surgery at the facilities with 12 h light/dark cycles and food and water ad libitum. 24 h prior to surgery, dorsal skin of the mice was shaved and residual hair layer were cleaned using a commercial hair-removing cream. On surgery day, the mice were anesthetized via inhalation of 4% isoflurane in an induction chamber. Following the procedure, mice were positioned in ventral recumbency on the 37 °C heated blanket to keep their body temperature stable. Additionally, the mice were connected to a breath circuit (2% isoflurane) to provide maintenance of anesthesia. Before the incision step, 5–10 mg kg−1 of meloxicam in 150 μl of sterile saline was ejected subcutaneously. Then, the surgical area was disinfected using iodine and 70% ethanol. 6 mm sterile biopsy punches were used to generate bilateral full-thickness wounds on the dorsum. The silicon splints (inner diameter = 6 mm, outer diameter = 9 mm, thickness = 1 mm) were then immobilized on the wounds with sutures. The animals were randomly assigned to the three groups: (1) saline treated, (2) EGF-doped nano C:G films and (3) EGF-free nano C:G films; (n = 3 per group, 2 wounds per animal). For the saline-treated group, 50 μl of saline were pipetted on the top of each wound and the films were placed onto wounds for the other two groups. Then the wounds were covered with Tegaderm films and protected using bandages. Meloxicam (5–10 mg kg−1) was injected subcutaneously after analgesia procedure.
The mice were daily weighed and observed for symptoms of pain or discomfort and wounds were photographed digitally to monitor wound closure. No additional treatments were provided and Tegaderm was not altered/changed to protect the wound area from any trauma. ImageJ program was used to calculate wound closure for each photograph of the wounds (n = 3). On days 5 and 10 post-surgery, the animals were euthanasia via CO2 inhalation followed by cervical dislocation. The full-thickness wounds and 1–2 mm of the adjacent area were harvested for histopathological examination, protein and qPCR analyses.
2.10. Evaluation of melanogenic activity of the skin biopsies
After the protein extraction procedure, total protein content was measured using BCA assay for the skin biopsy samples. Total melanin amount was analyzed from 50–150 mg skin biopsy samples. The samples were frozen by soaking in liquid nitrogen and were subsequently homogenized in 5 ml water. Then, the samples were centrifuged at 1kg for 20 min and the pellets were washed with 5 ml of 0.05 M (pH 6.8) phosphate buffer solution to remove soluble components of the tissues. The pellets were then centrifuged in chloroform
:
methanol (2
:
1) and ethanol
:
ether (3
:
1) solutions to remove fatty tissue, respectively. Afterwards, the samples were dried under nitrogen gas and hemoproteins were removed using 0.3% saponin, 0.9% NaCl aqueous solution. Then, the samples were first treated with 0.15 M NaCl + 5 mM MgCl2 solution and were later incubated with mixture of 0.05 M Tris buffer and 2 mg ml−1 pronase-CB solution (Sigma Aldrich, 10165921001) at 30 °C for 48 h. After incubation, the samples were centrifuged for 30 min at 10kg and were washed with 0.9% NaCl solution and water. The final pellets collected were dissolved in 1 N NaOH and the absorbance values of samples were recorded at 405 nm. Standard curve was prepared using commercial melanin powder (Sigma Aldrich, M0418). Total melanin content was expressed as μg mg−1 protein.
qPCR analyses were conducted to quantify the gene level expression of tyrosinase, TRP-1 and TRP-2. The skin biopsy samples were stored in RNAlater solution (Thermo Fisher, AM7020) at −20 °C right after the extraction of samples. For RNA extraction, the samples were weighed and were triturated using a mortar and pestle under liquid nitrogen. Total RNA was isolated from the pellets using 600 μl TRIzol solution (Thermo Fisher, 15596018) following the manufacturer's protocol. The concentration of RNA was measured using Nanodrop 2000 (Thermo Scientific). RNA (1 μg) was reversely transcribed into cDNA using cDNA synthesis kit (Applied Biosystems) following manufacturer's protocol. The expression of tyrosinase, TRP-1 and TRP-2 mRNA was quantified by 7300 Real-Time PCR System from Applied Biosystems using the cycle program for SYBR® green reaction. GAPDH was used as a house keeping gene. Primer sequences that were mentioned in the above section were used. The comparative Ct method was used to analyze expression of mRNA.
2.11. Histological evaluation of the skin biopsies
After extraction, the skin biopsy samples were fixed in 10% formalin for 24 h. Then, the samples were first incubated in 30% sucrose solution and later in 100% FBS for overnight at 4 °C. Next, the biopsy samples were washed using PBS buffer and were flash-frozen in liquid nitrogen in Optimal Cutting Temperature (OCT) solution. 10 μm cryosections were acquired using a CryoStar™ NX70 Cryostat and all the sections were processed for hematoxylin & eosin and Masson's trichrome staining, following published protocols.17 All the sections were visualized on an inverted microscope (Keyence, Japan), (n = 3).
2.12. Data analysis
Data analyses was determined using 1- or 2-way ANOVA test and Tukey post test with Microsoft Excel software. Error bars represent mean ± standard error mean (SE) of measurements. Data values in cell experiments were presented as mean ± SE, n = 3 individual experiments per group. Differences were considered significant at *p < 0.05 (for comparing groups with saline and other groups), #p < 0.05 (for comparing groups with healthy tissue), **p < 0.005 and ***p < 0.0005.
3. Results and discussion
3.1. Physicochemical analyses of nano C:G films
The nano C:G films were synthesized by using a drop casting strategy,24 where, a C:G solution was applied on nanoporous AAMs and upon solvent evaporation, the C:G films were peeled from the mold's surface (Fig. 1A). The morphological analysis of films was conducted using scanning electron microscope (SEM). The SEM analyses through ImageJ program revealed that the AAMs were decorated with arrays of nanopores having approximately 100 nm pore size with a 109 pore per cm2 pore density and the nanostructures generated from the C:G mixture via such molds have approximately 90 nm pillar diameter and 300 nm pillar height (Fig. 1B). The topography of the mold was successfully replicated with a small variation in diameter values, which was attributed to the solvent evaporation and the film peeling process.12 The flat films were prepared using a silicon wafer as a mold and then characterized with atomic force microscopy, which yielded an average surface roughness value of ∼7 nm. This value was approximately 30-folds lower compared to the roughness of the nano C:G films.25 Furthermore, the total surface area of nano C:G films was calculated using cylinders (pillars) with the above-mentioned dimensions and density and a 1.4-fold difference was found between the nanostructured and flat C:G films. Overall, the results showed that the fabrication of arrays of ordered nanopillared films can be achieved using the non-lithographic AAO-based drop-casting approach.
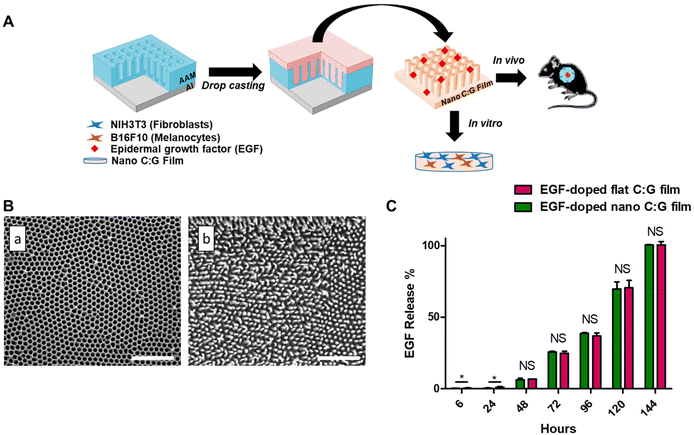 |
| Fig. 1 Fabrication route, morphology and release behavior of nano C:G films. (A) Schematic illustration of the formation of nano C:G films and their use in biological systems. Nano C:G films were designed by drop casting strategy. The films were used as a skin barrier, where epidermal growth factor (EGF) is released continuously and acts as a depot for cell migration in an in vitro coculture model of fibroblasts and melanocytes and in vivo excisional wound model (AAM: anodic aluminum oxide mold, Al: aluminum). (B) SEM images of (a) nanoporous AAMs and (b) EGF-doped nano C:G films, respectively. Scale bar denotes 1 μm. (C) In vitro release profile of EGF-doped nano and flat C:G films up to 144 h. The total EGF was loaded in the films at ∼14 ng per film. Data presented as mean ± SE, n = 3 per group. *p < 0.05; EGF-doped nano C:G films vs. EGF-doped flat C:G films. NS indicates not significant. | |
The overall effectiveness of EGF treatment in such doped matrices is significantly influenced by the release characteristics of EGF from the composite biomaterial.27 We measured the amount of released EGF from the nano and flat C:G films into phosphate buffer by conducting ELISA at various time points up to 144 h (Fig. 1C). Note that both films contain ∼14 ng of total EGF, they are both ∼60 μm thick and the nanopillars only span the top 0.3 μm. Moreover, according to our previous study, both C:G films display up to ∼30% swelling in 120 h.25 Due to these close similarities, the difference in the EGF release characteristics for flat and nano films was insignificant (p > 0.05) for all the time points investigated in this study. Effective EGF release could be observed after 48 h, where 0.918 ± 0.12 and 0.965 ± 0.11 ng ml−1 EGF was released by nano and flat films, respectively. At 72 h, 3.73 ± 0.18 ng ml−1 EGF was released from the flat film, whereas 3.61 ± 0.02 ng ml−1 EGF was released from the nano C:G film. The experiments were terminated after 144 h of release, where the total EGF in the release media was found to be ∼14 ng ml−1 for both film types. The EGF release from both films were fitted by using the Korsmeyer–Peppas model, which defines the release kinetics of hydrophilic matrixes. R2 values approaching unity were obtained for both the nanostructured and flat films (R2 = 0.994, Table S1†).28 The n value of the EGF-doped nano films was 1.73, while the value of the EGF-doped flat films was 1.66, indicating that anomalous transport occurred owing to Fickian diffusion and polymer relaxation as the films swelled.29
Several reports discussed the toxicity of excessive EGF levels both for in vitro and in vivo studies.27,30,31 In particular, the report by Ramineni and coworkers successfully demonstrated that a concentration regime between 1–5 ng mL−1 EGF provided maximal cell migration, cellular differentiation and wound healing.32 Similar EGF levels were attained after 72 hours in our case; hence, this time point was chosen for the following in vitro investigations.
3.2.
In vitro studies
Wound healing is a complex process containing contributions from various cell types involving melanocytes, fibroblasts and keratinocytes. The melanocytes are known to reside among the keratinocytes in the innermost layer of epidermis and there is an indirect interaction between fibroblasts (present in dermis) and melanocytes. Fibroblast secretions such as keratinocyte growth factor, basic fibroblast growth factors or transferrins have known effects on the proliferation, differentiation and melanogenesis of melanocytes.33 Despite this fact, majority of the related studies utilize monoculture systems and only few reports focus on the interaction between fibroblasts and melanocytes.3,33–35 Therefore, we performed the in vitro tests using NIH/3T3 cells (fibroblasts), B16-F10 cells (melanocytes) as well as coculture of these two cell lines (NIH/3T3
:
B16-F10 in 5
:
1 ratio).
3.2.1. Evaluation of cell viability.
To assess the biocompability of the nano and flat C:G films; we performed MTT assay using NIH/3T3, B16-F10 and cocultured cells (NIH/3T3:B16-F10) grown on the films with or without EGF on day 3 post-treatment. The viability results revealed that both mono and cocultured cells showed >98% cell viability for the nano or flat films, regardless of their EGF content, compared with the tissue culture polystyrene (TCP) control group (Fig. 2A). Our previous work also shows that these films support cell growth for osteoblasts and stem cells, with no cytotoxicity25 and therefore would be apt for cell culture studies or in vivo applications. We have also prepared EGF-dropped samples to elucidate the influence of controlled EGF release by depositing 14 ng of EGF from a stock solution onto the surfaces of nano and flat C:G films as well as TCP controls. As this protein quickly dissolves upon the addition of cell media, an immediate increase in local EGF in these groups caused drastic cell death, especially for the melanocytes and coculture group.
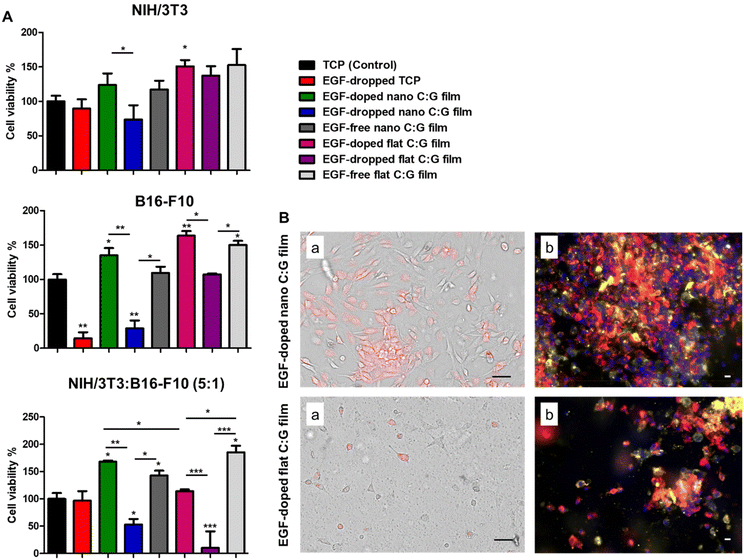 |
| Fig. 2 Cell viability measurements (A) and optical/fluorescence microscopy images (B) for NIH/3T3 (fibroblasts), B16-F10 (melanocytes) and cocultured cells (NIH/3T3 : B16-F10 (5 : 1)) grown on nano and flat C:G films with or without EGF content. (A) Cell viability measurements of the cells was measured by using MTT assay on day 3 post-treatment. Data presented as mean ± SE, n = 3 per group. *p < 0.05, **p < 0.005, ***p < 0.0005; TCP control vs. test groups. *p < 0.05, **p < 0.005, ***p < 0.0005; between test groups. (B) Optical (a) and confocal (b) microscope images of the cocultured cells (NIH/3T3 : B16-F10 (5 : 1)) grown on EGF-doped nano and EGF-doped flat C:G films, respectively, after 3 days of incubation. For optical images, the red color represents melanocytes and the rest of the cells are fibroblasts. For confocal images, blue color (DAPI) represents the cell nucleus, red color represents fibroblasts and yellow-orange represents melanocytes. Scale bars denote 50 μm. | |
Interestingly, the MTT data also indicated a significant viability difference between the EGF-doped nano and flat C:G films for the coculture experiments. As these surfaces only differ by the surface topography faced by the cells, further examination of cell viability and growth on these substrates were carried out using optical and fluorescence microscopy. Herein, to observe the growth of melanocytes in the coculture system in the former setup, melanocytes were pre-labeled with a red dye and mixed with fibroblasts before seeding onto the films. Top-view optical images of live cells on the substrates indicate that the EGF-doped nano C:G films could enhance both melanocyte (red-colored cells) and fibroblast (non-colored cells) growth compared to their flat counterparts (Fig. 2B). The cocultured cells were also imaged using fluorescence microscopy after fixation, which again confirmed the apparently higher population of melanocytes (yellow-orange cells) as well as fibroblasts (red-colored cells) for the nanostructured films. Although our results indicate that nanotopography is influential in the cellular viabilities plausibly via the improved formation of focal adhesion points on such surfaces,36 previous studies with coculture systems have noted the importance of fibroblast secretions on proliferation, migration and differentiation of the melanocytes3,33 and these factors should also be taken into account for the observed differences.
3.2.2. Cellular EGFR and fibronectin expressions on C:G films.
The complex structure of skin, hosting several different cell types from keratinocytes to fibroblasts, also homes indirect interactions of secreted biomolecules in inducing several pathways from melanin synthesis to tissue regeneration (Fig. 3A). Among these secretions, fibronectin plays critical roles in wound healing stages like clot formation, development of granulation tissue, re-epithelialization as well as melanocyte differentiation and proliferation.37,38 It is also reported that fibronectin secretion in human fibroblasts is upregulated by EGF.39 Therefore, we assessed total fibronectin content in monocultured fibroblast and cocultured cells were grown on the nano and flat films in the presence or absence of EGF (Fig. 3B and C). Fibronectin expression analysis was firstly conducted with monocultured fibroblasts. The results showed the statistical difference between the EGF-doped (6.62 ± 1.53 μg mg−1 protein) and EGF-free nano C:G films. Moreover, EGF-free nano C:G films (11.32 ± 0.83 μg mg−1 protein) have highly increased fibronectin levels compared with the TCP control (7.20 ± 0.39 μg mg−1 protein) as well as EGF-free flat films (5.46 ± 0.33 μg mg−1 protein). Interestingly, the flat C:G films showed a significant decrease in fibronectin content compared to TCP independent of their EGF load. Overall, the results showed that the fibronectin expression is enhanced by nanotopography, not by EGF content of the films, for the monocultured fibroblasts.
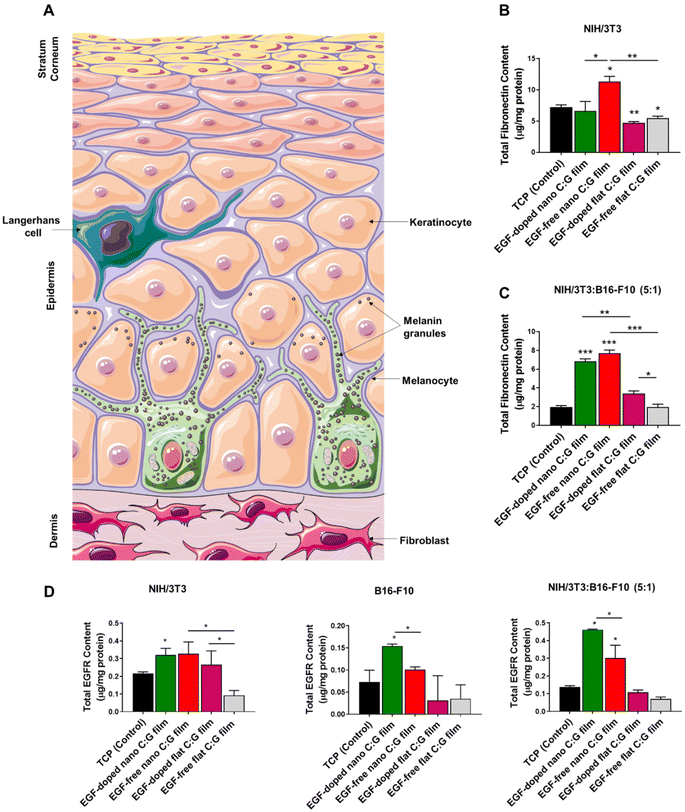 |
| Fig. 3 Fibronectin content measurements of NIH/3T3 (fibroblasts) and cocultured cells (NIH/3T3 : B16-F10 (5 : 1)) grown on nano and flat C:G films post day 3 of incubation. (A) Schematic representation of different layers and cells in a full thickness skin. Total fibronectin content measurements of (B) fibroblasts and (C) the cocultured cells grown on nano and flat C:G films, respectively. Cells grown on TCP were used as control group. (D) Total EGFR content measurements of NIH/3T3 (fibroblasts), B16-F10 (melanocytes) and the cocultured cells grown on nano and flat C:G films after 3 days of incubation. Cells grown on TCP were used as control group. Data presented as mean ± SE, n = 3 per group. *p < 0.05, **p < 0.005, ***p < 0.0005; TCP control vs. test groups. *p < 0.05, **p < 0.005, ***p < 0.0005; between respective test groups. | |
We used also the coculture setup to observe the effects of topography and EGF on fibronectin expression in the presence of melanocytes (Fig. 3C). Parallel results as the monoculture experiments were attained where the highest fibronectin was detected for the EGF-free nano C:G films. The presence of EGF in such films slightly lowered the amount of fibronectin; however, the difference is not significant and both nano C:G films provided >3 times higher protein synthesis compared to TCP. The influence of topography also becomes apparent when EGF-free nano film is compared to EGF-free flat film or EGF-doped nano film is compared to EGF-doped flat films. For instance, for the latter comparison, the amount of detected fibronectin is about 2-folds higher for the nanostructured counterpart. Moreover, it can also be inferred from these results that the biopolymeric matrix did not enhance fibronectin expression as the flat EGF-free composite films performed worse (in monoculture) or similar (in coculture) when compared to the TCP control. It should be noted, however, that presence of EGF in the flat films causes a slight but significant increase in fibronectin level. Taken together, these results showed that the fibronectin expression is mainly influenced by the nanotopography of the films and EGF-doping as well as the biochemical content of the films had minor effects.
We next evaluated the EGF receptor (EGFR) profiles of the fibroblasts, melanocytes as well as their coculture incubated with C:G films possessing different topographies and EGF contents (Fig. 3D). The results revealed that the only film type with significant improvement in total EGFR content with respect to TCP, irrespective of the culture type, was EGF-doped nano C:G films. Other than that, only EGF-free nano C:G films induced a drastic EGFR increase (>2 folds) compared with the TCP control in the coculture case, despite being statistically lower than the EGF-loaded counterpart. These two observations dictate that both nanotopography and EGF presence are effective in increasing the EGFR contents. The former factor also takes place in fibroblast EGFR profiles, where EGF-free nano C:G films caused ∼3-folds higher EGFR expression compared to EGF-free flat ones. The influence of EGF, on the other hand, was further confirmed, where its presence caused significant raise for the melanocyte EGFR levels with regards to nano C:G films and for the fibroblast EGFR levels with regards to flat ones. The latter observation is in line with the literature as several research groups have reported overexpression of EGFR in fibroblasts after incubation with EGF.40
Note that, when the total fibronectin levels of EGF-doped and EGF-free nanotopographic films were compared in Fig. 3B and C, the significant difference present for single fibroblasts was lost for the coculture experiments. This loss might be due to the large EGFR expression for the EGF-containing nano C:G films in the coculture incubation (Fig. 3D). It has been shown by Yamaki and coworkers that the increment of EGF concentration upregulates EGFR release, which reduces the rate of fibronectin mRNA degradation from human fibroblasts.41 Hence, higher levels of EGFR receptor presence in the fibroblasts of coculture studies for EGF-doped nano films can increase fibronectin synthesis, which approaches to that of EGF-free counterparts yielding a non-significant difference for fibronectin levels (Fig. 3D).
3.2.3. Effects nanotopography and EGF on melanogenic activity-related cellular processes.
We examined the effect of nanotopography and EGF release on the melanogenic activity-related cellular processes to better understand their potential role in wound healing.33 This activity was first evaluated by measuring gene expression levels of tyrosinase, tyrosinase related protein-1 (TRP-1) and tyrosinase related protein-2 (TRP-2), which were known to be related with the melanogenesis process3 (Fig. 4A). The results showed that the only substrate that causes significantly improved mRNA expression levels for all tyrosinase, TRP-1 and TRP-2 proteins compared to the TCP (control) group was EGF-doped nano C:G films. Their melanogenesis potential was further confirmed with tyrosinase activity assays, which displayed that consistently improved enzyme activity of melanocytes as well as cocultured cells was only attained for the nanostructured films with EGF content (Fig. S1†). Fig. 4A also illustrates that, regarding the nano C:G films, the presence of EGF caused a drastic change (∼2.3-fold) only for the tyrosinase mRNA expression. Furthermore, EGF-free nano C:G films were also effective in causing difference for TRP-1 and TRP-2 mRNA levels compared to the control group, again stressing the importance of nanotopography. The presence of EGF did not alter the results for the flat films except for the TRP-1 levels, which resulted in about 4 times higher mRNA levels for the indicated protein with respect to TCP samples. The cocultured cells did not show any significant differences due to either nanotopography or EGF (data not shown).
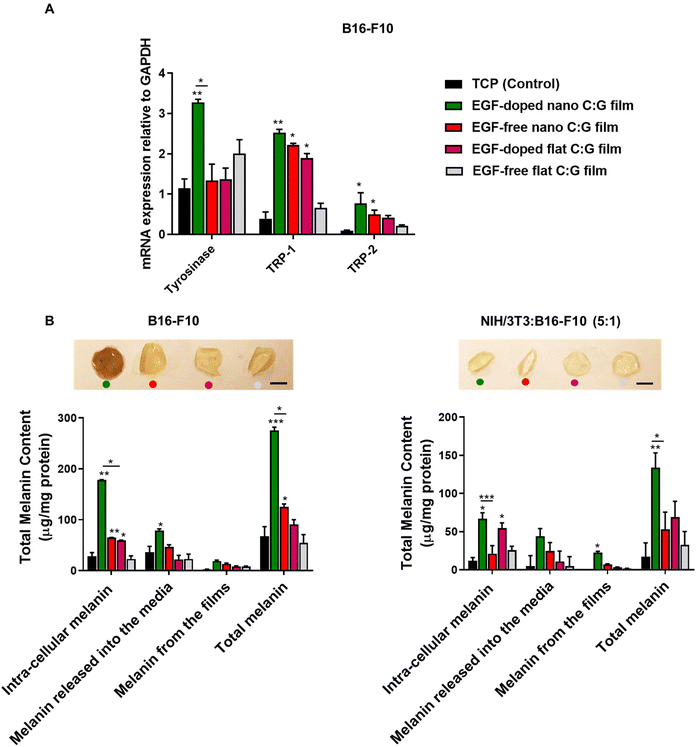 |
| Fig. 4 Melanogenic activity related genes and melanin content measurements of B16-F10 and cocultured cells (NIH/3T3 : B16-F10 (5 : 1)) grown on nano and flat C:G films, after 3 days of incubation. (A) qPCR analysis of tyrosinase, TRP-1 and TRP-2 genes to evaluate melanogenic activity of melanocytes grown on nano and flat C:G films in the presence/absence of EGF. The gene expression levels were expressed relative to GAPDH mRNA expression for respective groups. (B) Total melanin content of melanocytes and cocultured cells grown on the nano and flat C:G films prepared with or without EGF. Cells grown on TCP were used as control group. The photographs of the films represent residual melanin accumulation on the respective films at day 3, post-incubation with the cells. Scale bar denotes 3 mm. Data presented as mean ± SE, n = 3 per group. *p < 0.05, **p < 0.005, ***p < 0.0005; TCP control vs. test groups. *p < 0.05, ***p < 0.0005; between respective test groups. | |
Further, we quantified the melanin pigment collected from monocultured melanocytes and coculture systems after 3 days of incubation (Fig. 4B). In the case of melanocytes, the total melanin content of the EGF-doped nano C:G films was found to be higher compared with the TCP (control) group and EGF-free nano C:G film group. Similar trend was observed for the intra-cellular melanin content and melanin released into the media. For instance, the EGF-doped nano C:G films could stimulate melanocytes to synthesize approximately 3-fold more intra-cellular melanin (178.03 ± 0.92 μg mg−1 protein) compared with the EGF-free nano C:G films (65.06 ± 0.23 μg mg−1 protein, p < 0.05). Additionally, the secreted melanin from melanocytes was also found to be stuck on the films (inset photograph Fig. 4B). The images showed that the melanin accumulation on the films was apparently higher for the EGF-doped nano C:G films compared with other groups. However, when melanin from the films was extracted via membrane dissolution, only a minor was difference observed between substrate groups. The overall results suggested that nanotopography influences melanin secretion, but the combination of nanotopography and EGF doping seems to have a greater impact on the melanin secretion process of melanocytes.
The total melanin measurements for the coculture platform showed similar trend as the monocultured melanocytes. As expected, the extent of synthesized melanin was drastically decreased compared to monocultured melanocytes. Nevertheless, the amount of intra-cellular melanin for the EGF-doped nano C:G films (66.88 ± 7.89 μg mg−1 protein) was significantly higher than the EGF-free nano C:G films (21.33 ± 10.50 μg mg−1 protein) (p < 0.0005) as well as the TCP (control) group (11.78 ± 4.30 μg mg−1 protein) (p < 0.05). Total melanin content measurements also followed a parallel behavior. Besides, the C:G films photographed after 3-day of incubation of the cocultured cells did not show a visible color change in the films, which is probably due to lower amounts of adsorbed melanin compared to the monocultured melanocyte counterparts. It was interesting to note that, when compared to the TCP control, the presence of EGF in the flat films for both melanocyte and coculture experiments caused a significant difference only for the intra-cellular melanin levels.
Overall, the in vitro analyses of the cocultured cells and monocultures revealed that for most of the gene and protein studies, EGF-doped films possessing nanoscale topography outperformed their counterparts. The presence of topographical and biochemical cues in this substrate resulted in superior performances regarding the total EGFR and melanin contents for the former coculture experiments, which better represents the complex in vivo environment. The presence of EGF consistently seems to impact the results when it is embedded within the nanostructured films. The discrepancies between the EGF-loaded nano and flat films plausibly stem from both the nanoscale ECM-mimetic topography of the former substrates as well as their increased surface areas that yield a greater extend of cellular interactions in addition to a lower EGF local surface density. In the case of fibronectin, however, the EGF-free nano C:G film caused the highest protein synthesis for both monocultured fibroblast as well as the coculture experiments. Thus, in order to continue with the substrates displaying the highest in vivo potential for wound healing/skin coloration, the nanostructured films with and without EGF doping were selected for the remaining part of this work.
3.3.
In vivo study
In vivo studies were conducted using the rodent excisional model of wound healing,17 where nano C:G films with and without EGF (n = 3 for each) were placed on top of the wounds, generated on back side of the mice skin. The edge of the wounds was stabilized with silicon splints to prevent the normal contraction of the wound area and to better mimic the human wound healing mechanism42 and saline treatment of the generated wound was used as the control. Visual examination of the wounds revealed an immense difference in terms of wound closure for the EGF-doped nanostructured films at days 3, 5 and 7 compared to EGF-free counterpart. Still, especially when compared to saline control (Fig. 5A). To further elucidate this difference, such images were employed to extract wound closure percentage data for the samples investigated. The resultant figure illustrates that both of the nano C:G films showed marginal increase in wound closure till day 5 compared with the saline group (Fig. 5B). However, the wound closure enhancement caused by the EGF-doped nano C:G film becomes more evident between day 5 to day 7 post-treatment compared with the other two groups. Here, the nanostructured film with EGF doping caused 95 ± 12% wound closure, which was significantly higher than EGF-free nano C:G films (40 ± 10%) as well as the saline (control) (35 ± 12%) groups at day 7. After 10 days of treatment, all of the groups showed ∼100% wound closure, which was expected from a relatively small-sized wound (0.28 mm2).17
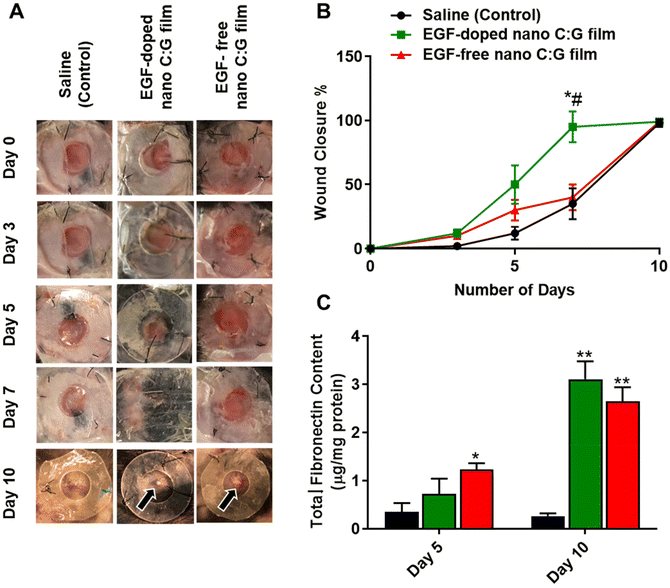 |
| Fig. 5 Wound area and total fibronectin content analysis of wounds treated with EGF-doped and EGF-free nano C:G film as well as the control. (A) Representative images of the wounds treated with saline (control), EGF-free and EGF-doped nano C:G films at various time points up to day 10 after treatment. The arrows indicate the residual films in the wound area. (B) Quantitative evaluation of wound closure. (C) Total fibronectin content measurements for the skin biopsy samples treated with saline (control), EGF-doped and EGF-free nano C:G films on day 5 and day 10 post treatment. Data presented as mean ± SE, n = 3 per group. *p < 0.05, **p < 0.005; saline (control) group vs. test groups. #(p < 0.05); EGF-doped vs. EGF-free nano C:G films. | |
We next investigated the fibronectin content of the wound biopsy samples as fibronectin is one of the main wound healing related proteins (Fig. 5C).43 The fibronectin levels were significantly higher in the EGF-free nano C:G film group (1.23 ± 0.13 μg mg−1 protein) compared with the saline (control) group (0.35 ± 0.18 μg mg−1 protein), on day 5 post-treatment. There was no significant difference between the EGF-doped nano C:G film group (0.72 ± 0.32 μg mg−1 protein) and the other two groups, For the extended 10-day treatment, however, statistically higher levels of fibronectin were expressed in the EGF-free and EGF-doped nano C:G film groups compared with the control. It was interesting to note that on day 10 post-treatment where complete wound closure was observed for all samples, the fibronectin levels of EGF-doped (3.10 ± 0.37 μg mg−1 protein) and EGF-free nano C:G films (2.64 ± 0.29 μg mg−1 protein) had very similar total fibronectin contents as the healthy tissue (2.69 ± 0.28 μg mg−1 protein, p > 0.05 for all three comparisons). The fibronectin expression profiles from the in vivo studies follow the trend in the in vitro experiments (see Fig. 3C and related discussion) and dictate that nanotopography of the films has the dominant role in fibronectin synthesis at early time points. This trend slightly shifts and EGF contributes to fibronectin synthesis for extended periods as observed in the synergistic influence of EGF on day 10 post-treatment (Fig. 5C).
To further elaborate the microscopic changes in the wound area during the healing process, we performed histological evaluation of skin biopsy samples collected after 10 days of treatment, using hematoxylin and eosin (H&E) and Masson's trichrome (MTC) staining techniques. H&E staining was utilized to show different skin cell types and identify the skin layers (Fig. 6A top row, Fig. S2†), while MTC was used to separate collagen fibers (blue) from the rest of the tissue (muscle, keratin and cell bodies: red, Fig. 6A bottom row). The EGF-doped and EGF-free nano C:G film groups showed effective wound healing, as demonstrated by the formation of new healed skin tissue on day 10 post-surgery. The H&E-stained samples showed the presence of purple-colored cells, which were accumulated around the wounds treated with both of the nanostructured films. Still, a slightly higher cell density was observed for the EGF-containing substrate (see the black arrow in the middle figure and also Fig. S2A† for higher magnification image). The purple cells can be blast cells have potential to differentiate into fibroblasts and their existence indicates that the wounds started to heal.44 Additionally, panniculus carnosus (PC) muscle formations were observed in EGF-doped C:G film applied wounds, which indicates the desirable healing compared to other test group for day 10 (Fig. S2B†).17
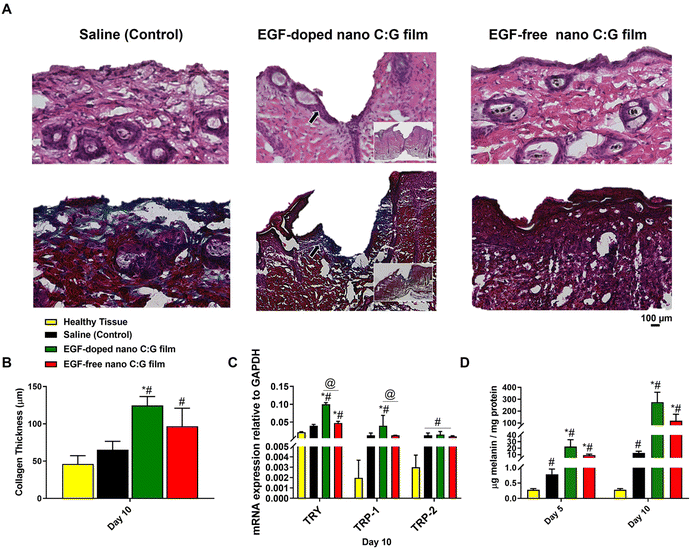 |
| Fig. 6 Histological analysis and melanogenic activity evaluation of wounds after 10 days of treatment with EGF-doped and EGF-free nano C:G films. (A) Hematoxylin and eosin (H&E) and Masson's trichrome (MTC) stained sections of a full-thickness wound samples collected after 10 days of treatment with saline (control), the EGF-free nano C:G film and the EGF-doped nano C:G film. (B) Collagen thickness quantification of wounds. Quantification of the collagen thickness was measured at the center of the wound using MTC stained tissue. EGF-doped nano C:G film treated wounds showed higher collagen deposition compared to the saline group as well as EGF-free nano film groups after 10 days of treatment. (C) qPCR analysis of tyrosinase, TRP-1 and TRP-2 genes were conducted for the wound biopsy samples treated with saline (control), EGF-doped nano C:G films and the EGF-free nano C:G films, on day 10 post-treatment. GAPDH was used as a housekeeping gene. The gene expression level was expressed relative to GAPDH mRNA expression for respective groups. (D) Total melanin content of the skin biopsy samples treated with saline (control), EGF-doped and EGF-free nano C:G films on day 5 and day 10 post treatment. Data presented as mean ± SE, n = 3 per group. *p < 0.05, **p < 0.005; saline (control) group vs. test groups. #(p < 0.05); wound treatment groups vs. healthy tissue. @(p < 0.05); EGF-doped nano C:G films vs. EGF-free nano C:G films. | |
Collagen formation also plays an important role during the proliferative stage of wound healing through enabling cell migration and ECM deposition. In order to evaluate the influence of wound treatment with the nanostructured films on collagen formation, histological assessment of the corresponding MTC-stained wound biopsies for different samples were carried out. Fig. 6B displays the collagen thicknesses obtained from healthy mice skin as well as the thicknesses from the wound treatment groups. As expected in such wound healing models, all treatment groups had higher collagen thicknesses compared to the healthy tissue (Fig. S3†),45 however, the difference is significant only for the nano C:G substrates. The highest collagen thickness values belong to EGF-doped nanostructured substrates (∼2-fold higher compared to saline control, p < 0.05) but the presence of EGF does not seem to cause a statistical difference between the nano C:G films. Compared to saline control, EGF-free nanostructured films also display higher levels of collagen deposition, but significantly different thickness values are observed only when EGF is present, which is known to induce ECM secretions, including collagen46 in addition to taking part during critical stages like angiogenesis during the whole wound healing process.47
In the final part of this study, certain indicators of the melanogenic activity within the wounds were analyzed through qPCR (tyrosinase, TRP1 and TRP2 genes), tyrosinase activity and total melanin content measurements (Fig. 6C, D and Fig. S4†). A common theme across all the biomolecules investigated was that healthy tissue constantly had the lowest levels of melanogenic indicators compared to the three treatment groups p < 0.05 in all cases except tyrosine mRNA levels compared to saline control). This observation is most probably related to the occurrence of inflammation in the wound site, where significant migration of immune cells including the monocytic melanocytes takes place.48 Furthermore, in all measurement, EGF-doped nano C:G films consistently had the highest level of detected biomolecule, however, when the nanostructured films were compared, the presence of EGF made a significant difference only for the tyrosinase mRNA expression levels. Regarding the genomic profiles investigated, same pattern was observed for the monocultured melanocytes as well (Fig. 4). TRP-1 and TRP-2 levels of wound treatment groups had very similar values, but this trio always had large differences between the healthy tissue.
Examination of the wound melanin contents revealed that the succession of melanin values for the different wound treatment groups follow an interestingly parallel pattern with that of tyrosinase mRNA levels as well as the tyrosine activities (Fig. 6C, D and Fig. S4†). For instance, the order of investigated groups is EGF-doped nano C:G > EGF-free nano C:G > saline (control) > healthy tissue for all tyrosinase gene expression, tyrosinase activity as well as the melanin content. Such results not only confirm the correlation between the tyrosinase levels and the resultant secreted melanin,49 but also dictate the protective role of the nanostructured films which act as a barrier to protect this oxidative and sensitive enzyme when compared to the saline control case. Note that both nanostructured films had order of magnitude higher levels of melanin compared to saline control for both treatment durations, implicating the potential of such films as wound patches for re-colorization of the injured tissue.
It is worth mentioning that, while the presence of EGF on the nanostructured films reproducibly created drastic changes in the melanin levels for the in vitro studies (Fig. 4), it had a weak influence in the excisional wound model. The role of EGF in melanogenesis is a debated topic in the literature. For example, Garcez et al. suggested that EGF induces formation of melanocytic phenotypes,50 while Yun et al. found that EGF does not have any significant effects on melanocytes.6 In the current study, EGF presence caused a similar trend as the former report especially in the in vitro case, however due possibly to complex pH, viscosity and cellular migration profiles at the wound milieu, a limited resultant functionality for EGF is attained in terms of enhancing melanogenesis. Such a result is not surprising since this growth factor is known to have a sensitive character in similar environments51 and one can potentially improve the role of EGF by doping larger quantities of the protein into the functional nanostructured films for further in vivo studies.
4. Conclusion
We have fabricated EGF-doped biopolymeric matrixes displaying arrays of nanopillars that possess both topographic and biochemical cues to enhance the wound healing mechanism as well as to induce melanogenesis around the wound area. In vitro studies with mono and coculture setups showed that, compared to the flat films, nanopillared surfaces with or without EGF had always improved cellular adhesion as well as fibronectin and EGFR profiles. The expression of the latter protein was positively influenced by the presence of EGF, which also caused immense differences for the melanogenetic activity-related genomic and proteomic markers when present within the nanopillared substrates. These EGF-doped nanostructured substrates also consistently yielded the highest collagen thickness, initial wound closure rate and melanin accumulation data for the in vivo excisional wound model. However, the statistically significant difference between the EGF-free counterparts was attained only for certain parameters investigated, involving tyrosinase, the level of which seemed to correlate well with the deposited amount of melanin. Taken together, major improvement in melanogenic activity as well as wound healing can be provided by nanopillared substrates compared to control groups and the presence of EGF can enhance their efficacy. As shown by our previous study, these films can also display antibacterial properties;25 hence, future studies will focus on evaluating the abilities of the EGF-doped nano C:G films to elicit wound healing using a physiologically relevant model of chronic wounds such as diabetes.
Conflicts of interest
There are no conflicts to declare.
Acknowledgements
S. A. would like to thank grants from the Scientific and Technological Research Council of Turkey TUBITAK BIDEB 2214-A Program (no. 1059B141601323).
References
- Z. Luo, W. Sun, J. Fang, K. Lee, S. Li, Z. Gu, M. R. Dökmeci and A. Khademhosseini, Adv. Healthcare Mater., 2018, 8, 1–9 CAS.
- M. Talikowska, X. X. Fu and G. Lisak, Biosens. Bioelectron., 2019, 135, 50–63 CrossRef CAS PubMed.
- Y. J. Wang, C. Viennet, S. Robin, J. Y. Berthon, L. He and P. Humbert, J. Dermatol. Sci., 2017, 88, 159–166 CrossRef CAS.
- S. L. Chadwick, C. Yip, M. W. J. Ferguson and M. Shah, J. Anat., 2013, 223, 74–82 CrossRef CAS PubMed.
- Y. Shi, T. L. Xing, H. B. Zhang, R. X. Yin, S. M. Yang, J. Wei and W. J. Zhang, Biomed. Mater., 2018, 13, 1–19 Search PubMed.
- W. J. Yun, S. H. Bang, K. H. Min, S. W. Kim, M. W. Lee and S. E. Chang, Dermatol. Surg., 2013, 39, 1903–1911 CrossRef CAS PubMed.
- M. Huzaira, F. Rius, M. Rajadhyaksha, R. R. Anderson and S. Gonzalez, J. Invest. Dermatol., 2001, 116, 846–852 CrossRef CAS PubMed.
- L. G. Parkinson, S. M. Rea, A. W. Stevenson, F. M. Wood and M. W. Fear, Tissue Eng., Part A, 2012, 18, 703–714 CrossRef CAS.
- S. Altuntas, F. Buyukserin, A. Haider, B. Altinok, N. Biyikli and B. Aslim, Mater. Sci. Eng., C, 2016, 67, 590–598 CrossRef CAS PubMed.
- B. Daglar, G. B. Demirel, T. Khudiyev, T. Dogan, O. Tobail, S. Altuntas, F. Buyukserin and M. Bayindir, Nanoscale, 2014, 6, 12710–12717 RSC.
- B. Daglar, T. Khudiyev, G. B. Demirel, F. Buyukserin and M. Bayindir, J. Mater. Chem. C, 2013, 1, 7842–7848 RSC.
- S. Altuntas and F. Buyukserin, J. Raman Spectrosc., 2018, 49, 1247–1256 CrossRef CAS.
- M. Blumenberg, BMC Genomics, 2013, 14, 1–20 CrossRef PubMed.
- A. Tanaka, T. Nagate and H. Matsuda, J. Vet. Med. Sci., 2005, 67, 909–913 CrossRef CAS.
- A. N. Dehkordi, F. M. Babaheydari, M. Chehelgerdi and S. R. Dehkordi, Stem Cell Res. Ther., 2019, 10, 1–20 CrossRef.
- D. Archana, J. Dutta and P. Dutta, Int. J. Biol. Macromol., 2013, 57, 193–203 CrossRef CAS PubMed.
- B. Saleh, H. K. Dhaliwal, R. Portillo-Lara, E. Shirzaei Sani, R. Abdi, M. M. Amiji and N. Annabi, Small, 2019, 15, 1–15 CrossRef.
- S. Patel, S. Srivastava, M. Singh and D. Singh, Int. J. Biol. Macromol., 2018, 107, 1888–1897 CrossRef CAS PubMed.
- C. Yang, L. Xu, Y. Zhou, X. M. Zhang, X. Huang, M. Wang, Y. Han, M. L. Zhai, S. C. Wei and J. Q. Li, Carbohydr. Polym., 2010, 82, 1297–1305 CrossRef CAS.
- B. Evranos, D. Aycan and N. Alemdar, Carbohydr. Polym., 2019, 222, 1–7 CrossRef.
- B. Dhandayuthapani, U. M. Krishnan and S. Sethuraman, J. Biomed. Mater. Res., Part B, 2010, 94B, 264–272 CAS.
- S. Chadwick, R. Heath and M. Shah, Indian J. Plast. Surg., 2012, 45, 403–411 CrossRef PubMed.
- S. P. Miguel, A. F. Moreira and I. J. Correia, Int. J. Biol. Macromol., 2019, 127, 460–475 CrossRef CAS.
- N. Benbettaieb, M. Kurek, S. Bornaz and F. Debeaufort, J. Sci. Food Agric., 2014, 94, 2409–2419 CrossRef CAS PubMed.
- S. Altuntas, H. K. Dhaliwal, N. Bassous, A. E. Radwan, P. Alpaslan, T. Webster, F. Buyukserin and M. Amiji, ACS Biomater. Sci. Eng., 2019, 5, 4311–4322 CrossRef CAS.
- H. C. Huang, H. Lin and M. C. Huang, Int. J. Mol. Med., 2017, 39, 595–602 CrossRef CAS.
- A. K. Dogan, M. Gumusderelioglu and E. Aksoz, J. Biomed. Mater. Res., Part B, 2005, 74, 504–510 Search PubMed.
- I. Permanadewi, A. C. Kumoro, D. H. Wardhani and N. Aryanti, J. Phys.: Conf. Ser., 2019, 1295, 1–8 CrossRef.
- T. Kopac, A. Lisac, R. Mravljak, A. Rucigaj, M. Krajnc and A. Podgornik, Polymers, 2021, 13, 1–12 CrossRef.
- F. Chen, F. F. Cao, Z. J. Su, L. Y. Li, A. B. Huang and H. Xu, Trop. J. Pharm. Res., 2014, 13, 689–696 CrossRef CAS.
- R. Maraschin, R. Bussi, A. Conz, L. Orlando, R. Pirovano and A. Nyska, Toxicol. Pathol., 1995, 23, 356–366 CrossRef CAS PubMed.
- S. Ramineni, C. Fowler, P. D. Fisher, L. Cunningham and D. Puleo, Biomed. Mater., 2015, 10, 1–13 Search PubMed.
- T. Hirobe, K. Hasegawa, R. Furuya, R. Fujiwara and K. Sato, J. Dermatol. Sci., 2013, 71, 45–57 CrossRef CAS.
- J. A. Buffey, A. G. Messenger, M. Taylor, A. T. T. Ashcroft, G. E. Westgate and S. Macneil, Br. J. Dermatol., 1994, 131, 836–842 CrossRef CAS.
- Y. Yamaguchi, S. Itami, H. Watabe, K. Yasumoto, Z. A. Abdel-Malek, T. Kubo, F. Rouzaud, A. Tanemura, K. Yoshikawa and V. J. Hearing, J. Cell Biol., 2004, 165, 275–285 CrossRef CAS.
- M. Arnold, E. A. Cavalcanti-Adam, R. Glass, J. Blummel, W. Eck, M. Kantlehner, H. Kessler and J. P. Spatz, ChemPhysChem, 2004, 5, 383–388 CrossRef CAS PubMed.
- M. Sieberblum, F. Sieber and K. M. Yamada, Exp. Cell Res., 1981, 133, 285–295 CrossRef CAS PubMed.
- B. H. Bin, D. K. Kim, N. H. Kim, E. J. Choi, J. Bhin, S. T. Kim, Y. S. Gho, A. Y. Lee, T. R. Lee and E. G. Cho, J. Invest. Dermatol., 2016, 136, 957–966 CrossRef CAS PubMed.
- M. Martínez-Ibáñez, N. S. Murthy, Y. Mao, J. Suay, M. Gurruchaga, I. Goñi and J. Kohn, J. Biomed. Mater. Res., Part B, 2018, 106, 1138–1147 CrossRef PubMed.
- P. P. Difiore, J. H. Pierce, T. P. Fleming, R. Hazan, A. Ullrich, C. R. King, J. Schlessinger and S. A. Aaronson, Cell, 1987, 51, 1063–1070 CrossRef CAS.
- Y. Mimura, H. Ihn, M. Jinnin, Y. Asano, K. Yamane and K. Tamaki, J. Invest. Dermatol., 2004, 122, 1390–1398 CrossRef CAS PubMed.
- S. Jimi, F. De Francesco, G. A. Ferraro, M. Riccio and S. Hara, J. Cell. Physiol., 2017, 232, 1225–1232 CrossRef CAS PubMed.
- J. L. Young, A. W. Holle and J. P. Spatz, Exp. Cell Res., 2016, 343, 3–6 CrossRef CAS.
- F. Han, Y. Dong, Z. Su, R. Yin, A. H. Song and S. M. Li, Int. J. Pharm., 2014, 476, 124–133 CrossRef CAS PubMed.
- M. Zhang, P. Wang, R. H. Luo, Y. Q. Wang, Z. Y. Li, Y. Q. Guo, Y. L. Yao, M. H. Li, T. T. Tao, W. W. Chen, J. B. Han, H. T. Liu, K. L. Cui, X. Zhang, Y. T. Zheng and J. H. Qin, Adv. Sci., 2021, 8, 1–14 Search PubMed.
- D. Kim, S. Y. Kim, S. K. Mun, S. Rhee and B. J. Kim, Int. J. Mol. Med., 2015, 35, 1017–1025 CrossRef CAS PubMed.
- J. Hardwicke, D. Schmaljohann, D. Boyce and D. Thomas, Surgeon, 2008, 6, 172–177 CrossRef CAS PubMed.
- S. Koike and K. Yamasaki, Int. J. Mol. Sci., 2020, 21, 1–18 Search PubMed.
- K. Nakamura, M. Yoshida, H. Uchiwa, Y. Kawa and M. Mizoguchi, Pigm. Cell Res., 2003, 16, 494–500 CrossRef CAS PubMed.
- R. C. Garcez, B. L. Teixeira, S. D. Schmitt, M. Alvarez-Silva and A. G. Trentin, Cell. Mol. Neurobiol., 2009, 29, 1087–1091 CrossRef CAS PubMed.
- Y. T. Cheng, Y. F. Li, S. Y. Huang, F. L. Yu, Y. Bei, Y. F. Zhang, J. Z. Tang, Y. D. Huang and Q. Xiang, Front. Bioeng. Biotechnol., 2020, 8, 1–12 CrossRef PubMed.
|
This journal is © The Royal Society of Chemistry 2023 |
Click here to see how this site uses Cookies. View our privacy policy here.