DOI:
10.1039/D2BM01535H
(Paper)
Biomater. Sci., 2023,
11, 96-107
Preparation and characterizations of an injectable and biodegradable high-strength iron-bearing brushite cement for bone repair and vertebral augmentation applications
Received
23rd September 2022
, Accepted 11th November 2022
First published on 28th November 2022
Abstract
Brushite cements have good osteoconductive and resorbable properties, but the low mechanical strength and poor injectability limit their clinical applications in load-bearing conditions and minimally invasive surgery. In this study, an injectable brushite cement that contains monocalcium phosphate monohydrate (MCPM) and β-tricalcium phosphate (β-TCP) as its solid phase and ammonium ferric citrate (AFC) solution as the aqueous medium was designed to have high mechanical strength. The optimized formulation achieved a compressive strength of 62.8 ± 7.2 MPa, which is above the previously reported values of hand-mixing brushite cements. The incorporation of AFC prolonged the setting times and greatly enhanced the injectability and degradation properties of the cements. In vitro and in vivo experiments demonstrated that the brushite cements exhibited good biocompatibility and bone regeneration capacity. The novel brushite cement is promising for bone healing in load-bearing applications.
1. Introduction
Orthopedic disease is a major global health issue that places tremendous economic burdens on society.1 With the increase of the aging population, the demand for bone grafts is rising rapidly.2 Currently, autologous bone grafts remain the gold standard for the repair of osseous defects.3 However, their clinical applications have been limited by the lack of donor sites and long operation time.4 The use of allografts has some disadvantages as well, such as potential disease transmission or bacterial infection.5 Therefore, synthetic bone grafts, with their unlimited availability, consistency, and no need for two subsequent surgeries, have attracted more attention during the past decades.6,7
Among the synthetic biomaterials, calcium phosphate cement (CPC) has shown great potential as a bone substitute material because of its excellent biocompatibility, osteoconductivity, and self-setting properties.8–10 Depending on the final products, CPC can be classified into two types: basic CPC with hydroxyapatite (Ca10(PO4)6(OH)2) as its end product and acidic CPC with brushite (CaHPO4·2H2O) as the final phase.11,12 In comparison with hydroxyapatite, brushite is a metastable phase with a higher solubility at physiological conditions.13–15 Previous studies have shown that brushite bioceramics are biocompatible, biodegradable, and osteoconductive in vitro and in vivo.16–20 They have been used as void fillers for craniofacial applications or cementitious materials for implants fixation.21–23 Brushite cement, which is self-setting and injectable, was first reported by Mirtchi and Lemaitre in 1989.24 The starting materials of brushite cements usually consist of monocalcium phosphate monohydrate (MCPM) and β-tricalcium phosphate (β-TCP) as the solid phase.25 After mixing the solid with aqueous media, the pastes can be injected into bone defects and harden in situ.26 The acidic reaction medium is essential for the formation of brushite cement, and the setting pH is usually between 3.5 and 6.5.27,28
Despite all these advantages, the main drawbacks of brushite cement lie in its inferior mechanical strength and poor injectability, which hinder its further applications in load-bearing conditions and minimally invasive surgery. Many attempts have been made to overcome the weakness of brushite cements. One effective way is through the incorporation of inorganic or polymeric additives. These additives affect the hardening process by regulating crystal nucleation and growth, resulting in cements with improved working and mechanical properties.29 Citric acid, pyrophosphate, tartaric acid and glycolic acid are among the most commonly used additives for brushite cements.30–32 They can be adsorbed on the surface of calcium phosphate crystals, which change surface charge and lead to mutual repulsion of cement particles. For example, a cement with a compressive strength of 32 MPa has been obtained by using L-(+)-tartaric acid as the additive.33 In another study, brushite cement with a compressive strength of 57 MPa was prepared with the addition of disodium dihydrogen pyrophosphate and citric acid.13 However, a low liquid-to-powder ratio (0.22 mL g−1) was required to obtain a cement with high mechanical strength, at the cost of decreased handling property and injectability.
Ion substitution has been found to be efficient in enhancing the chemical–physical and biological properties of calcium phosphate cement. For example, the presence of Sr2+ and Mg2+ inhibits the growth of brushite crystals and therefore can prolong the setting time of the cements.34–36 Sr2+ is reported to accelerate bone healing by inhibiting osteoclast differentiation and promoting osteoblast bone-forming activity.37 More recently, Fe3+ has attracted increasing interest as they have shown the functions of stimulating angiogenic differentiation of endothelial cells and improving angiogenic properties of bone repairing materials.38 Sr/Fe co-doped HAp nanomaterials exhibit good biocompatibility and improved osteogenic properties.39 Moreover, Fe3+ was found to have a positive effect on the mechanical strength of brushite cements, probably due to the smaller particle size and reduced porosity.21 Although the compressive strength of the cement increased from 11.5 MPa to 24.5 MPa when Fe3+ was added as a dopant, the mechanical strength was still insufficient for load-bearing conditions. Therefore, brushite cements with high mechanical strength and good injectability remain to be developed.
In this study, an injectable iron-bearing brushite cement with ultra-high mechanical strength was fabricated in the presence of ammonium ferric citrate (AFC). The compressive strength of the cement (62.8 ± 7.2 MPa) was above the previously reported values of hand-mixing brushite cements. Moreover, it showed a final setting time of 13 min and good injectability. The hydration products, in vitro degradation property, biocompatibility, and ion release of the cements were evaluated. Finally, the in vitro vertebral augmentation property and in vivo bone repairing property were investigated (Scheme 1).
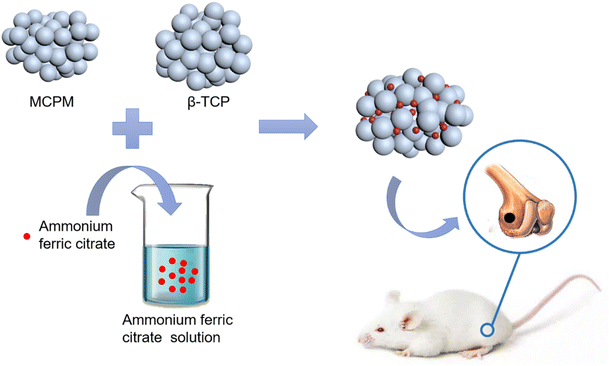 |
| Scheme 1 . Schematic illustration of the brushite cement preparation and its implantation in a rat femur defect model. | |
2. Materials and methods
2.1 Preparation of brushite cements
MCPM (Scharlu, Spain) was sieved to ensure the particle size was smaller than 75 μm. MCPM and β-TCP (Dingan, China) powders with various mass ratios (60
:
40, 55
:
45, 50
:
50, 45
:
55, 40
:
60, and 35
:
65) were mixed mechanically for 10 min. The liquid phases of the cements were prepared from solutions of AFC with various concentrations (0.15 M, 0.20 M, 0.26 M, 0.31 M, and 0.37 M). The solid and liquid phases were manually mixed on a glass slab for 2 min, then put into polydimethylsiloxane (PDMS, Dow Corning, USA) molds. Subsequently, samples were left at room temperature for 2 h and then incubated in PBS at 37 °C for a predetermined time.
2.2 Setting time measurement
The powders were mixed with aqueous solutions for 2 min before being put in PDMS rings with the diameter of (10 mm and the height of 5 mm). The setting times of the specimens were measured using the Gillmore needle method (ASTM C266-89) at room temperature (22 ± 1 °C). The initial setting time and final setting time were determined as the moment when the light needle (113.4 g in weight and 2.12 mm in diameter) and heavy needle (453.6 g in weight and 1.06 mm in diameter) failed to mark the surface of the cement. Three samples per group were used.
2.3 Mechanical property test
Cylindrical specimens (6 mm in diameter and 13 mm in height) were prepared using PDMS molds. After incubating in PBS at 37 °C for a predetermined time, the specimens were taken out of PBS and polished with 1000-grit paper. A universal testing machine (Shanghai Hengyi, China) was used to measure the compressive strength of the cements. The crosshead speed was set as 1 mm min−1. Five samples were used for each group.
2.4 Injectability test
A quantitative evaluation of injectability was conducted using a 2.5 mL syringe with a nozzle diameter of 2.0 mm at room temperature. Certain amount of cement pastes were added into the syringe and loaded on the universal testing machine with the loading speed of 5 mm min−1 until the force reached 50 N. The weight of the initial pastes (w0) and that of expelled from the syringe (wt) were measured. Each test was repeated at least three times. The injectability was calculated using the equation below.
Injectability (%) = wt/w0 × 100%. |
2.5 Ion release test
To prepare the extracts, cement specimens were prepared and sterilized by irradiation (20 kGy). After washing three times for 5 minutes each with PBS, samples were incubated for 24 hours in serum-free α-MEM (0.2 g mL−1) in a standard cell culture incubator. The extracts were obtained by filtering the supernatant with a 0.22 μm filter. The levels of atomic Ca2+ at 396.847 nm, P5+ at 213.618 nm, and Fe3+ at 238.204 nm released in extracts were analyzed using inductively coupled plasma atomic emission spectrometer (ICP, Vista-MPX, Agilent, USA). Three samples were measured for each group.
2.6
In vitro degradation test
In vitro degradation of the brushite cements was investigated by immersing the samples in PBS and the degradation was characterized by its residual weight ratio. After storage for 1, 3, 7, 14, 28, and 56 d at 37 °C, samples were washed with deionized water and dried in a vacuum oven for 48 h. The remaining mass of specimens was calculated with the following equation: remaining mass (%) = mw/m0 × 100%, where m0 is the initial mass of the sample and mw is the mass of the dried sample. Three samples per group were used.
2.7 Scanning electron microscope (SEM) measurement
Cement samples were ground to fine-grained powder and sputtered with a platinum layer to improve sample conductivity. A scanning electron microscope (SEM, Hitachi Limited, Japan) was used to observe the morphology of the powder.
2.8 X-ray diffractometer
The phase composition was examined using an X-ray diffractometer (XRD, PANalytical, Netherlands) equipped with a copper source. XRD analyses were performed with a 2θ range of 5–70°, with steps of 0.02° and 0.25 s for per step.
2.9 Cell culture
After being harvested from rats of 6 weeks old, bone marrow mesenchymal stem cells (BMSCs) were cultured in α-MEM supplemented with 10% FBS (HyClone, USA) and 1% penicillin–streptomycin (Gibco, USA) at 37 °C at 5% CO2. The biocompatibility of brushite cements was evaluated using cement extracts. The preparation process is similar to that of the ion release test, as mentioned above. Filtered extracts were then supplemented with 10% FBS and 1% penicillin–streptomycin to obtain the extract medium. BMSCs were seeded onto 24 well plates (3 × 103 cells per well) in α-MEM. After culturing for 24 h, the α-MEM was replaced with extract medium. Cell proliferation was evaluated using the Cell Counting Kit-8 assay (CCK-8, Kumamoto, Japan). 10% CCK-8 solution was added to each sample and incubated at 37 °C for 2 h. The absorbance was measured at a wavelength of 450 nm using a microplate reader (BioTek instruments, USA).
2.10
In vitro vertebral augment test
Adult sheep spine specimens (L2–L6) were obtained from a local abattoir. After removing soft tissues, a hole (6 mm in diameter) was drilled across the middle of the specimen. Polymethyl methacrylate (PMMA) was applied to cover the top and bottom of the vertebrae, keeping the vertebra upright. The brushite cement was then injected into the drilled vertebrae specimens. The samples were soaked in PBS for 1 day at 37 °C before test. A compression speed of 1 mm min−1 was used and the maximum load values (N) were recorded.
2.11 Animal studies
All procedures followed the NIH Guide for the Care and Use of Laboratory Animals. The experiments were approved by the Institutional Animal Care and Use Committee of Soochow University. Male rats (10 weeks old) were used for implantation of the cements. Rats were anesthetized with pentobarbital sodium and a cylindrical defect (3 mm in diameter and 3 mm in depth) was drilled on the condyles of the femur using a micro bone drill. Cements were injected into the defect sites and wounds were carefully sutured. Animals were sacrificed and the femur was obtained for analysis at 4 or 8 weeks after implantation.
2.12 Microcomputed tomography and histological analysis
The removed rat femurs were fixed in 10% neutral buffered formalin for 24 h at room temperature. The samples were analyzed using a microcomputed tomography (micro-CT) imaging system (SkyScan 1176, SkyScan, Aartselaar, Belgium). Scan data were reconstructed using the Skyscan NRecon program and analyzed using Dataviewer and SkyScan CTAn. Three-dimensional rendering was prepared from the analyzed data using the Mimics software.
Before dehydrating with graded ethanol, each sample was decalcified with 12% ethylenediaminetetraacetic acid (EDTA) for 4 weeks. Residual alcohol was removed by immersion in xylene and the tissue sample was embedded in paraffin blocks. Tissue slices (6 μm thick) were cut using a microtome (LEICA, Germany) and hematoxylin and eosin (H&E) used for staining. The sections were observed using a bright field microscope (Zeiss, Germany).
2.13 Statistical analysis
Quantitative data were expressed as the mean ± standard deviation. One-way analysis of variance (ANOVA) followed by Tukey post hoc comparison (GraphPad Software 7.0, CA, USA) was used for statistical analysis. A value of p < 0.05 denotes statistically significant difference.
3. Results
3.1 Characterization of raw materials
The phase compositions of the raw materials were characterized using XRD, as shown in Fig. 1A. The raw materials exhibited the characteristic peaks of β-TCP and MCPM, respectively, confirming the purity of the raw materials. β-TCP showed flake-like morphology (Fig. 1B), and MCPM had irregular micro-sized particles (Fig. 1C). The particle sizes of β-TCP and MCPM were around 7 μm and 20 μm, respectively, on average (Fig. 1D and E).
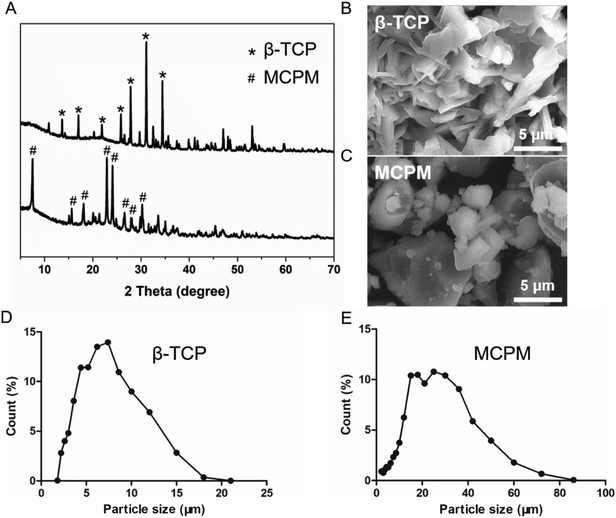 |
| Fig. 1 XRD patterns (A) and SEM photographs of β-TCP (B) and MCPM (C) and size distributions of β-TCP (D) and MCPM (E). | |
3.2 Setting time
Both initial and final setting time were prolonged with increasing concentrations of AFC (Fig. 2). The initial setting times of brushite cements with 0.15 M, 0.20 M, 0.26 M, 0.31 M, and 0.37 M AFC solutions were 5.2 ± 0.7 min, 13.8 ± 0.3 min, 13.0 ± 0.5 min, 33.8 ± 2.2 min, 36.0 ± 1.5 min, respectively (Fig. 2A). The final setting times of brushite cements with 0.15 M, 0.20 M, 0.26 M, 0.31 M, and 0.37 M AFC solutions were 7.0 ± 0.5 min, 20.0 ± 1.0 min, 21.2 ± 2.2 min, 55.5 ± 2.5 min, and 59.2 ± 4.3 min, respectively (Fig. 2B).
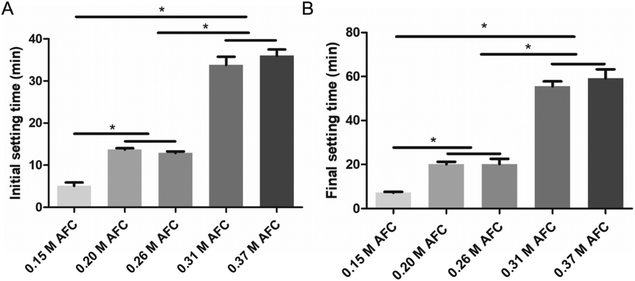 |
| Fig. 2 Initial setting time (A) and final setting time (B) of the brushite cements with different concentrations of AFC solutions (* indicates p < 0.05). | |
3.3 Mechanical properties
The MCPM
:
β-TCP mass ratio and concentration of AFC solutions had a substantial impact on the compressive strength of the brushite cements (Fig. 3). When the mass ratio of MCPM and β-TCP was 60
:
40, the compressive strength of the cement was only 12.4 ± 1.3 MPa. The compressive strength increased with the decrease of MCPM
:
β-TCP mass ratio and reached the highest (62.8 ± 7.2 MPa) when the mass ratio was 50
:
50 (Fig. 3A). Further decreasing the mass ratio resulted in the decline of the compressive strength. The influence of the AFC concentrations on the compressive strength of the cements was evaluated (Fig. 3B). The samples with 0.15 M AFC showed a compressive strength of 32.7 ± 12.2 MPa. The brushite cements with 0.20 M AFC, 0.26 M AFC, 0.31M AFC, and 0.37 M AFC solutions showed enhanced compressive strength (57.3 ± 6.6 MPa, 62.8 ± 7.2 MPa, 57.2 ± 4.1 MPa, and 52.4 ± 5.7 MPa), and there were no significant differences among the groups. Soaking in PBS solutions did not influence the compressive strength of brushite cements (Fig. 4).
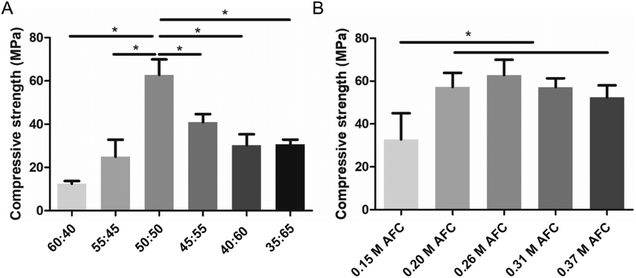 |
| Fig. 3 Compressive strength of the brushite cements (0.26 M AFC) with different MCPM : β-TCP ratios (A) and AFC solutions of different concentrations (B) after soaking in PBS for 1 day (* indicates p < 0.05). | |
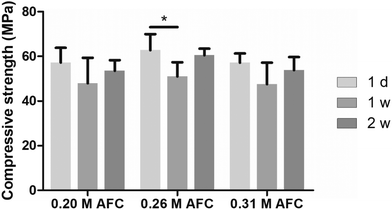 |
| Fig. 4 Compressive strength of the brushite cements with different concentrations of AFC solutions after soaking in PBS for different periods. | |
3.4 Structural analysis of the cements
Morphology of the brushite cements with different concentrations of AFC was observed through SEM. As shown in Fig. 5, most of the resultant particles were irregular, with particle size less than 1 μm. Variation of AFC concentration showed no effect on the microstructure of brushite cements. XRD patterns confirmed that the final products were brushite in all groups (Fig. 6).
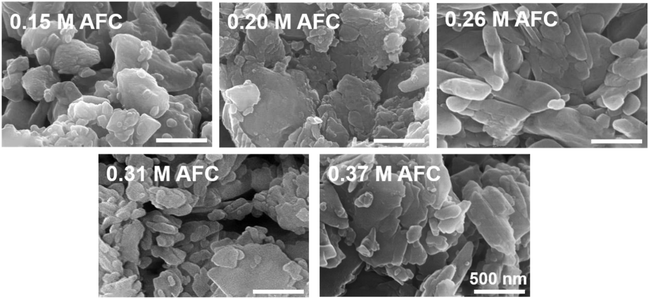 |
| Fig. 5 SEM images of the brushite cements with different concentrations of AFC solutions after hydration for 24 h. | |
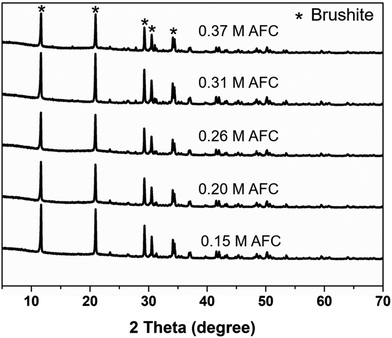 |
| Fig. 6 XRD patterns of the brushite cements with different concentrations of AFC solutions after hydration for 1 day. | |
3.5 Injectability
The injectability of brushite cements prepared with AFC solutions is shown in Fig. 7. The results showed that the presence of AFC greatly increased the injectability of brushite cements. The injectability of brushite cements prepared with 0.15 M was only 2.9 ± 0.3%. The injectability increased to 70.9 ± 2.8% when 0.20 M AFC was added. Further increase in the AFC concentration did not influence the injectability of brushite cement.
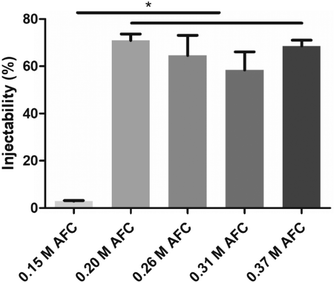 |
| Fig. 7 Injectability of the brushite cements with different concentrations of AFC solutions. The measurements were conducted after preparing for 2 min (* indicates p < 0.05). | |
3.6
In vitro degradation
The in vitro degradation of the cements was evaluated by immersing the cements in PBS for various periods (Fig. 8). All the samples gradually degraded during storage. Brushite cements prepared with high concentrations of AFC solutions showed faster degradation. The remaining mass of brushite cements with 0.15 M, 0.20 M, 0.26 M, 0.31 M and 0.37 M AFC solutions was 84.57 ± 0.33%, 84.03 ± 1.13%, 79.28 ± 0.78%, 72.41 ± 3.49%, and 64.59 ± 3.15% after storage in PBS for 8 weeks, respectively.
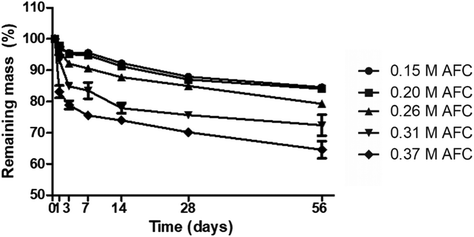 |
| Fig. 8 Degradation of the brushite cements with different concentrations of AFC solutions after hydration for 56 days. | |
3.7 Cell culture
BMSCs were cultured with cement extracts either used as obtained (100%) or diluted (50% and 20%). The cell proliferation test was performed by the CCK-8 assay. As shown in Fig. 9, brushite cements prepared with 0.15 M AFC and 0.20 M AFC solutions showed good biocompatibility regardless of their dilutions; 100% extracts of brushite cements with increasing concentrations of AFC (0.26 M, 0.31 M, and 0.37 M) resulted in a decrease in cell numbers (Fig. 9A). In the case of using the 50% extract, the cell number dropped when using 0.31 M and 0.37 M AFC. Regardless of the dilution, the cell number dropped when cells were incubated with an extract of 0.37 M of the AFC group.
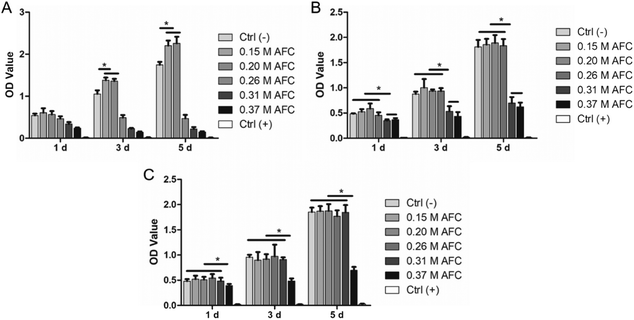 |
| Fig. 9 The proliferation of BMSCs cultured in medium with cement extracts (A), 2-fold diluted extracts (B), 5-fold diluted extracts (C) (* indicates p < 0.05). | |
3.8 Ion release
The concentrations of atomic Ca2+, Fe3+, and P5+ in cement extracts after soaking 24 h were systematically investigated. In general, all the ions showed an increase with increasing concentrations of AFC (Fig. 10). This is in accordance with the results of the degradation test (Fig. 8), showing that the cements degraded more rapidly with higher concentrations of AFC. Cements with 0.15 M AFC showed the lowest concentration of Ca2+ (747.7 ± 49.5 mg L−1) and P5+ (1959.6 ± 87.5 mg L−1) (Fig. 10A and B). The ion concentrations gradually increased with the increase of AFC concentration and reached the highest for cements with 0.37 M AFC (2194.3 ± 167.0 mg L−1 Ca2+, 5091.7 ± 207.1 mg L−1 P5+). The cement prepared with 0.15 M AFC and 0.20 M AFC showed low concentrations of Fe3+ (0.7 ± 0.1 mg L−1 for 0.15 M AFC, 0.7 ± 0.2 mg L−1 for 0.20 M AFC) (Fig. 10C). However, further increasing the concentration of AFC resulted in the rapid increase of Fe3+. The highest amount of Fe3+ was observed in cement with 0.37 M AFC (16.8 ± 3.9 mg L−1).
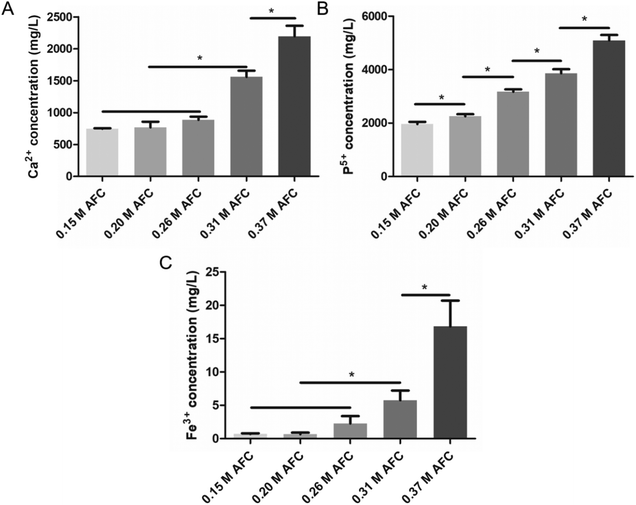 |
| Fig. 10 Concentrations of Ca2+ (A), Fe3+ (B), and P5+ (C) in cement extracts after soaking for 24 h tested by ICP-AES. * indicates p < 0.05. | |
3.9
In vitro vertebral augmentation
The mechanical properties of CPC-filled sheep vertebrae are shown in Fig. 11. Compared with those of drilled vertebrae, both the compressive strength and stiffness of CPC-filled vertebrae increased. The highest compressive strength was observed in 0.20 M AFC (5130 ± 226 N) and 0.26 M AFC (5209 ± 323 N) groups, in comparison to 2389 ± 113 N in the control group. The cements with 0.20 M AFC and 0.26 M AFC showed stiffness of 1089 ± 102 N mm−1 (0.20 M AFC) and 1104 ± 84 N mm−1 (0.26 M AFC) respectively, compared to that of 689 ± 103 N mm−1 in control group.
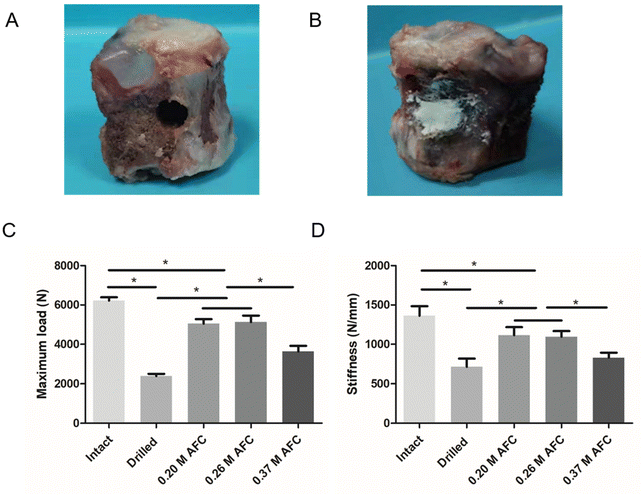 |
| Fig. 11 Schematic diagram of the vertebral specimens with defects (A), vertebral specimens filled with brushite cements (B). Mechanical strength (C) and stiffness (D) of vertebral specimens (* indicates p < 0.05). | |
3.10 Micro-CT analysis
The rat femoral condyle defects model was used to investigate the repair effects of the brushite cements. Implants after 3D reconstruction are shown in Fig. 12. Red and gray represent new bone and residual implanted materials, respectively. Among all the groups, new bone formation was observed on the surface of implanted materials. The defects filled with brushite cements exhibited a larger amount of new bone, and only a small amount of new bone was observed along the defect area in the control group. After 8 weeks, all the groups showed a larger amount of new bone formation (Fig. 12). Bone volume/total volume (BV/TV) of the new bone after implantation for 4 and 8 weeks was calculated for each sample (Fig. 13). Cements with AFC showed higher BV/TV than the control group. At 4 weeks and 8 weeks, BV/TV values of cements prepared with AFC were similar, regardless of the AFC concentration.
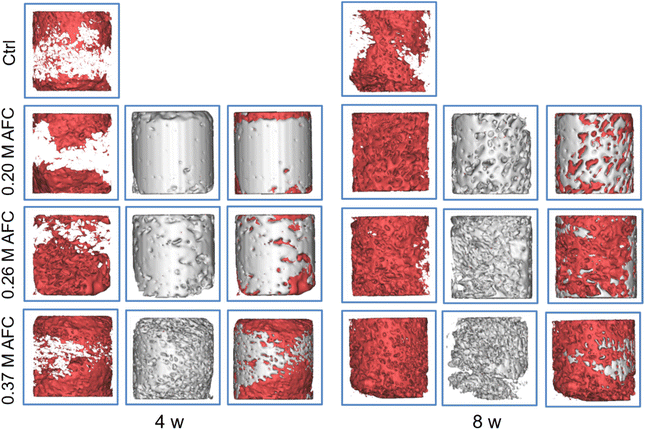 |
| Fig. 12 CT scanning images along with the radial directions of grafts in a rat femur defect model after implantation for 4 and 8 weeks. | |
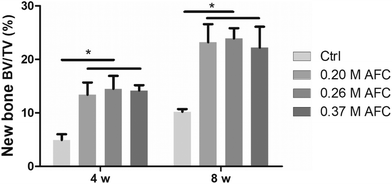 |
| Fig. 13 Quantitative analysis of new bone after implantation for 4 and 8 weeks (* indicates p < 0.05). | |
3.11 Histological staining
H&E staining of the sections is shown in Fig. 14. After implantation for 8 weeks, new bone regeneration was observed at the periphery of the defect sites in groups filled with cements. Compared with the control group, the bone defect filled with brushite cements showed more new bone formation. The results indicated that the brushite cements promoted bone formation around the implants.
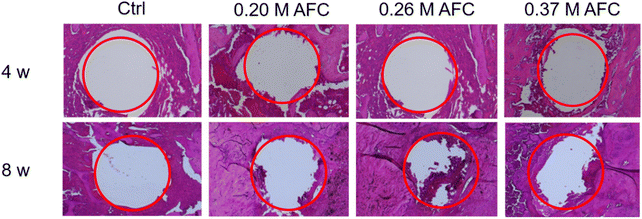 |
| Fig. 14 Representative histological sections of brushite cements for four and eight weeks post-implantation in rat femurs. | |
4. Discussion
In this study, a brushite cement with good injectability and enhanced mechanical properties are prepared. Brushite cements have the advantage of being resorbable in comparison to apatite cements.20,40 However, their shortcomings such as fast setting, inferior mechanical strength, and poor injectability limit the scope of their applications.41 In the orthopedic clinic, appropriate setting time is required to facilitate the operation. It is suggested that the initial setting time should be 4–8 minutes and the final setting time should be 10–15 minutes for vertebral augmentation. Cement with 0.15 M AFC showed an initial setting time of 5 min and a final setting time of 7 min (Fig. 2). The final setting time of brushite cements can be regulated from 7.0 ± 0.5 to 59.2 ± 4.3 min by adjusting the concentrations of AFC solutions. Despite of the various setting times, the concentrations of AFC solutions have no significant effect on the microstructure and phase composition of brushite cements (Fig. 5 and 6). All the specimens mainly presented lamellar crystals and the XRD patterns showed that the main component of all cements is brushite. Previous studies have shown that injectability is also essential for the application of brushite cements, specifically in minimally invasive surgery such as percutaneous kyphoplasty. As is shown in Fig. 7, the injectability of brushite cements is enhanced by introducing AFC, the value of which reached 70.9 ± 2.8% when 0.20 M AFC was incorporated. Another factor that limits the application of brushite cements lies in their low mechanical strength.33 Many studies have been conducted to improve the mechanical strength of brushite cements. Tamimi et al.,42found that the combination of collagen with citric acid exhibited an interesting synergistic effect on the compressive strength, and the novel brushite cements showed enhanced compressive strength (48.9 ± 5.9 MPa). Hofmann et al.,13prepared a high-strength brushite cement (52 MPa when wet and uncompacted) by adjusting the particle size, size distribution, and concentration of citric acid. Luo et al.,29prepared a brushite cement with high compressive strength (57 MPa) with the addition of disodium dihydrogen pyrophosphate and citric acid. In this study, we have shown that the AFC is a key contributor to the mechanical property of the cements (Fig. 3). With the incorporation of 0.26 M AFC solution, a cement with compressive strength as high as 62.8 MPa has been obtained, which is higher than the previously reported values of hand-mixed brushite cements.
Ideally, an implant should degrade gradually to allow new bone formation.43,44 The brushite cements are more soluble than apatite cements and are quickly resorbed in vivo.18 In our study, it is interesting to note that the degradation rate of brushite cements increases with the increase of AFC concentration (Fig. 8). Moreover, the compressive strength of the brushite cements is retained after soaking in PBS for different periods (Fig. 4). The absorbable feature of the novel cement, combined with its high mechanical strength, is beneficial for its application in load-bearing conditions. Cell cytotoxicity assays verified that brushite cements prepared with 0.15 M and 0.20 M AFC show good biocompatibility (Fig. 9). Further increasing AFC concentration results in inhibition of proliferation of BMSCs. This is probably due to the higher release of Fe3+. As shown in Fig. 10, the Fe3+ concentration increases from 0.7 mg L−1 to 2.6 mg L−1 when the concentration of AFC is increased from 0. 20 M to 0.26 M, which might be responsible for the toxicity of cements at high concentrations of AFC. The in vivo experiment was conducted to evaluate the bone repairing properties of the prepared cements (Fig. 12–14). The H&E staining and micro-CT results demonstrate that the bone defects implanted with the brushite cements achieved superior bone regeneration to that of the control group. However, no significant difference was observed among the groups with different AFC concentrations. This work presents an option to enhance the mechanical property of brushite cements. Many topics such as particle size of the raw materials and synthetic effect of AFC with other additives still need to be investigated. More exploration is needed to develop the therapeutic effect to achieve clinical translation in the future.
5. Conclusion
An injectable iron-bearing brushite cement with ultra-high mechanical strength was prepared in the presence of ammonium ferric citrate. The addition of ammonium ferric citrate prolonged the initial and final setting times, improved the mechanical properties and injectability, and accelerated the degradation of brushite cements. In vitro studies have demonstrated that the novel brushite cements exhibit good biocompatibility and can restore the mechanical function of impaired vertebra. In vivo experiments have shown that the brushite cements possess excellent bone repairing ability, indicating their great potential for load-bearing applications in the orthopedic field.
Conflicts of interest
There are no conflicts to declare.
Acknowledgements
The authors are grateful for the funding support from the National Key R&D Program of China (2020YFC1107401), National Natural Science Foundation of China (82002275, 81925027), Natural Science Foundation of the Jiangsu Higher Education Institutions of China (20KJB430041), and the Priority Academic Program Development of Jiangsu Higher Education Institutions.
References
- J. Yao, Z. Liu, W. Ma, W. Dong, Y. Wang and H. Zhang,
et al., Three-Dimensional Coating of SF/PLGA Coaxial Nanofiber Membranes on Surfaces of Calcium Phosphate Cement for Enhanced Bone Regeneration, ACS Biomater. Sci. Eng., 2020, 6(5), 2970–2984 CrossRef CAS PubMed.
- Y. Peng, J. Li, H. Lin, S. Tian, S. Liu and F. Pu,
et al., Endogenous repair theory enriches construction strategies for orthopaedic biomaterials: a narrative review, Biomater. Transl. Med., 2021, 2(4), 343–360 Search PubMed.
- Y. Zhang, Y. Jiang, D. Zou, B. Yuan, H. Z. Ke and W. Li, Therapeutics for enhancement of spinal fusion: A mini review, J. Orthop. Translat., 2021, 31, 73–79 CrossRef.
- Y. Wang, W. Zhang and Q. Yao, Copper-based biomaterials for bone and cartilage tissue engineering, J. Orthop. Translat., 2021, 29, 60–71 CrossRef.
- E. Garcia-Gareta, M. J. Coathup and G. W. Blunn, Osteoinduction of bone grafting materials for bone repair and regeneration, Bone, 2015, 81, 112–121 CrossRef CAS PubMed.
- J. Zhang, W. Liu, V. Schnitzler, F. Tancret and J. M. Bouler, Calcium phosphate cements for bone substitution: chemistry, handling and mechanical properties, Acta Biomater., 2014, 10(3), 1035–1049 CrossRef CAS.
- F. Zhao, Z. Yang, L. Liu, D. Chen, L. Shao and X. Chen,
et al., Design and evaluation of a novel sub-scaffold dental implant system based on the osteoinduction of micro-nano bioactive glass, Biomater. Transl. Med., 2020, 1(1), 82–88 Search PubMed.
- L. Cui, S. Xiang, D. Chen, R. Fu, X. Zhang and J. Chen,
et al., A novel tissue-engineered bone graft composed of silicon-substituted calcium phosphate, autogenous fine particulate bone powder and BMSCs promotes posterolateral spinal fusion in rabbits, J. Orthop. Translat., 2021, 26, 151–161 CrossRef PubMed.
- Y. Chen, Z. Sun, Y. Li and Y. Hong, Osteogenic commitment of mesenchymal stem cells in apatite nanorod-aligned ceramics, ACS Appl. Mater. Interfaces, 2014, 6(24), 21886–21893 CrossRef CAS.
- J. M. Bouler, P. Pilet, O. Gauthier and E. Verron, Biphasic calcium phosphate ceramics for bone reconstruction: A review of biological response, Acta Biomater., 2017, 53, 1–12 CrossRef CAS PubMed.
- J. Luo, H. Engqvist and C. Persson, A ready-to-use acidic, brushite-forming calcium phosphate cement, Acta Biomater., 2018, 81, 304–314 CrossRef CAS PubMed.
- A. Cahyanto, K. Tsuru and K. Ishikawa, Effect of setting atmosphere on apatite cement resorption: An in vitro and in vivo study, J. Mech. Behav. Biomed. Mater., 2018, 88, 463–469 CrossRef CAS PubMed.
- M. P. Hofmann, A. R. Mohammed, Y. Perrie, U. Gbureck and J. E. Barralet, High-strength resorbable brushite bone cement with controlled drug-releasing capabilities, Acta Biomater., 2009, 5(1), 43–49 CrossRef CAS.
- M. Bohner and U. Gbureck, Thermal reactions of brushite cements, J. Biomed. Mater. Res., Part B, 2008, 84(2), 375–385 CrossRef CAS PubMed.
- S. Chen, M. Krumova, H. Cölfen and E. V. Sturm, Synthesis of Fiber-like Monetite without Organic Additives and Its Transformation to Hydroxyapatite, Chem. Mater., 2019, 31(5), 1543–1551 CrossRef CAS.
- Z. Xia, L. M. Grover, Y. Huang, I. E. Adamopoulos, U. Gbureck and J. T. Triffitt,
et al., In vitro biodegradation of three brushite calcium phosphate cements by a macrophage cell-line, Biomaterials, 2006, 27(26), 4557–4565 CrossRef CAS.
- F. Theiss, D. Apelt, B. Brand, A. Kutter, K. Zlinszky and M. Bohner,
et al., Biocompatibility and resorption of a brushite calcium phosphate cement, Biomaterials, 2005, 26(21), 4383–4394 CrossRef CAS.
- D. Apelt, F. Theiss, A. O. El-Warrak, K. Zlinszky, R. Bettschart-Wolfisberger and M. Bohner,
et al., In vivo behavior of three different injectable hydraulic calcium phosphate cements, Biomaterials, 2004, 25(7–8), 1439–1451 CrossRef CAS PubMed.
- M. Bohner, Calcium orthophosphates in medicine: from ceramics to calcium phosphate cements, Injury, 2000, 31(Suppl 4), 37–47 CrossRef.
- M. Bohner, F. Theiss, D. Apelt, W. Hirsiger, R. Houriet and G. Rizzoli,
et al., Compositional changes of a dicalcium phosphate dihydrate cement after implantation in sheep, Biomaterials, 2003, 24(20), 3463–3474 CrossRef CAS.
- F. T. Marino, J. Torres, I. Tresguerres, L. B. Jerez and EL. Cabarcos, Vertical bone augmentation with granulated brushite cement set in glycolic acid, J Biomed Mater Res A, 2007, 81(1), 93–102 CrossRef PubMed.
- J. M. Kuemmerle, A. Oberle, C. Oechslin, M. Bohner, C. Frei and I. Boecken,
et al., Assessment of the suitability of a new brushite calcium phosphate cement for cranioplasty - an experimental study in sheep, J. Craniofac. Surg., 2005, 33(1), 37–44 CrossRef.
- G. Schneider, K. Blechschmidt, D. Linde, P. Litschko, T. Korbs and E. Beleites, Bone regeneration with glass ceramic implants and calcium phosphate cements in a rabbit cranial defect model, J. Mater. Sci. Mater. Med., 2010, 21(10), 2853–2859 CrossRef CAS.
- A. A. Mirtchi, J. Lemaitre and N. Terao, Calcium phosphate cements: study of the beta-tricalcium phosphate-monocalcium phosphate system, Biomaterials, 1989, 10(7), 475–480 CrossRef CAS PubMed.
- R. Jayasree, T. S. S. Kumar, R. Venkateswari, R. P. Nankar and M. Doble, Eggshell derived brushite bone cement with minimal inflammatory response and higher osteoconductive potential, J. Mater. Sci. Mater. Med., 2019, 30(10), 113 CrossRef CAS.
- K. Hurle, J. M. Oliveira, R. L. Reis, S. Pina and F. Goetz-Neunhoeffer, Ion-doped Brushite Cements for Bone Regeneration, Acta Biomater., 2021, 123, 51–71 CrossRef CAS PubMed.
- K. A. Shariff, K. Tsuru and K. Ishikawa, Fabrication of interconnected pore forming alpha-tricalcium phosphate foam granules cement, J. Biomater. Appl., 2016, 30(6), 838–845 CrossRef CAS PubMed.
- S. Chen, K. Grandfield, S. Yu, H. Engqvist and W. Xia, Synthesis of calcium phosphate crystals with thin nacreous structure, CrystEngComm, 2016, 18(6), 1064–1069 RSC.
- J. Luo, I. Ajaxon, M. P. Ginebra, H. Engqvist and C. Persson, Compressive, diametral tensile and biaxial flexural strength of cutting-edge calcium phosphate cements, J. Mech. Behav. Biomed. Mater., 2016, 60, 617–627 CrossRef CAS.
- J. L. Giocondi, B. S. El-Dasher, G. H. Nancollas and C. A. Orme, Molecular mechanisms of crystallization impacting calcium phosphate cements, Philos. Trans. R. Soc., A, 2010, 368(1917), 1937–1961 CrossRef CAS.
- M. Bohner, J. Lemaitre and T. A. Ring, Effects of sulfate, pyrophosphate, and citrate ions on the physicochemical properties of cements made of beta-tricalcium phosphate-phosphoric acid-water mixtures, J. Am. Ceram. Soc., 1996, 79(6), 1427–1434 CrossRef CAS.
- F. T. Marino, J. Torres, M. Hamdan and C. R. Rodriguez, Cabarcos EL. Advantages of using glycolic acid as a retardant in a brushite forming cement, J. Biomed. Mater. Res., Part B, 2007, 83(2), 571–579 CrossRef.
- H. Moussa, W. Jiang, A. Alsheghri, A. Mansour, A. E. Hadad and H. Pan,
et al., High strength brushite bioceramics obtained by selective regulation of crystal growth with chiral biomolecules, Acta Biomater., 2020, 106, 351–359 CrossRef CAS.
- S. Pina, P. M. Torres, F. Goetz-Neunhoeffer, J. Neubauer and J. M. Ferreira, Newly developed Sr-substituted alpha-TCP bone cements, Acta Biomater., 2010, 6(3), 928–935 CrossRef CAS PubMed.
- K. J. Lilley, U. Gbureck, J. C. Knowles, D. F. Farrar and J. E. Barralet, Cement from magnesium substituted hydroxyapatite, J. Mater. Sci. Mater. Med., 2005, 16(5), 455–460 CrossRef CAS.
- U. Klammert, T. Reuther, M. Blank, I. Reske, J. E. Barralet and L. M. Grover,
et al., Phase composition, mechanical performance and in vitro biocompatibility of hydraulic setting calcium magnesium phosphate cement, Acta Biomater., 2010, 6(4), 1529–1535 CrossRef CAS.
- P. M. C. Torres, A. Marote, A. R. Cerqueira, A. J. Calado, J. C. C. Abrantes and S. Olhero,
et al., Injectable MnSr-doped brushite bone cements with improved biological performance, J. Mater. Chem. B, 2017, 5(15), 2775–2787 RSC.
- H. S. Shi, S. Y. Yang, S. H. Zeng, X. Liu, J. Zhang and J. Zhangb,
et al., Enhanced angiogenesis of biodegradable iron-doped octacalcium phosphate/poly(lactic-co-glycolic acid) scaffold for potential cancerous bone regeneration, Appl. Mater. Today, 2019, 15, 100–114 CrossRef.
- I. Ullah, W. Zhang, L. Yang, M. W. Ullah, O. M. Atta and S. Khan,
et al., Impact of structural features of Sr/Fe co-doped HAp on the osteoblast proliferation and osteogenic differentiation for its application as a bone substitute, Mater. Sci. Eng., C, 2020, 110, 110633 CrossRef CAS PubMed.
- F. Tamimi, Z. Sheikh and J. Barralet, Dicalcium phosphate cements: brushite and monetite, Acta Biomater., 2012, 8(2), 474–487 CrossRef CAS PubMed.
- J. Engstrand, C. Persson and H. Engqvist, The effect of composition on mechanical properties of brushite cements, J. Mech. Behav. Biomed. Mater., 2014, 29, 81–90 CrossRef CAS.
- F. Tamimi, B. Kumarasami, C. Doillon, U. Gbureck, D. Le Nihouannen and E. L. Cabarcos,
et al., Brushite-collagen composites for bone regeneration, Acta Biomater., 2008, 4(5), 1315–1321 CrossRef CAS.
- I. Lodoso-Torrecilla, J. van den Beucken and J. A. Jansen, Calcium phosphate cements: Optimization toward biodegradability, Acta Biomater., 2021, 119, 1–12 CrossRef CAS PubMed.
- E. Steijvers, A. Ghei, Z. Xia and E. Zx,
et al., Manufacturing artificial bone allografts: a perspective, Biomater. Transl. Med., 2022, 3(1), 65–80 Search PubMed.
Footnote |
† These authors contributed equally to this work. |
|
This journal is © The Royal Society of Chemistry 2023 |
Click here to see how this site uses Cookies. View our privacy policy here.