DOI:
10.1039/D2BM01551J
(Paper)
Biomater. Sci., 2023,
11, 248-262
ICG-loaded and 125I-labeled theranostic nanosystem for multimodality imaging-navigated phototherapy of breast cancer†
Received
26th September 2022
, Accepted 26th October 2022
First published on 16th November 2022
Abstract
Multimodality imaging-navigated precise phototherapy has been well-established as a promising strategy for enhancing the diagnostic and therapeutic efficiency of cancer in preclinical trials. However, proper theranostic agents with adequate biosafety and biological efficacy as well as simple components and preparations are still in great demand to promote the clinical translation of this regimen. Here, we developed a multifunctional nanosystem based on the self-assembly of FDA-approved indocyanine green (ICG) and 125I-labeled glycopeptides, which were composed of FDA-approved natural polysaccharide sodium alginate and endogenous tyrosine, for fluorescence imaging/single photon emission computed tomography (FLI/SPECT)-guided synergistic photothermal/photodynamic therapy (PTT/PDT) of breast cancer. The as-prepared ICG@ADY(125I) NPs possessed a stable nanostructure and radiolabel, an ICG-equivalent ROS and hyperthermia generation property, and a preferable photo/photothermal stability and biocompatibility, favoring its tumor homing, multimodality imaging, and phototherapy with high biosafety. Consequently, ICG@ADY(125I) NPs smoothly accumulated in tumors by virtue of their long blood circulation (t1/2 = 15.76 ± 1.34 h) and the EPR effect, thereby presenting highly sensitive FLI/SPECT images to realize cancer diagnosis. Guided by multimodality imaging, accurate PTT/PDT was performed using NIR laser irradiation, achieving a high tumor inhibition rate (81.8%) against 4T1 breast cancer models without appreciable side effects. Altogether, this theranostic nanosystem may have huge potential for the clinical diagnosis and treatment of breast cancer.
Introduction
Breast cancer is the most commonly diagnosed malignancy and the leading cause of cancer-induced deaths in women globally.1,2 Although numerous antitumor treatment modes, such as radiotherapy, chemotherapy, immunotherapy, and surgery, have undergone rapid development in the past few years, new-style and effective therapeutics against breast cancer are still insufficient.3–8 In the past decades, phototherapy, mainly including photothermal therapy (PTT) and photodynamic therapy (PDT), has received increasing attention in cancer treatment due to its remarkable spatiotemporal controllability, low side effects, and minimal invasiveness.9–12 PTT and PDT usually utilize photothermal conversion agents (PTCAs)13–15 and photosensitizers (PSs)16–18 gathered in tumor tissues to absorb specific wavelengths of light, especially near-infrared (NIR) light, to generate local hyperthermia and cytotoxic reactive oxygen species (ROS), respectively, for eliminating tumors. Recently, the ingenious integration of a PTCA and a PS into one system to exert the synergistic action of PTT and PDT has been corroborated to be more advantageous over monotherapy.19–21 Concretely, by combining PTT and PDT, PTT-produced heat can induce cell membrane permeability increase and intratumoral blood flow acceleration to elevate the cellular internalization of PS and oxygen supplement in tumors, respectively, thus facilitating PDT.22–24 In turn, PDT-generated ROS is able to eliminate heat-resistant tumor cells to potentiate PTT efficacy.24–26 However, as an exogenous light-activated therapy modality, phototherapy has to be performed under the guidance of imaging to delineate the lesion spot and determine the optimal irradiation time point.27,28 Facing this dilemma, various imaging techniques have been employed in combination with phototherapy for improving treatment accuracy and efficacy. Nevertheless, single-modality imaging often provides limited information, which may be insufficient to obtain precise tumor diagnosis and localization outcomes. Alternatively, multimodality imaging that integrates data from several imaging modalities is expected to compensate for the inherent shortcomings of each modality and acquire more comprehensive diagnostic information.29–34 Among miscellaneous imaging modalities, fluorescence imaging (FLI) has emerged as a powerful tool for cancer diagnosis by virtue of its high sensitivity, relatively low cost, simplicity of operation, and real-time imaging performance.35 However, FLI has limited imaging depth, and the autofluorescence of bio-tissues inevitably introduces substantial background signals, both of which severely restrict the quality of images.36 Unlike FLI, single photon emission computed tomography (SPECT) is recognized as one of the most important and frequently used radio-imaging techniques in clinical practice due to its superb sensitivity, unlimited tissue penetration, low background interference, and accurate quantification advantages, while still exists the defect of long scanning time during imaging.37,38 In view of the commendable and complementary properties of FLI and SPECT, incorporating the advantages of these two patterns is particularly attractive when aiming at providing sensitive, quantifiable, and real-time imaging for tumor diagnosis and imaging-navigated phototherapy.39
The rapid development of advanced nanotechnology enables the rational integration of multimodality imaging probes and PTCAs/PSs into a single nanosystem, which can usually effectively accumulate in tumors with the aid of its unique long blood circulation and the enhanced permeability and retention (EPR) effect, exerting imaging and hyperthermia/ROS treatment functions.40–43 However, despite the tremendous development of such nanoagents, sophisticated designs, complex components, potential toxicities, and deficient theranostic outcomes severely restrict their clinical application.29,44 Thus, exploring multifunctional theranostic nanosystems with adequate biosafety and biological efficacy, as well as simple components and preparations, is still greatly desirable. The utilization of endogenous or clinically adopted matters with well-established pharmacokinetics may be a convenient and practical approach for constructing such nanomaterials with eligible biosafety.45 As a choice, U.S. Food and Drug Administration (FDA)-approved NIR fluorochrome indocyanine green (ICG) comes into our sight due to its outstanding FLI and PTT/PDT performance in the fight against cancer.46–48 Unfortunately, some inherent restrictions, including concentration-dependent aggregation, chemical, and optical instability, rapid body clearance, and non-specific binding to proteins, largely impede the effectiveness of ICG in cancer theranostics.49–51 For SPECT, low-energy γ-ray emitting radionuclide, 125I, was chosen as an ideal radiotracer for tumor imaging due to its suitable activity for long-term disease surveillance.52–54 However, free 125I trends to promptly concentrate on thyroids once injected in vivo, severely impairing its capability to visualize tumors.52 To circumvent the inherent defects of ICG and 125I, integrating them into a nanosystem may be a promising approach to improve their bioavailability, thereby maximizing the FLI, PTT, and PDT functions of ICG and the SPECT performance of 125I. To load ICG and 125I, biocompatible glycopeptides came to our attention for the design of applicable nanocarriers. Here, natural polysaccharide sodium alginate (ALG-Na)55 was employed as the hydrophilic section, and endogenous tyrosine (Tyr)56 was polymerized to serve as the hydrophobic portion and label 125I. The fabricated amphiphilic glycopeptides were expected to self-assemble into core–shell structured nanoparticles to encapsulate ICG via hydrophobic interaction, offering opportunities to combine FLI, SPECT, PTT, and PDT for cancer theranostics.
Here, we developed an ICG-loaded and 125I-labeled theranostic nanosystem with favorable biocompatibility for FLI/SPECT multimodality imaging-navigated synergistic PTT/PDT of breast cancer. As demonstrated in Scheme 1, the amphiphilic glycopeptide ALG-Na-DAB-PTyr was labeled with radionuclide 125I to form ALG-Na-DAB-PTyr(125I), which can conveniently self-assemble into stable nanoparticles to encapsulate ICG, yielding the target ICG@ADY(125I) NPs with a hydrophilic ALG-Na shell and a hydrophobic DAB-PTyr(125I) and ICG core. Benefiting from its suitable nanoscale and commendable pharmacokinetics, ICG@ADY(125I) NPs could smoothly accumulate in tumors and efficiently exhibit high-quality FLI/SPECT images under a NIR stimulation, thereby realizing the precise diagnosis of tumors. Navigated with multimodality imaging, accurate PTT/PDT can be conducted with minimal systemic side effects using an 808 nm laser irradiation. Collectively, this multifunctional nanosystem with biocompatible components and simple preparation together with superb tumor imaging and treatment performance is expected to be a potential candidate for clinical theranostics of breast cancer.
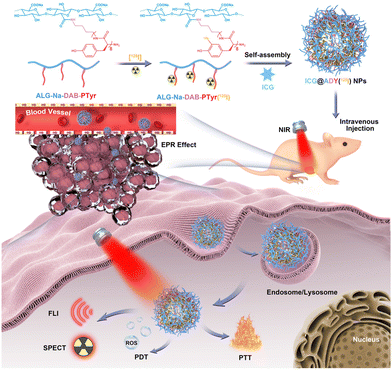 |
| Scheme 1 The preparation of ICG-loaded and 125I-labeled theranostic nanosystem (ICG@ADY(125I) NPs) for FLI/SPECT-navigated PTT/PDT of breast cancer. | |
Materials and methods
Materials
ICG, indocyanine green N-hydroxysulfosuccinimide ester (ICG-Sulfo-OSu), and L-tyrosine-N-carboxyanhydride (Tyr-NCA) were purchased from Xi'an Ruixi Biological Technology Company (Xi'an, China). Na125I was obtained from PerkinElmer Corporation. N-Boc-1,4-diaminobutane (Boc-DAB), trifluoroacetic acid (TFA), alginic acid (ALG-H), ALG-Na, tetrabutylammonium hydroxide (TBA-OH), 2-chloro-1-methylpyridinium iodide (CMPI), chloramine-T, sodium pyrosulfite (Na2S2O5), dichloromethane (DCM), N,N′-dimethylformamide (DMF), dimethyl sulfoxide (DMSO), Triton X-100, 2′,7′-dichlorofluorescin diacetate (DCFH-DA), and 1,3-diphenylisobenzofuran (DPBF) were obtained from J&K Chemical Technology Company (Beijing, China). Penicillin/streptomycin, fetal bovine serum (FBS), RPMI-1640 medium, Cell Counting Kit-8 (CCK-8), 4′,6-diamidino-2-phenylindole dihydrochloride (DAPI), and Calcein-AM/PI double stain kit were purchased from Gibco BRL (Eggenstein, Germany). All the commercially available reagents were used as received. Ultrapure Milli-Q water was used for preparing various aqueous solutions. All the cell lines were maintained in our lab. All the mice were purchased from Beijing Vital River Laboratory Animal Corporation (Beijing, China) and maintained under specific pathogen-free conditions.
Synthesis of ALG-Na-DAB-PTyr(125I) glycopeptide
Boc-DAB (1 mmol, 0.188 g) and Tyr-NCA (8 mmol, 1.656 g) were dissolved in 20 mL of absolute DMF and stirred at 50 °C for 24 h under N2 atmosphere. Then, the mixture was added dropwise into diethyl ether to obtain white precipitates as the crude product of N-boc-1,4-diaminobutane-poly(L-tyrosine) (Boc-DAB-PTyr). Next, a mixture of TFA (10 mL) and DCM (10 mL) was used to dissolve the obtained Boc-DAB-PTyr precipitate, followed by a 4 h of reaction at room temperature to remove the Boc protecting group. The solvent was removed by rotary evaporation, and the residue was redissolved in 2 mL of DMSO, followed by a dialysis and lyophilization process to obtain the pure product of 1,4-diaminobutane-poly(L-tyrosine) (DAB-PTyr) as a white powder (1.191 g, yield: 85.5%).
TBA-OH (25% in water) was dropwise added to a solution of ALG-H (3 g) in 90 mL of Milli-Q water under stirring. When the pH value reached 7.4, the mixture was lyophilized to obtain tetrabutylammonium alginate (ALG-TBA) as a white powder (6.776 g, yield: 95.3%).
ALG-TBA (1 mmol, 417 mg) was dispersed in 40 mL of absolute DMF, followed by a 12 h stirring at room temperature to completely dissolve the solid. CMPI (0.1 mmol, 26 mg) was added to the solution at 0 °C under N2 atmosphere to activate the carboxylate groups. After 1 h of stirring at room temperature, TEA (0.15 mmol, 15 mg) and DAB-PTyr (0.05 mmol, 70 mg) were added, followed by a further stirring for 24 h under N2 atmosphere. Then, a saturated NaCl solution was added to exchange TBA+ with Na+, and the crude product was precipitated. The crude product was redissolved in water and precipitated again in EtOH to remove the unconjugated DAB-PTyr. After lyophilization, pure ALG-Na-DAB-PTyr was obtained as a white powder (184 mg, yield: 69.2%).
ALG-Na-DAB-PTyr (20 mg), chloramine-T (1 μmol, 0.23 mg), and Na125I (1 mCi) were dissolved in 1 mL of PB (pH 7.4), followed by 30 min of shaking at room temperature. Next, Na2S2O5 (1.5 μmol, 0.29 mg) was added to quench the reaction. ALG-Na-DAB-PTyr(125I) was then obtained via a dialysis and lyophilization process (13.78 mg, yield: 68.9%).
Preparation of ICG@ADY(125I) NPs
A solution of ALG-Na-DAB-PTyr(125I) (10 mg) in H2O (1 mL) and a solution of ICG (1 mg) in DMSO (1 mL) were simultaneously added dropwise into PBS (8 mL, pH 7.4) under vigorous stirring. After stirring overnight in the dark, the mixture was dialyzed against PBS for 3 days in the absence of light to completely remove the unloaded ICG and DMSO, and then ICG@ADY(125I) NPs were obtained. Blank ADY(125I) NPs were prepared in the same way without using ICG.
Characterizations
The structure of glycopeptides was confirmed from the 1H NMR spectrum collected using a nuclear magnetic resonance spectrometer (Bruker, 300 MHz). The substitution degree of DAB-PTyr in ALG-Na-DAB-PTyr (defined as the number of DAB-PTyr per 100 sugar units of ALG-Na) was determined using an elemental analyzer (Heraeus, Vario-EL). The radiolabeling rate and radiochemical purity were determined using radioactive thin-layer chromatography (TLC) scanner (BioScan, AR-2000). The critical micelle concentration (CMC) was determined using Nile Red as a fluorescence probe. The zeta potential and size distribution were detected using a dynamic light scattering (DLS) spectrometer (Malvern, BI-200SM). The morphology was characterized by transmission electron microscopy (TEM) images obtained on an electron microscope (FEI, Talos F200C). The UV-vis spectra were acquired on a UV-visible spectrophotometer (Shimadzu, UV-2550) and the fluorescence spectra were recorded using a fluorescence spectrophotometer (Hitachi, F-4600).
Photo/size/radiolabel stability
2 mL of free ICG and ICG@ADY(125I) NP solution with an equivalent ICG concentration (20 μg mL−1) were separately exposed under an 808 nm laser (Laserwave, LI-P20 W, 1 W cm−2) for 10 min. Before and 2, 4, 6, 8, and 10 min after the irradiation, UV-vis absorption spectra were collected. The maximum absorption values were recorded to evaluate the photostability of these two materials. Furthermore, photographs of these two solutions before and after irradiation were collected using a digital camera. Photostability assay was performed 3 times.
To evaluate the size stability, ICG@ADY(125I) NPs were diluted with serum, followed by 5 days of co-incubation at 37 °C. The hydrodynamic size was monitored every day. The size stability assay was performed 3 times.
ICG@ADY(125I) NPs were mixed with mouse plasma, followed by a 48 h of co-incubation at 37 °C. Before and after incubation, a radioactive TLC scanner was used to detect the radiochemical purity.
ROS generation
The generation of ROS in PBS was measured using DPBF. In detail, 2 mL of free ICG and ICG@ADY(125I) NP solutions were mixed with DPBF solution (freshly dissolved in CH3CN) to prepare the final cocktail with an equivalent DPBF concentration (30 μg mL−1) and ICG concentration (50 μg mL−1). Then, the mixtures received an 808 nm NIR laser (1 W cm−2) irradiation for 10 min. The UV-vis absorption spectra were collected at scheduled time points (0, 2, 4, 6, 8, and 10 min). The drop in the absorbance value of DPBF accompanied by ROS generation was measured at 410 nm. Pure DPBF solution was used as the control. This assay was performed 3 times.
Photothermal conversion and stability
ICG@ADY(125I) NPs and free ICG solutions (2 mL) with an equivalent ICG concentration (50 μg mL−1) were placed in a glass cuvette, followed by a 10 min of irradiation under an 808 nm laser (1 W cm−2). The temperature changes and thermal images were recorded using an infrared thermal imaging camera (Fluke, ST20 MAX) every 30 s during the irradiation. Besides, the natural cooling curve was recorded after the laser irradiation was switched off. PBS was used as the control. The photothermal conversion efficiency of these two materials were calculated according to our previous reports.57 To further assess the photothermal stability of the materials, ICG@ADY(125I) NPs and free ICG solutions (2 mL) with an equivalent ICG concentration (50 μg mL−1) were prepared and they received an 808 nm laser (1 W cm−2) irradiation for 5 min each time. Subsequently, the laser was turned off, and the solutions were naturally cooled to room temperature. The temperature of the solutions was recorded using an infrared thermal imaging camera. The cycle was repeated 5 times.
Cellular uptake and intracellular ROS detection
Mouse breast cancer 4T1 cells were seeded into confocal microscopic dishes (4 × 105 cells per dish) using the RPMI-1640 medium and were cultured overnight. Then, the initial medium was removed, and the cells were incubated with free ICG or ICG@ADY(125I) NPs possessing an equivalent ICG concentration (20 μg mL−1) with or without various inhibitors, including MβCD (1 × 10−2 M), amiloride (1 × 10−3 M), and chlorpromazine (15 μg mL−1), in serum-free medium at 37 °C for 4 h. After incubation, the cells were washed with PBS, fixed with 4% paraformaldehyde solution, and stained with DAPI. Lastly, the cellular uptake behavior and intracellular distribution of these two materials were investigated using a confocal laser scanning microscope (CLSM) (Nikon, C2). The intracellular fluorescence signal intensity was analyzed with ImageJ software. In order to investigate whether the endocytosis process of ICG@ADY(125I) NPs is energy-dependent, 4T1 cells received a 4 h of incubation with ICG@ADY(125I) NPs at 4 °C. Then, the cellular uptake was evaluated following the above procedure. Afterwards, mouse breast cancer 4T1 cells were seeded into 6-well plates (4 × 105 cells per well) using the RPMI-1640 medium and cultured overnight. Then, the cells received the same incubation treatment with ICG or ICG@ADY(125I) NPs with or without various inhibitors. The cells were then washed with PBS, digested, collected, and lysed by adding 20 μL of lysis solution for 30 min. Finally, the supernatant was obtained by centrifugation, and the absorbance at 780 nm was measured using a UV-visible spectrophotometer. This assay was repeated 3 times.
4T1 cells were seeded into confocal microscopic dishes (4 × 105 cells per dish) with the RPMI-1640 medium and cultured overnight. Next, the original medium was replaced by a fresh medium involving free ICG or ICG@ADY(125I) NPs with an equivalent ICG concentration (10 μg mL−1). After 4 h of incubation, the cells were rinsed with PBS, followed by 30 min of treatment with DCFH-DA (10 μM) in the absence of light. Afterward, the cells were rinsed with PBS and exposed under an 808 nm laser (1 W cm−2) for 5 min. Intracellular ROS images were acquired using an inversed fluorescence microscope (Leica, DMI 6000B) immediately, and the average fluorescence intensity was determined using ImageJ software. PBS-treated cells and unirradiated cells were set as the controls.
In vitro antitumor evaluation
The in vitro antitumor effect of ICG@ADY(125I) NPs with or without laser irradiation was evaluated using the CCK-8 kit. 4T1 cells were seeded into 96-well plates (1 × 104 cells per well) containing 100 μL of RPMI-1640 medium and incubated at 37 °C for 1 day. The cells then received the treatment with free ICG or ICG@ADY(125I) NPs with a series of ICG concentrations (0, 1, 5, 10, 25, and 50 μg mL−1). After incubating for 4 h, the cells were rinsed with PBS, exposed under an 808 nm laser (1 W cm−2) for 5 min, and incubated for 2 h. Then, 10 μL of CCK-8 in 100 μL of fresh medium was introduced into each well, followed by further incubation for 2 h. A microplate reader (Thermo Scientific, Varioskan Flash) was used to detect the absorbance at 450 nm. The cells receiving the treatment with free ICG or ICG@ADY(125I) NPs without irradiation were set as the controls. This test was performed 6 times.
To further assess the PTT/PDT cytotoxicity induced by ICG@ADY(125I) NPs on breast cancer cells, a live/dead staining assay was carried out. In detail, 4T1 cells were seeded into confocal microscopic dishes (4 × 105 cells per dish) and cultured for 12 h. Then, the fresh medium involving free ICG or ICG@ADY (125I) NPs with an equivalent ICG concentration (25 μg mL−1) was prepared to replace the original medium. After incubating for 4 h, the cells were rinsed with PBS, irradiated with an 808 nm laser (1 W cm−2) for 5 min, and received a further 2 h of incubation. Then, the cells were rinsed with PBS and stained with Calcein-AM/PI for 30 min. Finally, an inversed fluorescence microscope was used to observe the cells. PBS-treated cells and unirradiated cells were set as the controls.
Moreover, two other breast cancer cell lines (MDA-MB-231 and MCF-7) were used for performing live/dead staining assays and CCK-8 assays to further evaluate the in vitro antitumor activity of ICG@ADY(125I) NPs.
Biocompatibility evaluation
Free ICG and ICG@ADY(125I) NP solutions (0.5 mL) with a series of ICG concentrations (1, 5, 10, 25, 50, 100, and 200 μg mL−1) were individually incubated with fresh rat blood (2% in PBS, 0.5 mL) at 37 °C for 4 h. Afterwards, the mixtures were centrifugated (3000 rpm min−1) for 10 min. A digital camera was used to capture the hemolysis photos. Subsequently, the supernatant was taken out to measure the absorbance at 570 nm using a microplate reader. Positive and negative controls were used using Triton X-100 and PBS, respectively. The hemolysis ratio was determined according to our previous reports.57 The hemolysis assay was repeated 3 times.
L929 cells were seeded into 96-well plates (1 × 104 cells per well) containing 100 μL of RPMI-1640 medium and incubated for 1 day. Then, the cells were treated with fresh medium (100 μL) involving free ICG or ICG@ADY (125I) NPs with a series of ICG concentrations (0, 1, 2, 5, 10, 25, 50, 100, and 200 μg mL−1). After culturing for 24 h, the media was discarded. Lastly, 10 μL of CCK-8 in 100 μL of fresh medium was introduced into each well, followed by further incubation for 2 h. A microplate reader was adopted to detect the absorbance at 450 nm. The cytocompatibility assay was repeated 6 times.
6–8 Weeks old healthy BALB/c mice were casually distributed into 3 groups (n = 5) and received intravenous injections with 200 μL of PBS, ICG in PBS, or ICG@ADY (125I) NPs in PBS with an equivalent ICG concentration (100 μg mL−1), respectively. After two consecutive days of injection (once a day), the blood routine indexes, involving mean corpuscular volume (MCV), white blood cell (WBC), mean corpuscular hemoglobin concentration (MCHC), red blood cell (RBC), hemoglobin (HGB), mean corpuscular hemoglobin (MCH), platelets (PLT), and hematocrit (HCT), were analyzed by an automatic blood analyzer (Nihon Kohden, MEK-7222K) 1 day and 1 week later. In addition, main organs, including the liver, heart, spleen, kidney, and lung, were collected for H&E staining to further explore the potential side effects.
Tumor model
The tumor models used in this study were established via subcutaneous inoculation of 4T1 cells (1 × 106 cells per mouse) in the right flank of female BALB/c nude mice (4–6 weeks old). After the cells were inoculated, the tumor size was monitored every day. Once the tumors grew to about 100 mm3, follow-up in vivo tumor imaging and phototherapy were conducted. All animal procedures were performed in accordance with the Guidelines for the Care and Use of Laboratory Animals of Peking Union Medical College and approved by the Animal Ethics Committee of the Institute of Radiation Medicine, Chinese Academy of Medical Sciences.
Blood circulation
Healthy Spraque-Dawley rats (n = 3) at the age of 5 weeks were used to investigate the blood circulation performance of ICG@ADY(125I) NPs. Briefly, the rats were intravenously injected with 1 mL of ICG@ADY(125I) NP solution with a radiation dosage of 100 μCi. At designated time points (1 min, 5 min, 15 min, 30 min, 1 h, 2 h, 4 h, 8 h, 12 h, and 24 h post-injection), 200 μL of blood samples were collected from the orbital vein, weighed, and the amount of radioactivity was measured using an automatic gamma counter (PerkinElmer, 2470).
Multimodality imaging
The FLI potency of ICG@ADY(125I) NPs was investigated using a small animal optical imaging system (CRI, Maestro 2). In detail, tumor-bearing mice were casually distributed into 3 groups (n = 3), followed by intravenous injection with 200 μL of PBS, free ICG in PBS, or ICG@ADY (125I) NPs in PBS with the equal ICG concentration (100 μg mL−1). Before, and 0.5, 4, 10, 24, and 48 h after the administration, the fluorescence images were collected. After the in vivo imaging, these mice were euthanized to obtain tumors and the main organs (liver, heart, spleen, kidney, and lung) for ex vivo imaging to investigate the biodistribution of ICG@ADY(125I) NPs.
Before SPECT, KI (1%) in water was used to feed the employed tumor-bearing mice for 3 days to saturate the thyroid and avoid the uptake of 125I. Next, tumor-bearing mice were casually distributed into 2 groups (n = 3), followed by intravenous injections with 200 μL of Na125I or ICG@ADY(125I) NPs in PBS with an equivalent radiation dosage (100 μCi per mouse). At designated time points (0.5, 4, 10, 24, and 48 h post-injection), SPECT images were captured using a nanoScan SPECT-CT (Mediso). After in vivo imaging, these mice were euthanized to obtain tumors and the main organs (liver, heart, spleen, kidney, and lung) for weighing and recording the radioactivity amounts by an automatic gamma counter to perform the biodistribution analysis.
Imaging-navigated PTT/PDT
The in vivo PTT/PDT therapeutic efficacy of ICG@ADY(125I) NPs against the 4T1 breast cancer model was further assessed. Tumor-bearing mice were casually distributed into 6 groups (n = 6): PBS, PBS + Laser, ICG, ICG + Laser, ICG@ADY(125I) NPs, and ICG@ADY(125I) NPs + Laser. After receiving an intravenous injection with 200 μL of PBS, free ICG in PBS, or ICG@ADY (125I) NPs in PBS with an equivalent ICG concentration (100 μg mL−1) for 24 h, the tumors were exposed under an 808 nm laser (1 W cm−2) for 5 min. The temperature was recorded every 30 s using an infrared thermal imaging camera during the irradiation process. On each day after irradiation, the body weight and tumor volume of the mice were monitored. The tumor volume was calculated using the equation: V (mm3) = length (mm) × [width (mm)]2/2. The comparative tumor volume was calculated as V/V0, where V0 is the original tumor volume before treatment. After 20 days of monitoring and recording, the mice were sacrificed to collect the tumors for photographing and weighing. The tumor inhibition rate was calculated according to our previous reports.58 To further investigate the PTT/PDT efficacy, 2 days after treatment, 1 mouse from each group was euthanized to collect the tumors for TUNEL apoptosis staining, H&E staining, and Ki67 staining following the standard protocols. On the 20th day after treatment, the remaining mice were euthanized to obtain the main organs (liver, heart, spleen, kidney, and lung), which were then fixed with the formalin solution. After embedment and section, tissue samples were stained with H&E, followed by observation using an optical microscope (Leica, DMI 3000 B).
Statistical analysis
The statistical analyses were carried out using SPSS 22.0 software. Data are shown as mean ± standard deviation (SD). Student's t-test was adopted to determine the significant differences. * stands for P < 0.05, ** stands for P < 0.01, and *** stands for P < 0.001. P < 0.05 was considered statistically significant.
Results and discussion
Synthesis and characterization of the polymer and glycopeptides
The glycopeptide ALG-Na-DAB-PTyr(125I) was synthesized as follows (Fig. S1†): (i) the ring-opening polymerization of Boc-DAB with Tyr-NCA, followed by deprotection to obtain DAB-PTyr; (ii) the amidation reaction of ALG-TBA and DAB-PTyr to give ALG-Na-DAB-PTyr; (iii) the chloramine-T method to label ALG-Na-DAB-PTyr with 125I onto the ortho carbon of the phenolic hydroxyl group of Tyr, resulting in the target glycopeptide ALG-Na-DAB-PTyr(125I). The structure of the intermediate DAB-PTyr was identified from the 1H NMR spectrum. As shown in Fig. S2,† the signals around 1.36, 1.40, 2.33, and 2.99 ppm are associated with the methylene protons (c, b, a and d) in DAB. While the peaks around 2.75, 4.42, 6.60, 6.96, 7.98, and 9.13 ppm are assigned to the signal of benzyl proton (g), methyne proton (f), phenyl proton (h), phenyl proton (i), secondary amine proton (e), and phenolic hydroxyl proton (j) in PTyr, respectively. Based on the integration of these characteristic signals, the Tyr polymerization degree in DAB-PTyr was determined to be 8. Afterwards, we analyzed the 1H NMR spectrum of ALG-Na-DAB-PTyr by comparing it with that of ALG-Na (Fig. 1A). In addition to the proton signals of the ALG-Na, there also existed the characteristic proton signals (g, f, h, i) of PTyr in the spectrum of ALG-Na-DAB-PTyr, demonstrating the successful conjugation of DAB-PTyr to polysaccharide skeletons. Furthermore, the DAB-PTyr substitution degree of ALG-Na-DAB-PTyr was determined to be 1.45 on the basis of the nitrogen elemental analysis (Fig. S3†). After being labelled with radioisotope 125I, a radiolabeling rate of 93.5% and radiochemical purity of 99.8% were obtained for ALG-Na-DAB-PTyr(125I) (Fig. S4†).
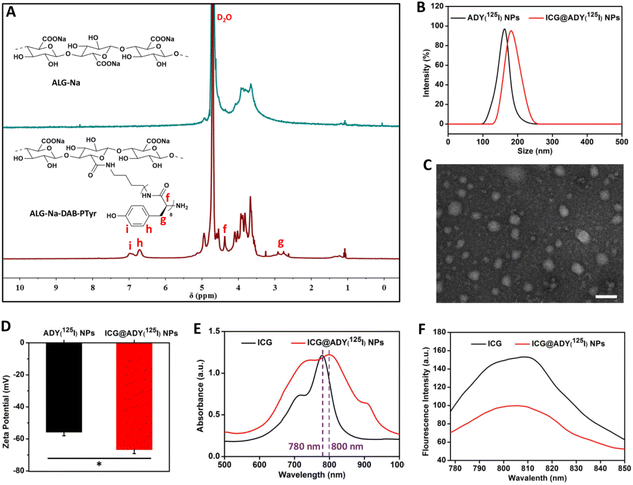 |
| Fig. 1 (A) 1H NMR spectra of ALG-Na and ALG-Na-DAB-PTyr in D2O. (B) Hydrodynamic size distribution of ADY(125I) NPs and ICG@ADY(125I) NPs. (C) The representative TEM image of ICG@ADY(125I) NPs. The scale bar is 200 nm. (D) Zeta potentials of ADY(125I) NPs and ICG@ADY(125I) NPs. The data are presented as the mean ± SD (n = 3). * stands for P < 0.05. The UV-vis absorption spectra (E) and the fluorescence spectra (F) of ICG and ICG@ADY(125I) NPs. | |
Preparation and characterization of the nanoparticles
The amphiphilic ALG-Na-DAB-PTyr(125I) could self-assemble into nanostructured ADY(125I) NPs with a hydrophilic ALG-Na shell and a hydrophobic DAB-PTyr(125I) core as a result of the molecular interactions between hydrophobic segments in aqueous media. The CMC of ADY(125I) NPs was determined to be approximately 25.8 μg mL−1 (Fig. S5†). Based on the hydrophobic interactions, ICG@ADY(125I) NPs were fabricated through the encapsulation of ICG into the core of ADY(125I) NPs. Both ADY(125I) NPs and ICG@ADY(125I) NPs exhibited a superb dispersity in an aqueous solution with a mean hydrodynamic diameter of 162.6 ± 5.9 nm and 181.7 ± 8.2 nm, respectively (Fig. 1B). TEM was then used to characterize the size and morphology of the materials. As presented in Figs. S6† and Fig. 1C, the two nanoparticles were both spherical-like in shape with an average diameter of 85.6 ± 7.5 nm for ADY(125I) NPs and 91.4 ± 6.8 nm for ICG@ADY(125I) NPs.59–61 The zeta potential of ICG@ADY(125I) NPs was −66.6 ± 2.6 mV, which was obviously lower than that of the blank nanoparticles (−55.6 ± 2.4 mV) (Fig. 1D). The size expansion together with the zeta potential decrease in ICG@ADY(125I) NPs in comparison with ADY(125I) NPs clearly indicated the successful deposition of the negatively-charged ICG molecules into the nanoparticles. Subsequently, UV-vis absorption spectra and fluorescence spectra were recorded to investigate the optical properties of ICG@ADY(125I) NPs. ICG exhibited a distinct characteristic absorption peak near 780 nm, which was also observed in that of ICG@ADY(125I) NPs with a much broader absorption region and an obvious red-shift (∼20 nm) due to the π–π stacking between ICG molecules (Fig. 1E).62 Besides, it was found that the fluorescence intensity of ICG was weakened after being loaded into the nanoparticles, which was probably a fluorescence self-quenching result of the ICG aggregation (Fig. 1F).63 On the basis of the linear relation between the UV-vis absorption values and the concentrations of the ICG, the ICG loading content and ICG loading efficiency of ICG@ADY(125I) NPs were determined to be 8.6 ± 0.9% and 94.6 ± 1.1%, respectively.
Photo/size/radiolabel stability
The photostability of the light-activated nanoparticles is a precondition for achieving satisfactory results of optical imaging and phototherapy. Exposed under an 808 nm laser (1 W cm−2), the absorption peaks of ICG and ICG@ADY(125I) NPs both decreased with the extension of irradiation time (Fig. 2A and B). However, the decreased rate of absorbance is distinctly different between the two materials. It was found that the maximum absorbance of ICG quickly decreased to 47.2% of its initial value after 10 min of irradiation, which was significantly lower than that of ICG@ADY(125I) NPs (63.7%) (Fig. 2C). Furthermore, after the irradiation, the green color of the ICG solution remarkably faded and presented an obviously lighter color than that of the ICG@ADY(125I) NP solution due to severe photo-bleaching (Fig. 2C). These results prove that loading ICG into ADY(125I) NPs endows ICG with enhanced photostability. The stability in serum is an important indicator to evaluate whether the nanoparticles can perform long-term circulation. We monitored the hydrodynamic size of ICG@ADY(125I) NPs in serum over 5 days. The negligible change in their hydrodynamic size greatly corroborated the splendid storage stability and physiological stability of ICG@ADY(125I) NPs (Fig. 2D). The radiolabel stability is of vital importance for SPECT. After being co-incubated with mouse plasma at 37 °C for up to 48 h, the radiochemical purity remained as high as 99.3% (Fig. S7B†), and only a tiny amount of radioactive iodine was lost from the original nanoparticles (radiochemical purity: 99.6%, Fig. S7A†), demonstrating the excellent radiolabel stability of ICG@ADY(125I) NPs.
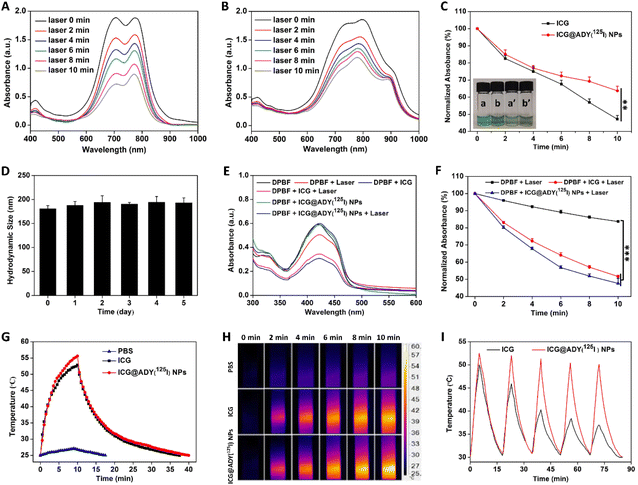 |
| Fig. 2 The UV-vis absorption spectra of ICG (A) and ICG@ADY(125I) NPs (B) under an 808 nm laser (1 W cm−2) irradiation at different time points. (C) Time-dependent variation in the normalized absorbance values of ICG and ICG@ADY(125I) NPs with irradiation. The inset photograph stands for ICG (a and a′) and ICG@ADY(125I) NPs (b and b′) solutions before (a and b) and after (a′ and b′) irradiation. (D) The hydrodynamic size of ICG@ADY(125I) NPs in serum during 5 days of storage. (E) The UV-vis absorption spectra of DPBF dissolved in pure PBS, ICG solution, or ICG@ADY(125I) NP solution with or without laser irradiation for 10 min. (F) A time-dependent decline in the normalized absorbance values of DPBF in the presence of PBS, ICG, or ICG@ADY(125I) NPs with irradiation. (G) The temperature change curves of PBS, ICG, and ICG@ADY(125I) NPs under an 808 nm laser (1 W cm−2) irradiation. (H) The thermal imaging photographs of pure PBS, ICG solution, and ICG@ADY(125I) NP solutions with irradiation at different time points. (I) The photothermal stability of ICG and ICG@ADY(125I) NPs under repeated 808 nm laser irradiation for five cycles. The data are presented as the mean ± SD (n = 3). ** stands for P < 0.01 and *** stands for P < 0.001. | |
1O2 generation
ROS production is a key factor in determining PDT efficacy. The 1O2 production by ICG and ICG@ADY(125I) NPs was determined on the basis of the decreased absorbance of DPBF using UV-vis spectroscopy. As shown in Fig. 2E and F, under 808 nm laser irradiation, the characteristic absorbance of DPBF at 410 nm decreased rapidly with time in the presence of ICG or ICG@ADY(125I) NPs due to the 1O2-induced oxidation of DPBF. The maximum UV-vis absorption of DPBF sharply declined to 47.6% of its initial value after 10 min of irradiation, which was similar to that of ICG (51.6%). However, the absorbance decrease was negligible in pure DPBF solution after 10 min of irradiation. These results demonstrate the remarkable 1O2-generating capability of ICG@ADY(125I) NPs.
Photothermal conversion of ICG@ADY(125I) NPs
In order to explore the photothermal conversion performance of ICG@ADY(125I) NPs, the temperature changes were monitored in real-time using 808 nm laser irradiation (1 W cm−2) for 10 min. As shown in Fig. 2G, ICG and ICG@ADY(125I) NPs induced the temperature to reach from 25.0 °C to 52.8 °C and 55.6 °C, respectively. However, the blank control PBS only exhibited an inapparent temperature rise. Based on the heating and cooling curves, the photothermal conversion efficiency of the ICG@ADY(125I) NPs was calculated to be 20.4%, which was slightly higher than that of ICG (19.0%). Moreover, during irradiation, the infrared thermal images were photographed and are shown in Fig. 2H. To probe the photothermal stability, ICG and ICG@ADY(125I) NP solutions were subjected to repetitive laser irradiation and cooling down (Fig. 2I). With the extension of irradiation time, the peak temperature of the ICG solution sharply declined because of the photo-bleaching of ICG molecules. Whereas, the peak temperature of ICG@ADY(125I) NPs remained nearly constant during the five cycles of turning the laser on and off, indicating the elevated photothermal stability of the loaded ICG. The enhanced photothermal stability may be attributed to the isolation of the encapsulated ICG from the surrounding environment by ADY(125I) NPs, leading to the reduction of water-induced transformations.46,64 The satisfactory photothermal conversion capability and photothermal stability of ICG@ADY(125I) NPs provided a practical foundation for the follow-up study on tumor PTT.
Cellular uptake
A CLSM was used to investigate the localization of ICG@ADY(125I) NPs in 4T1 cells. After 4 h of incubation, compared with ICG, ICG@ADY(125I) NP-treated cells presented a significantly stronger fluorescence intensity in the cytoplasm, suggesting that the intracellular concentration of the ICG@ADY(125I) NPs was higher than that of ICG (Fig. 3A). The results demonstrated that the encapsulation of ICG into nanoparticles could improve its cellular uptake performance, favoring tumor imaging and treatment function of ICG@ADY(125I) NPs. The endocytosis pathway of nanomaterials is generally accepted to include caveolae-mediated endocytosis (CvME), clathrin-mediated endocytosis (CME), and micropinocytosis (MP).65,66 To further verify the cellular uptake mechanism of ICG@ADY(125I) NPs, various endocytosis inhibitors, including MβCD (inhibitor of CvME), amiloride (inhibitor of MP), and chlorpromazine (inhibitor of CME), were introduced to incubate with the cells during the cellular uptake process. As shown in Fig. 3A and B, the intracellular fluorescence intensity of ICG@ADY(125I) NPs in the 4T1 cells treated with chlorpromazine, amiloride, and MβCD decreased to 62.8%, 46.7%, and 35.0%, respectively, compared with the cells free of inhibitors. These results comprehensively demonstrated that ICG@ADY (125I) NPs were internalized into the cells through CME, CvME, and MP, of which CvME was the predominant pathway. Moreover, the intracellular fluorescence intensity of ICG@ADY(125I) NPs at 4 °C was merely 18.6% of that at 37 °C, suggesting that the internalization of this material was mediated by energy-dependent endocytic processes. The quantitative analysis of ICG in 4T1 cells was determined using a UV-visible spectrophotometer and revealed similar results as discussed above, further demonstrating the enhanced endocytosis of the encapsulated ICG and the energy-dependent CvME-mediated endocytic pathway of ICG@ADY(125I) NPs (Fig. S8†).
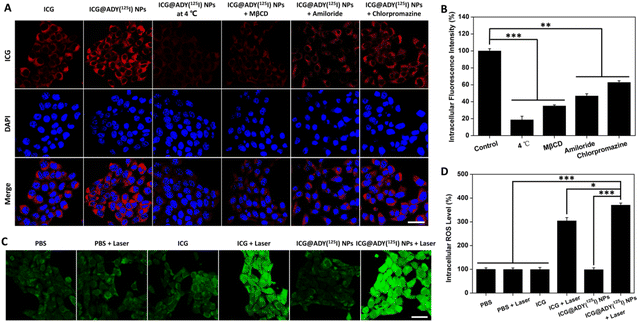 |
| Fig. 3 CLSM images (A) and the intracellular fluorescence intensities (B) of 4T1 cells after the treatment with ICG or ICG@ADY(125I) NPs with or without various inhibitors. The inverted fluorescence microscopy images (C) and the intracellular fluorescence intensities (D) of ROS generation in 4T1 cells after the treatment with PBS, ICG, or ICG@ADY(125I) NPs with or without irradiation. The data are presented as the mean ± SD (n = 3). * stands for P < 0.05, ** stands for P < 0.01, and *** stands for P < 0.001. Scale bars are 50 μm. | |
Intracellular ROS production
ROS can lead to irreversible cell damage through the oxidation of proteins and DNA. The generation of ROS in 4T1 cells was visualized using DCFH-DA, which can penetrate cell membranes and be oxidized by ROS to emit intracellular fluorescence. As indicated in Fig. 3C, in the absence of laser irradiation, the PBS-treated cells showed weak fluorescence, suggesting a small amount of intrinsic ROS inside 4T1 cells. This phenomenon was also observed in the cells treated with ICG or ICG@ADY(125I) NPs without irradiation. In contrast, ICG + Laser group and ICG@ADY(125I) NPs + Laser group exhibited significantly enhanced fluorescence intensities after irradiation. Through quantitative analysis (Fig. 3D), the average fluorescence intensity of ICG@ADY(125I) NP-treated cells after irradiation was about 3.7 folds higher than that before irradiation, indicating the splendid intracellular ROS production capability of ICG@ADY(125I) NPs under laser irradiation. Moreover, ICG@ADY(125I) NPs + Laser group exhibited more ROS production than the ICG group, which was probably ascribed to the superior cellular uptake of ICG@ADY(125I) NPs compared with free ICG. These results indicated that ICG@ADY(125I) NPs could be used as a favorable PS for tumor PDT under 808 nm laser excitation.
In vitro antitumor evaluation
To investigate the phototherapy effect, in vitro the antitumor activity of ICG@ADY(125I) NPs against 4T1 cells, MDA-MB-231 cells, and MCF-7 cells was evaluated by CCK-8 assays. As indicated in Fig. 4A–C, a remarkable enhancement in the damage to cell viability was seen with the increase of ICG concentrations. Especially, when the ICG concentration reached 25 μg mL−1, the viability of the three cell lines were all below 20%. In contrast, the cytotoxicity was negligible for the cells without receiving irradiation due to the lack of ROS or hyperthermia production. To further investigate and fluorescently visualize the PTT/PDT cytotoxicity, Calcein-AM/PI fluorescent staining was applied to distinguish the live/dead cells. As displayed in Fig. 4D, in the absence of laser irradiation, almost no red fluorescence was observed in the PBS-, ICG-, or ICG@ADY(125I) NPs-treated tumor cells, indicating the inappreciable dark cytotoxicity. However, with laser irradiation, as expected, a large number of red fluorescent signals appeared in the ICG- or ICG@ADY(125I) NP-treated cells, suggesting a high cell mortality rate. These results clearly indicated the superb PTT/PDT efficiency of ICG@ADY(125I) NPs against breast cancer tumor cells.
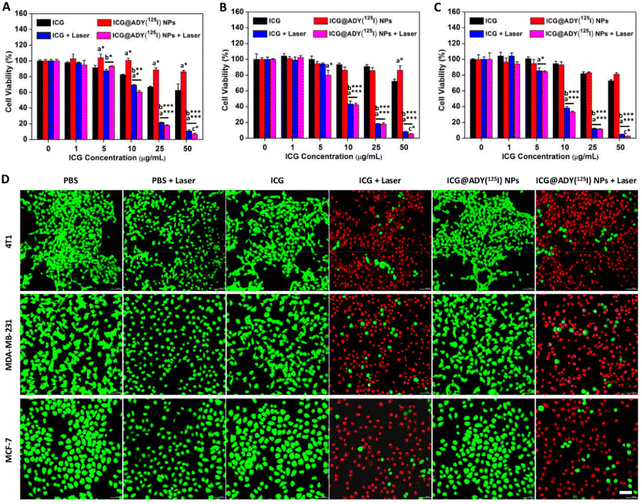 |
| Fig. 4 The cell viabilities of 4T1 (A), MDA-MB-231 (B), and MCF-7 (C) cells after incubation with ICG or ICG@ADY(125I) NPs at various ICG concentrations with or without laser irradiation determined by CCK-8 assays. Data are presented as the mean ± SD (n = 6). * stands for P < 0.05, ** stands for P < 0.01, and *** stands for P < 0.001 (a = in comparison with ICG group, b = in comparison with ICG@ADY(125I) NPs group, and c = in comparison with ICG + Laser group). (D) Inverted fluorescence microscopy images of 4T1, MDA-MB-231, and MCF-7 cells after the treatment with PBS, ICG, or ICG@ADY(125I) NPs with or without irradiation as determined by live/dead staining assays. The scale bar is 100 μm. | |
Biocompatibility
Hemolysis, cytotoxicity, and hematology analysis were conducted for biocompatibility evaluations and to determine the maximum nanoparticle concentration used for the subsequent in vivo experiments. As displayed in Fig. 5A and B, ICG showed apparent hemolytic phenomena at high concentrations, for instance, the hemolysis ratio at 100 μg mL−1 was 8.8%. However, ICG@ADY(125I) NPs did not disrupt erythrocytes to cause hemolysis at this ICG concentration (100 μg mL−1, hemolysis ratio: 2.2%). After 24 h of incubation with ICG@ADY(125I) NPs, no serious cytotoxicity toward L929 cells was observed at ICG concentration of 0–100 μg mL−1, in which the cell viabilities remained higher than 80% (Fig. 5C). To further investigate the systemic toxicity of ICG@ADY(125I) NPs in vivo, three groups of healthy mice were separately administered with PBS, ICG, and ICG@ADY(125I) NPs with a high ICG dose (100 μg mL−1). Blood was collected for analyses, and there was no difference in WBC, RBC, HGB, MCH, HCT, MCV, MCHC, and PLT for the mice with or without the administration of the nanoparticles (Fig. 5D–K). In addition, H&E staining of the main organs of ICG@ADY(125I) NPs-treated mice presented no evident apoptosis, necrosis, or other pathological abnormities (Fig. S9†). All the results demonstrated that ICG@ADY(125I) NPs were highly biocompatible, and nanoparticles with no more than 100 μg mL−1 (ICG concentration) were safe enough for further tumor imaging and treatment study.
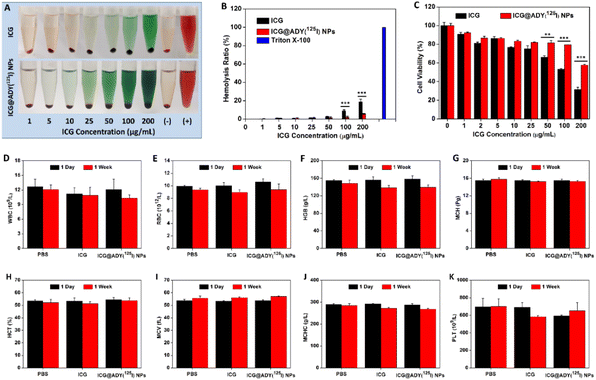 |
| Fig. 5 The hemolysis photographs (A) and the hemolysis ratios (B) of ICG and ICG@ADY(125I) NPs at various ICG concentrations. Data are shown as the mean ± SD (n = 3). (C) The cell viability of L929 cells after incubation with ICG or ICG@ADY(125I) NPs at various ICG concentrations. The data are presented as the mean ± SD (n = 6). (D–K) The changes in hematological markers 1 day and 1 week after the treatment with PBS, ICG, or ICG@ADY(125I) NPs. The data are presented as the mean ± SD (n = 5). ** stands for P < 0.01 and *** stands for P < 0.001. | |
Blood circulation
Nanoagents with long blood circulation capability are a precondition for their effective accumulation in tumors, and thus crucial for tumor theranostics. After being intravenously injected into healthy rats, the internal circulation behavior of ICG@ADY(125I) NPs was assessed by detecting the radioactivity in the blood at different time points. According to the blood clearance curve (Fig. 6A), ICG@ADY(125I) NPs showed a significantly longer blood circulation half-life (t1/2) (15.76 ± 1.34 h) than that of free ICG.67 The prolonged blood circulation time of ICG@ADY(125I) NPs was attributed to its appropriate nanoscale and decent physiological stability, which would be beneficial for the tumor accumulation of this nanomaterial.
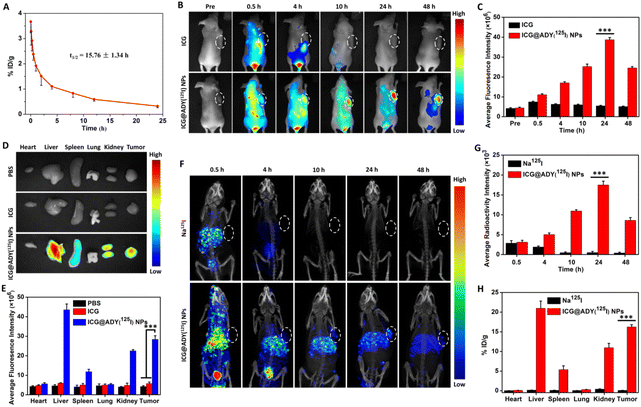 |
| Fig. 6 (A) Blood clearance curve of ICG@ADY(125I) NPs. The in vivo fluorescence images of the 4T1 tumor-bearing mice (B) and the average fluorescence intensity in the tumor regions (C) before and 0.5, 4, 10, 24, and 48 h after the injection with ICG or ICG@ADY(125I) NPs. The ex vivo fluorescence images (D) and the mean fluorescence intensity (E) of the isolated tumors and organs 48 h after the injection with PBS, ICG, or ICG@ADY(125I) NPs. The in vivo SPECT-CT images of tumor-bearing mice (F) and the average radioactivity intensity in the tumor regions (G) at 0.5, 4, 10, 24, and 48 h post-injection with Na125I or ICG@ADY(125I) NPs. (H) The distribution of Na125I and ICG@ADY(125I) NPs in the tumors and main organs at 48 h post-injection. The data are presented as the mean ± SD (n = 3). *** stands for P < 0.001. | |
Multimodality imaging
ICG@ADY(125I) NPs were injected into 4T1 tumor-bearing nude mice through the tail vein to investigate their in vivo multimodality imaging functions. For FLI, as presented in Fig. 6B, free ICG exhibited obvious fluorescence across the whole body at 0.5 h post-injection, followed by quick metabolism, and the fluorescence signals were hardly detectable 4 h after administration. In contrast, the fluorescence signals at the tumor sites of the mice treated with ICG@ADY(125I) NPs gradually heightened with time and reached a maximum at 24 h post-injection. The semi-quantitative analysis (Fig. 6C) indicated that the intratumoral mean fluorescence signal of ICG@ADY(125I) NPs was approximately 7 times stronger than that of ICG at 24 h post-injection, revealing the excellent tumor accumulation behavior of the nanoparticles. Subsequently, the tumors and representative organs were isolated for ex vivo FLI 48 h after the administration to further validate the biodistribution of this nanomaterial. As depicted in Fig. 6D and E, for ICG@ADY(125I) NP-treated mice, the strongest fluorescence signals were presented in the liver, followed by the tumor, kidney, spleen, and very weak fluorescence signals were detected in the heart and lung. Nevertheless, the ICG group exhibited the same weak fluorescence signal as the PBS group, demonstrating the complete clearance of free ICG. To probe the SPECT capability of ICG@ADY(125I) NPs in vivo, radioactivity signals were recorded at the predetermined time points. As exhibited in Fig. 6F, the intratumoral radioactivity signal intensity gradually strengthened with time and attained a maximum at 24 h post-injection with ICG@ADY(125I) NPs, which was roughly in line with the FLI results. After that, because of the metabolic clearance of the nanoparticles in physiological conditions, the signals gradually weakened as time progressed. Notably, the tumor could be distinctly visualized in the mice 10 h after the administration of ICG@ADY(125I) NPs. Nevertheless, there is no appreciable radioactivity signal in the tumors of mice treated with Na125I as a result of its rapid body clearance. Fig. 6G reveals that the intratumoral mean radioactivity intensity of the ICG@ADY(125I) NP group was much stronger than that of the Na125I group at 24 h post-injection. From the radioactivity counting of the isolated tumors and organs 48 h after administration, ICG@ADY(125I) NPs were determined to be mostly distributed in the liver, tumor, and kidney (Fig. 6H). Notably, ICG@ADY(125I) NPs presented the highest enrichment in the liver, indicating that our nanoparticles were prevailingly eliminated via the hepatobiliary system. Collectively, ICG@ADY(125I) NPs could accurately target and effectively concentrate in 4T1 tumors through the EPR effect owing to their suitable particle size and long blood circulation capability, and then simultaneously presented fluorescence signals and radioactivity signals with extremely high sensitivity, providing a promising FLI/SPECT nanoprobe for the diagnosis of breast cancer.
In vivo PTT/PDT of breast cancer tumors
Encouraged by the findings of excellent in vitro antitumor activity and remarkable in vivo FLI/SPECT performance of ICG@ADY(125I) NPs, we eventually evaluated the PTT/PDT efficacy against 4T1 breast cancer tumor models for this nanomaterial. Both FLI and SPECT revealed that the maximum concentration of ICG@ADY(125I) NPs in the tumor regions occurred at 24 h post-injection, the tumors were subjected to an 808 nm laser (1 W cm−2) irradiation at this time point to perform phototherapy. As depicted in Fig. 7A and Fig. S10,† after 5 min of exposure, the temperature in the ICG@ADY(125I) NPs-enriched tumors rapidly increased from 36.3 °C to 52.5 °C, which was high enough to cause irreversible damage to tumor cells. However, ICG-treated mice did not show significant temperature changes in tumors under the same irradiation conditions due to the fast clearance and deficient tumor accumulation of free ICG molecules. After PTT/PDT, we continuously monitored the growth of tumors to assess the treatment efficacy of various materials. As shown in Fig. 7B and C, after irradiation, remarkable suppression of tumor proliferation in the ICG@ADY(125I) NPs-treated mice was achieved attributed to NIR laser-excited local ROS and hyperthermia production by the designed nanoparticles. Conversely, other groups exhibited a frustrating tumor suppression, indicating that both intratumoral abundant PTCA/PS and laser were crucial for achieving satisfactory PTT/PDT efficacy. The size and morphology of the collected tumors were visually observed from the photographs shown in Fig. 7D. Tumors in ICG@ADY(125I) NPs + Laser group were the smallest among all the isolated tumors, with one tumor completely eradicated, which was in line with the tumor inhibition curves. By weighing the tumors, ICG@ADY(125I) NPs with irradiation showed the most remarkable treatment efficacy with a superb tumor inhibition rate of 81.8% (Fig. 7E). In addition, the antitumor effect was further assessed by H&E, TUNEL, and Ki67 staining 48 h after phototherapy. As shown in Fig. 7F, considerable cell shrinkage, nucleus loss, and karyolysis, which are the characteristics of dead cells, were found in the H&E-stained tumor tissues in the ICG@ADY(125I) NPs + Laser group. While tumors in other groups presented tightly packed cancer cells, and no obvious cell destruction was found. TUNEL staining and Ki67 staining revealed the same result with H&E staining, and tumor slices in the ICG@ADY(125I) NPs + Laser group presented the largest positive staining area of the apoptotic cells and the smallest positive staining area of the proliferative cells, respectively. These findings agreed well with the in vitro antitumor results, corroborating that ICG@ADY(125I) NPs with NIR laser irradiation could achieve a favorable tumor inhibition efficiency ascribed to the synergism of PTT and PDT. To evaluate the in vivo biosafety of ICG@ADY(125I) NPs treatment, we monitored the body weight changes of the mice throughout the experiment. No significant difference in body weight changes was found in all the mice, indicating the inappreciable side effects induced by ICG@ADY(125I) NPs (Fig. S11†). Finally, the potential toxicity of ICG@ADY(125I) NPs phototherapy was investigated through H&E staining of the main organs on the 20th day after PTT/PDT (Fig. S12†). Apparently, there is no visible injury or inflammatory lesion in the organs of the ICG@ADY(125I) NPs + Laser group compared with the other 5 groups, revealing the excellent organ compatibility of this nanomaterial. All of the above results demonstrated that the as-prepared ICG@ADY(125I) NPs were capable of serving as hopeful theranostic nanoagents for multimodality imaging-navigated PTT/PDT of breast cancer with excellent biosafety.
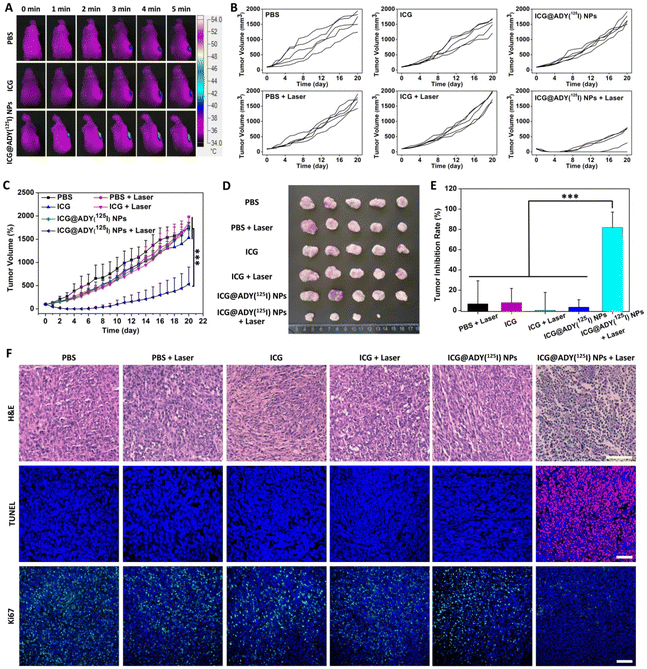 |
| Fig. 7 (A) Thermal images of the 4T1 tumor-bearing mice during an 808 nm laser (1 W cm−2) irradiation 24 h after the intravenous administration of PBS, ICG, or ICG@ADY(125I) NPs. The individual (B) and overall (C) tumor volume curves of the mouse after receiving various treatments. (D) Photographs of the isolated tumors 20 days after irradiation. (E) The tumor inhibition rate of various materials with or without laser irradiation in comparison with PBS. (F) H&E, TUNEL, and Ki67 staining of the tumor slices 2 days after the irradiation. Scale bars are 100 μm. The data are presented as the mean ± SD (n = 5). *** stands for P < 0.001. | |
Conclusions
In the present study, we successfully designed and prepared ICG-loaded and 125I-labeled nanoparticles based on a glycopeptide, serving as a multifunctional nanosystem for FLI/SPECT multimodality imaging-navigated PTT/PDT of aggressive breast cancers. In this system, ICG simultaneously served as a fluorescence dye for FLI, a PTCA for hyperthermia production, and a PS for ROS generation under NIR activation. Besides, the labeled 125I on the Tyr residues emitted a highly sensitive radioactivity signal for SPECT. The fabricated ICG@ADY(125I) NPs exhibited improved stability, blood circulation capability, and tumor accumulation of free ICG. As a result, FLI/SPECT images with high sensitivity and limitless tissue penetration were obtained for the accurate diagnosis of tumors. Under the guidance of multimodality imaging, a remarkable tumor suppression effect was achieved through NIR-activated synergistic PTT/PDT. In addition, this multifunctional system, mainly composed of FDA-approved ALG-Na and ICG and endogenous Tyr, possessed exceptional biocompatibility and inappreciable systemic toxicity, ensuring its safe application in vivo. It is hoped that this integrated theranostic nanosystem may become a practicable implementation for the precise diagnosis and effective treatment of breast cancer.
Author contributions
D. Wang: investigation, visualization; J. Yue: investigation, writing – original draft; Q. Cao: investigation, visualization; J. Liu: methodology, funding acquisition; L. Yang: conceptualization, data curation, writing – review and editing; W. Shen: validation; W. Zhang: formal analysis; J. Liu: supervision, writing – review and editing, funding acquisition.
Conflicts of interest
There are no conflicts to declare.
Acknowledgements
This work was supported by the National Natural Science Foundation of China (81971731 and 82172082), CAMS Innovation Fund for Medical Sciences (2021-I2M-1-042), the National Science Fund for Distinguished Young Scholars of Tianjin (18JCJQJC47300), and the Non-profit Central Research Institute Fund of Chinese Academy of Medical Sciences (2018PT35031).
References
- H. Sung, J. Ferlay, R. L. Siegel, M. Laversanne, I. Soerjomataram, A. Jemal and F. Bray, CA Cancer J. Clin., 2021, 71, 209–249 CrossRef.
- N. Harbeck, F. Penault-Llorca, J. Cortes, M. Gnant, N. Houssami, P. Poortmans, K. Ruddy, J. Tsang and F. Cardoso, Nat. Rev. Dis. Primers, 2019, 5, 66 CrossRef.
- Y. Xie, F. Xie, L. Zhang, X. Zhou, J. Huang, F. Wang, J. Jin, L. Zhang, L. Zeng and F. Zhou, Adv. Sci., 2021, 8, e2101672 CrossRef PubMed.
- D. De Ruysscher, G. Niedermann, N. G. Burnet, S. Siva, A. W. M. Lee and F. Hegi-Johnson, Nat. Rev. Dis. Primers, 2019, 5, 13 CrossRef PubMed.
- L. Cai, J. Xu, Z. Yang, R. Tong, Z. Dong, C. Wang and K. W. Leong, MedComm, 2020, 1, 35–46 CrossRef.
- W. Ma, Q. Chen, W. Xu, M. Yu, Y. Yang, B. Zou, Y. S. Zhang, J. Ding and Z. Yu, Nano Res., 2021, 14, 846–857 CrossRef CAS.
- G. Chen, Y. Yang, Q. Xu, M. Ling, H. Lin, W. Ma, R. Sun, Y. Xu, X. Liu, N. Li, Z. Yu and M. Yu, Nano Lett., 2020, 20, 8141–8150 CrossRef CAS.
- Y. Yang, Y. Yu, H. Chen, X. Meng, W. Ma, M. Yu, Z. Li, C. Li, H. Liu, X. Zhang, H. Xiao and Z. Yu, ACS Nano, 2020, 14, 13536–13547 CrossRef CAS.
- X. Deng, Z. Shao and Y. Zhao, Adv. Sci., 2021, 8, 2002504 CrossRef CAS.
- X. Li, J. F. Lovell, J. Yoon and X. Chen, Nat. Rev. Clin. Oncol., 2020, 17, 657–674 CrossRef.
- J. Li and K. Pu, Acc. Chem. Res., 2020, 53, 752–762 CrossRef CAS.
- H. Jeong, W. Park, D. H. Kim and K. Na, Adv. Drug Delivery Rev., 2021, 177, 113954 CrossRef CAS PubMed.
- Y. Liu, P. Bhattarai, Z. Dai and X. Chen, Chem. Soc. Rev., 2019, 48, 2053–2108 RSC.
- Y. Wang, N. Gong, Y. Li, Q. Lu, X. Wang and J. Li, J. Am. Chem. Soc., 2020, 142, 1735–1739 CrossRef CAS.
- S. Liu, X. Zhou, H. Zhang, H. Ou, J. W. Y. Lam, Y. Liu, L. Shi, D. Ding and B. Z. Tang, J. Am. Chem. Soc., 2019, 141, 5359–5368 CrossRef CAS PubMed.
- S. Wenger, Nat. Chem., 2020, 12, 323–324 CrossRef.
- D. Chen, Q. Xu, W. Wang, J. Shao, W. Huang and X. Dong, Small, 2021, 17, e2006742 CrossRef.
- D. Tao, L. Feng, Y. Chao, C. Liang, X. Song, H. Wang, K. Kang and Z. Liu, Adv. Funct. Mater., 2018, 28, 1804901 CrossRef.
- Q. Wu, G. Chen, K. Gong, J. Wang, X. Ge, X. Liu, S. Guo and F. Wang, Matter, 2019, 1, 496–512 CrossRef.
- M. Yu, F. Guo, J. Wang, F. Tan and N. Li, ACS Appl. Mater. Interfaces, 2015, 7, 17592–17597 CrossRef CAS PubMed.
- B. Liu, J. Jiao, W. Xu, M. Zhang, P. Cui, Z. Guo, Y. Deng, H. Chen and W. Sun, Adv. Mater., 2021, 33, e2100795 CrossRef PubMed.
- W. Fan, B. Yung, P. Huang and X. Chen, Chem. Rev., 2017, 117, 13566–13638 CrossRef CAS.
- W. Shao, C. Yang, F. Li, J. Wu, N. Wang, Q. Ding, J. Gao and D. Ling, Nano-Micro Lett., 2020, 12, 147 CrossRef CAS.
- J. Huang, B. He, Z. Zhang, Y. Li, M. Kang, Y. Wang, K. Li, D. Wang and B. Z. Tang, Adv. Mater., 2020, 32, e2003382 CrossRef PubMed.
- J. Yin, X. Jiang, G. Sui, Y. Du, E. Xing, R. Shi, C. Gu, X. Wen, Y. Feng, Z. Shan and S. Meng, J. Mater. Chem. B, 2021, 9, 7461–7471 RSC.
- K. Zhang, X. Meng, Y. Cao, Z. Yang, H. Dong, Y. Zhang, H. Lu, Z. Shi and X. Zhang, Adv. Funct. Mater., 2018, 28, 1804634 CrossRef.
- X. Mu, Y. Lu, F. Wu, Y. Wei, H. Ma, Y. Zhao, J. Sun, S. Liu, X. Zhou and Z. Li, Adv. Mater., 2020, 32, e1906711 CrossRef.
- D. Mao, W. Wu, S. Ji, C. Chen, F. Hu, D. Kong, D. Ding and B. Liu, Chem, 2017, 3, 991–1007 CAS.
- Y. Li, G. Liu, J. Ma, J. Lin, H. Lin, G. Su, D. Chen, S. Ye, X. Chen, X. Zhu and Z. Hou, J. Controlled Release, 2017, 258, 95–107 CrossRef CAS.
- S. Guo, K. Li, B. Hu, C. Li, M. Zhang, A. Hussain, X. Wang, Q. Cheng, F. Yang, K. Ge, J. Zhang, J. Chang, X. Liang, Y. Weng and Y. Huang, Exploration, 2021, 1, 35–49 CrossRef.
- C. Ren, Z. Wang, Q. Wang, C. Yang and J. Liu, Small Methods, 2020, 4, 1900403 CrossRef CAS.
- N. Song, Z. Zhang, P. Liu, D. Dai, C. Chen, Y. Li, L. Wang, T. Han, Y.-W. Yang, D. Wang and B. Z. Tang, Adv. Funct. Mater., 2021, 31, 2009924 CrossRef CAS.
- X. Shu, Y. Chen, P. Yan, Y. Xiang, Q.-Y. Shi, T. Yin, P. Wang, L.-H. Liu and X. Shuai, J. Controlled Release, 2022, 347, 270–281 CrossRef CAS.
- T. He, J. He, M. R. Younis, N. T. Blum, S. Lei, Y. Zhang, P. Huang and J. Lin, ACS Appl. Mater. Interfaces, 2021, 13, 22204–22212 CrossRef CAS.
- Y. Lai, Y. Dang, F. Li, C. Ding, H. Yu, W. Zhang and Z. Xu, Adv. Funct. Mater., 2022, 32, 2200016 CrossRef CAS.
- C. Li, E. Mei, C. Chen, Y. Li, B. Nugasur, L. Hou, X. Ding, M. Hu, Y. Zhang, Z. Su, J. Lin, Y. Yang, P. Huang and Z. Li, ACS Appl. Mater. Interfaces, 2020, 12, 12541–12548 CrossRef CAS PubMed.
- L. Ding, Z. Lyu, B. Louis, A. Tintaru, E. Laurini, D. Marson, M. Zhang, W. Shao, Y. Jiang, A. Bouhlel, L. Balasse, P. Garrigue, E. Mas, S. Giorgio, J. Iovanna, Y. Huang, S. Pricl, B. Guillet and L. Peng, Small, 2020, 16, e2003290 CrossRef.
- Z. Li, Y. Hu, Z. Miao, H. Xu, C. Li, Y. Zhao, Z. Li, M. Chang, Z. Ma, Y. Sun, F. Besenbacher, P. Huang and M. Yu, Nano Lett., 2018, 18, 6778–6788 CrossRef CAS PubMed.
- X. Zhang, M. Zhao, L. Wen, M. Wu, Y. Yang, Y. Zhang, Y. Wu, J. Zhong, H. Shi, J. Zeng, G. Wang and M. Gao, Biomater. Sci., 2021, 9, 3069–3075 RSC.
- Y. Yu, Z. Zhang, Y. Wang, H. Zhu, F. Li, Y. Shen and S. Guo, Acta Biomater., 2017, 59, 170–180 CrossRef CAS.
- J. Fang, W. Islam and H. Maeda, Adv. Drug Delivery Rev., 2020, 157, 142–160 CrossRef CAS PubMed.
- Y. Sun, Y. Wang, Y. Liu, B. Weng, H. Yang, Z. Xiang, J. Ran, H. Wang and C. Yang, ACS Appl. Mater. Interfaces, 2021, 13, 53646–53658 CrossRef CAS.
- M. Zhang, X. Qin, W. Xu, Y. Wang, Y. Song, S. Garg and Y. Luan, J. Colloid Interface Sci., 2021, 594, 493–501 CrossRef CAS PubMed.
- Y. Li, Y. Wu, J. Chen, J. Wan, C. Xiao, J. Guan, X. Song, S. Li, M. Zhang, H. Cui, T. Li, X. Yang, Z. Li and X. Yang, Nano Lett., 2019, 19, 5806–5817 CrossRef CAS.
- R. Xing, Q. Zou, C. Yuan, L. Zhao, R. Chang and X. Yan, Adv. Mater., 2019, 31, e1900822 CrossRef PubMed.
- D. Sheng, T. Liu, L. Deng, L. Zhang, X. Li, J. Xu, L. Hao, P. Li, H. Ran, H. Chen and Z. Wang, Biomaterials, 2018, 165, 1–13 CrossRef CAS.
- P. Xue, R. Yang, L. Sun, Q. Li, L. Zhang, Z. Xu and Y. Kang, Nano-Micro Lett., 2018, 10, 74 CrossRef CAS PubMed.
- H. Wang, X. Li, B. W. Tse, H. Yang, C. A. Thorling, Y. Liu, M. Touraud, J. B. Chouane, X. Liu, M. S. Roberts and X. Liang, Theranostics, 2018, 8, 1227–1242 CrossRef CAS PubMed.
- Y. Chen, Y. Li, J. Liu, Q. Zhu, J. Ma and X. Zhu, J. Controlled Release, 2021, 335, 345–358 CrossRef CAS PubMed.
- W. Shan, R. Chen, Q. Zhang, J. Zhao, B. Chen, X. Zhou, S. Ye, S. Bi, L. Nie and L. Ren, Adv. Mater., 2018, 30, e1707567 CrossRef.
- L. Yang, B. Huang, S. Hu, Y. An, J. Sheng, Y. Li, Y. Wang and N. Gu, Nano Res., 2022, 15, 4285–4293 CrossRef CAS.
- R. Clanton, A. Gonzalez, S. Shankar and G. Akabani, Appl. Radiat. Isot., 2018, 131, 49–57 CrossRef CAS PubMed.
- P. J. Gawne, F. Man, P. J. Blower and R. T. M. de Rosales, Chem. Rev., 2022, 122, 10266–10318 CrossRef CAS PubMed.
- J. Pellico, P. J. Gawne and R. T. M. de Rosales, Chem. Soc. Rev., 2021, 50, 3355–3423 RSC.
- C. Zhang, G. Shi, J. Zhang, J. Niu, P. Huang, Z. Wang, Y. Wang, W. Wang, C. Li and D. Kong, Nanoscale, 2017, 9, 3304–3314 RSC.
- J. Liu, Y. Zhang, Q. Li, Z. Feng, P. Huang, W. Wang and J. Liu, Acta Biomater., 2020, 114, 133–145 CrossRef CAS PubMed.
- L. Yang, C. Zhang, J. Liu, F. Huang, Y. Zhang, X.-J. Liang and J. Liu, Adv. Healthcare Mater., 2020, 9, e1901616 CrossRef PubMed.
- L. Yang, X. Hou, Y. Zhang, D. Wang, J. Liu, F. Huang and J. Liu, J. Controlled Release, 2021, 339, 114–129 CrossRef CAS.
- L. Yu, Z. Wang, Z. Mo, B. Zou, Y. Yang, R. Sun, W. Ma, M. Yu, S. Zhang and Z. Yu, Acta Pharm. Sin. B, 2021, 11, 2004–2015 CrossRef CAS.
- M. He, L. Yu, Y. Yang, B. Zou, W. Ma, M. Yu, J. Lu, G. Xiong, Z. Yu and A. Li, Chin. Chem. Lett., 2020, 31, 3178–3182 CrossRef CAS.
- Y. Yang, X. Liu, W. Ma, Q. Xu, G. Chen, Y. Wang, H. Xiao, N. Li, X.-J. Liang, M. Yu and Z. Yu, Biomaterials, 2021, 265, 120456 CrossRef CAS.
- Y. Liang, X. Deng, L. Zhang, X. Peng, W. Gao, J. Cao, Z. Gu and B. He, Biomaterials, 2015, 71, 1–10 CrossRef CAS PubMed.
- V. Mundra, Y. Peng, S. Rana, A. Natarajan and R. I. Mahato, J. Controlled Release, 2015, 220, 130–140 CrossRef CAS PubMed.
- W. Holzer, M. Mauerer, A. Penzkofer, R.-M. Szeimies, C. Abels, M. Landthaler and W. Bäumler, J. Photochem. Photobiol., B, 1998, 47, 155–164 CrossRef CAS PubMed.
- J. J. Rennick, A. P. R. Johnston and R. G. Parton, Nat. Nanotechnol., 2021, 16, 266–276 CrossRef CAS PubMed.
- H. J. Eom and J. Choi, Chem.-Biol. Interact., 2019, 311, 108774 CrossRef CAS PubMed.
- Q. Chen, C. Liang, C. Wang and Z. Liu, Adv. Mater., 2015, 27, 903–910 CrossRef CAS PubMed.
|
This journal is © The Royal Society of Chemistry 2023 |
Click here to see how this site uses Cookies. View our privacy policy here.