DOI:
10.1039/D3BM00544E
(Review Article)
Biomater. Sci., 2023,
11, 6035-6059
Immune homeostasis modulation by hydrogel-guided delivery systems: a tool for accelerated bone regeneration
Received
2nd April 2023
, Accepted 9th July 2023
First published on 31st July 2023
Abstract
Immune homeostasis is delicately mediated by the dynamic balance between effector immune cells and regulatory immune cells. Local deviations from immune homeostasis in the microenvironment of bone fractures, caused by an increased ratio of effector to regulatory cues, can lead to excessive inflammatory conditions and hinder bone regeneration. Therefore, achieving effective and localized immunomodulation of bone fractures is crucial for successful bone regeneration. Recent research has focused on developing localized and specific immunomodulatory strategies using local hydrogel-based delivery systems. In this review, we aim to emphasize the significant role of immune homeostasis in bone regeneration, explore local hydrogel-based delivery systems, discuss emerging trends in immunomodulation for enhancing bone regeneration, and address the limitations of current delivery strategies along with the challenges of clinical translation.
1 Introduction
Bones serve multiple crucial roles in the human body, including movement, support, and protection, as well as hematopoiesis and the storage of minerals like calcium and phosphorus. Therefore, maintaining the structural and functional integrity of bones is vital for our overall quality of life. Fractures often occur as a result of significant traumatic injuries, but fortunately, bones possess a robust regenerative capacity. Unlike many other tissues, bones have the remarkable ability to heal, allowing them to regain their biological composition and nearly normal function. This extraordinary regenerative capability may be attributed to the reactivation of cellular and molecular processes observed during embryonic bone formation, which are recapitulated during the healing of fractures.1,2
However, despite advancements, approximately 10% of nonunion fracture cases still occur, significantly diminishing patients’ quality of life and imposing a substantial financial burden on their families.3 Therefore, conducting sustainable and in-depth studies on fracture healing mechanisms is still necessary, which can be divided into two categories. Firstly, severe trauma and excessive inflammation can result in significant bone defects that exceed the regenerative capacity of bone tissue. Secondly, disruptions in the body's immune system balance can partially impair the original regenerative ability of bone tissue. In both cases, the aforementioned excessive inflammation and immune system imbalances emphasize the crucial role of maintaining immune homeostasis during fracture healing, leading to an increased research focus on regulating immune reconstruction for bone repair. The interrelationship between the skeletal system and the immune system has long been recognized. On one hand, bone tissue serves as the main hematopoietic organ and the common origin of most immune cells, creating a similar microenvironment shared by bone cells and immune cells in the early stages. On the other hand, immune cell participation plays an essential role in the physiological and pathological processes of bone tissue, including cartilage formation, bone turnover, and fracture healing.4 Consequently, a significant clinical challenge lies in efficiently, stably, and specifically regulating local immune homeostasis at the fracture site.
Bone transplantation, the traditional surgical treatment method in clinical practice, is associated with well-known drawbacks. Autologous bone grafting leads to increased morbidity at the resection site, while allogeneic bone grafting raises the risk of immune rejection and disease transmission. Moreover, these procedures are painful, expensive, and require additional surgeries. As a result, researchers began exploring biomaterials as potential bone substitutes. Initially, the focus was primarily on the low toxicity and biocompatibility of the biomaterials themselves. Biomaterials used for constructing bone tissue to repair defects can be classified into metals, ceramics, polymers, and composite materials, such as titanium alloys, silica, hydroxyapatite, and collagen, based on their chemical composition. Building upon this foundation, the concept of bone tissue engineering emerged as an attractive idea, aiming to use biomaterials to combine and control the release of active factors, hormones, drugs, and stem cells, thus inducing a favorable cellular response and enhancing the natural healing ability of the recipient tissue. This approach poses higher requirements for biomaterials, including their ability to incorporate biologically active substances, deliver drugs, carry cells, and effectively release the cargo.5,6 Among various biomaterials, hydrogels have garnered significant attention. Hydrogels can closely mimic the natural extracellular matrix (ECM), providing an ideal environment for cell encapsulation, seeding, and homogenization. They can also serve as scaffolds for the controlled delivery of growth factors.7 Hydrogels are polymer matrices containing cross-linked polymer chains and a substantial amount of water. They possess controllable mechanical properties and biocompatibility, making them versatile materials suitable for various applications such as immunomodulation, drug delivery, and tissue engineering. By modifying hydrogels with natural polymers or synthetic compounds, it is possible to tailor their biophysical and biochemical properties to support different cell types and optimize interactions, including cell adhesion, microstructure, and degradability.8,9 Despite the inherent trade-off between mechanical and biological properties, with mechanical properties and biocompatibility inversely correlated with polymer concentration, hydrogels show strong potential for clinical applications. They are user-friendly, cost-effective, and readily available materials that have already found use in various areas of clinical practice, including spinal surgery and wound dressings.10,11 It is foreseeable that in the future, more suitable hydrogels will be employed in bone tissue engineering, targeted drug delivery, and disease treatment.
In recent years, hydrogels have gained widespread attention for their ability to modulate immune microenvironments in various diseases, including cancer, cardiovascular conditions, and wound repair.12,13 These promising findings have led to an increasing focus on hydrogel-based immunomodulation strategies for bone repair. Examples of such strategies include dual-logic hydrogels with diagnostic and therapeutic functionalities, as well as titanium hydrogels modified with ROS-responsive coatings.14,15 However, it is worth noting that most of the existing studies are still in the experimental stage and have not yet been translated into clinical treatments. Therefore, this article aims to explore the changes in the immune microenvironment during bone repair, discuss hydrogel systems utilized for immune microenvironment modulation, and introduce relevant novel materials. The goal is to provide researchers with new perspectives for developing innovative bone repair strategies that leverage immune micro-regulation and to outline potential directions for future research in this field.
2 The prominent role of immune homeostasis in bone regeneration
Bone and the immune system have a close relationship, as several types of immune cell, including macrophages, T cells, and B cells, undergo differentiation or maturation in the bone marrow.16 The term “bone immunology” was first introduced by Arron, J. R., and Choi, Y. in 2000 to describe the molecular mechanisms by which T cells mediate bone loss.17 Nowadays, “bone immunology” refers to the study of the physiological and pathological interactions between the immune system and bone.18
The immune system plays an indispensable role in fracture healing, as multiple immune cells collaborate to regulate immune homeostasis at the site of the fracture, thereby facilitating the healing process (Fig. 1). The immune response during fractures is complex and still not fully comprehended by researchers. Since fractures can involve the contact of damaged bone tissue with the external environment, the repair process often encompasses both aseptic and bacterial inflammation. From this perspective, the immune response in fracture repair is even more intricate compared with the heart, which is one of the body's most delicate organs and typically experiences aseptic inflammation exclusively.19 In the next sections, we will delve into the various immune cell subtypes that play pivotal roles in fracture healing (Table 1).
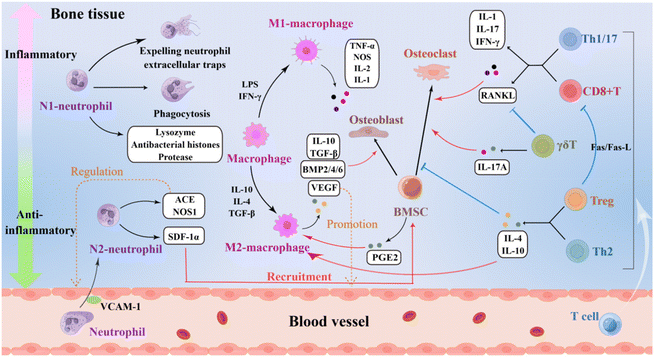 |
| Fig. 1 The dynamic crosstalk between immune cells and tissue functional cells during the process of fracture repair. In response to a fracture, various immune cells are recruited to the site of the injury and undergo coordinated activation. This coordinated activation aims to establish a pro-repair immune microenvironment that supports osteogenic formation and promotes osteoblastic differentiation, thereby facilitating fracture regeneration. | |
Table 1 The classification and function of immune cells during fracture repair
Classification |
Types |
Functional |
ACE: angiotensin-converting enzyme, NOS1: nitric oxide synthase 1, BMSCs: bone marrow-derived mesenchymal stem cells, SDF-1: stromal cell-derived factor 1, CXCR1: C-X-C chemokine receptor 1, IFN-γ: interferon-gamma, IL-17: interleukin-17, Fas-L: fas ligand, RANK: receptor activator of nuclear factor kappa-B, RANKL: RANK ligand, OPG: osteoprotegerin, γδT: gamma delta T cells, NA: not applicable. |
Macrophages |
M1 |
(a) Promote inflammatory processes during the initial stages of fracture healing.24 |
(b) Help to limit pathogen growth, invasion, and clearance of infection.24 |
M2 |
Secrete anti-inflammatory cytokines to reduce inflammation during the middle and late stages of fracture healing.25 |
Neutrophils |
N1 |
Exert pro-inflammatory effects.41 |
N2 |
(a) Exert direct anti-inflammatory effects by modulating vascular factors such as ACE and NOS1.42 |
(b) Recruiting BMSCs to the fracture through the SDF-1/CXCR1 axis.43 |
Mast cells |
NA |
Coordinating the catabolic activity, re-vascularization, and overall healing process of fractures.49,50 |
CD8+ T cells |
NA |
Contribute to trabecular bone loss.52 |
CD4+ T cells |
Th1/17 cells |
Both IFN-γ (primarily secreted by Th1 cells) and IL-17 (mainly secreted by Th17 cells) can promote osteoclastogenesis.56 |
Treg cells |
(a) Inhibit inflammation and elevate MSCs activity.57 |
(b) Regulate neutrophil activity, macrophage polarization, and the cytotoxicity of other cells via pathways involving perforin, granzyme, or Fas-L.58,59 |
γδT cells |
NA |
(a) Promote bone repair through the secretion of IL-17, which stimulates the proliferation of mesenchymal progenitor cells and the differentiation of osteoblasts.63,64 |
(b) Exert inhibitory effects on osteoclastogenesis by downregulating receptor activator of RANKL.65 |
B cells |
NA |
Influence on osteoclast maturation through regulation of the RANK/RANKL/OPG axis.67,68 |
2.1 Macrophages and fracture repair
Tissue-resident macrophages are the primary immune cells that respond to fracture events. These macrophages, derived from monocytes and residing in the tissue, act as immune sentinels and play a crucial role in nonspecific immune regulation. For instance, microglia, the resident immune cells in the brain, surveil and protect the brain from damage, infection, and abnormal cellular activity, while also contributing to neural development and maintaining overall brain homeostasis. In the lung, alveolar macrophages diligently monitor inhaled pathogens and ensure tissue homeostasis by facilitating surfactant clearance. Also, the specific intestinal macrophages are responsible for surveying the colon for invading pathogens, maintaining immune tolerance to the resident microbiota, and regulating inflammation to ensure the balance of the intestinal environment.20 Within the bone and bone marrow, various macrophage populations exist, such as osteoclasts responsible for bone resorption, erythroid island macrophages supporting erythropoiesis, and osteal macrophages located near bone-forming cells. The functional role of osteal macrophages in bone formation is currently under investigation.20 In the normal bone environment, the majority of macrophages are quiescent tissue-resident macrophages (TRMs) that contribute to maintaining balance and stability through controlled self-renewal. When inflammation is stimulated, hematopoietic stem cells (HSCs) can be activated, leading to increased myeloid differentiation and the circulation of monocytes into bone tissue. The niche of resident macrophages can be selectively activated based on the level of inflammation, with monocyte-derived pro-inflammatory macrophages occupying available niches to preserve homeostasis. However, in cases of diabetes, an increased number of available niches are occupied by pro-inflammatory macrophages, resulting in a prolonged pro-inflammatory state within the bone regeneration microenvironment (Fig. 2).21 While the origins of macrophages may vary under specific conditions, their contribution throughout the entire healing process is irreplaceable. Depletion of macrophages during the early stages of a fracture may lead to reduced callus formation, which could be attributed to the involvement of macrophages in early cartilage mediation. On the other hand, depletion of macrophages during the later stages of fractures may delay bone healing, potentially due to the role of macrophages in cytokine secretion.22,23
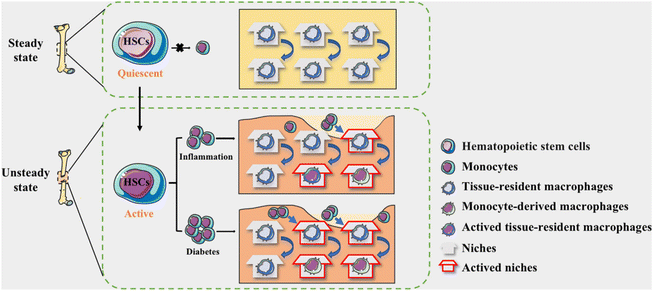 |
| Fig. 2 Macrophages' niche in the bone tissue. Under specific conditions, macrophages occupy specific niches within bone tissues. The macrophages are mostly inactive in the physiological bone niche and maintain homeostasis through moderate self-proliferation. HSCs can activate by the inflammation stimulation and this allows monocytes to circulate into bone tissue. Based on the degree of inflammation, the niche of TRMs can activate and monocyte-derived pro-inflammatory macrophages occupy available niches to maintain homeostasis. Adapted with permission.21 Copyright 2022, Frontiers. | |
The heterogeneity and polarization of macrophages have been widely recognized. In response to external stimuli, macrophages can be polarized into two functional subgroups: M1-like and M2-like macrophages. M1 macrophages are primarily activated by substances like LPS and IFN-γ. They secrete elevated levels of cytokines such as TNF-α, iNOS, IL-2, and IL-1α/β. These cytokines promote inflammatory processes during the initial stages of fracture healing, helping to limit pathogen growth, invasion, and clearance of infection. On the other hand, M2 macrophages are primarily activated by IL-10 and IL-4. They secrete high levels of anti-inflammatory cytokines such as IL-10 and TGF-β. These anti-inflammatory cytokines play a role in reducing inflammation during the middle and late stages of fracture healing, thereby facilitating tissue repair.
The regulation of inflammation in vivo, specifically to promote steady or subsiding inflammation, can be achieved through modulation of the M1/M2 macrophage ratio. Decreasing the M1/M2 ratio leads to the suppression of inflammation, which may be attributed to the secretion of anti-inflammatory cytokines by immunomodulatory cells.24 Conversely, when there is a need for inflammatory progression, M2 macrophages can differentiate into osteoclasts, thereby increasing the M1/M2 ratio and promoting the existence and development of inflammation.25 It should be noted that the classification of macrophages into M1 and M2 is not strictly polarized, and there is overlap in cytokine production. For instance, while the key anti-inflammatory factor IL-10 is predominantly secreted by M2 macrophages, small amounts can also be secreted by M1 macrophages.26
Under certain pathological conditions, an imbalance in the M1/M2 macrophage ratio can negatively impact bone healing. For instance, in diabetes, advanced glycation end products (AGEs) can activate the PPAR-γ/NF-κB pathway, leading to the polarization of M1 macrophages and an imbalance in the M1/M2 ratio. This can be detrimental to fracture healing. AGEs also affect the osteogenic differentiation of mesenchymal stem cells through DNA metabolism and the Wnt signaling pathway.27 Furthermore, studies have shown that adjusting the local M1/M2 macrophage ratio can accelerate fracture healing. Researchers have explored strategies such as administration of adrenomedullary 2 (ADM2) to counter the polarization imbalance of macrophages induced by AGEs in diabetic mice, thereby enhancing bone regeneration.28 Additionally, modulation of macrophage activity using biotic metallic ions has been investigated to coordinate the immune response in the bone tissue microenvironment and promote bone healing.29,30
In addition to immune function, macrophages play a role in bone formation by secreting cytokines and extracellular vesicles (EVs). For example, they secrete BMP2/4/6, which promotes osteogenesis through signaling pathways such as Smad and P38-MAPK.31,32 Macrophages also promote the differentiation of mesenchymal stem cells into osteoblasts and enhance angiogenesis in the fracture region through the secretion of VEGF, contributing to callus remodeling.33,34 The use of macrophage-derived EVs to promote endogenous osteogenesis has been reported, while the presence of miR144-5p in EVs from bone marrow-derived macrophages in type 2 diabetes has been found to hinder fracture healing by targeting Smad1.35 Lei et al. has proposed two strategies for bone tissue engineering that involve regulating macrophage secretion function. These include co-culturing macrophages and bone progenitor cells in vitro before transplantation and designing polymers to regulate macrophage activity in situ. Both approaches aim to provide a continuous supply of cytokines and EVs throughout fracture healing to promote the bone repair process.36
2.2 Neutrophils and fracture repair
In addition to resident macrophages within the bone tissue, fractures induce an inflammatory environment that attracts a significant influx of granulocytes, particularly neutrophils. Neutrophils, the most abundant immune cells in the body, are rapidly recruited to the site of injury during pathogenic invasion and various inflammatory conditions.37 Vascular endothelial cells at the fracture site express vascular cell adhesion molecule-1 (VCAM-1), which facilitates the recruitment of neutrophils from surrounding tissues, resulting in a high concentration of neutrophils in fracture hematomas.38 Furthermore, neutrophils play a critical role in tissue remodeling and pathogen elimination through processes such as phagocytosis, release of extracellular neutrophilic traps (NETs), and secretion of lysozyme, antimicrobial histones, and proteases.39 Previous studies have demonstrated that impairment of neutrophil function, as observed in a mouse fracture model with down-regulated neutrophil activity, significantly hinders bone regeneration, underscoring the pivotal role of neutrophils in the fracture healing process.40
The concept of neutrophil heterogeneity initially emerged in the field of oncology, but recent investigations have revealed their heterogeneity in the context of inflammation. Neutrophils can be classified into two subtypes based on their impact on inflammation: pro-inflammatory neutrophils (N1) with tumor-suppressive effects and anti-inflammatory neutrophils (N2) with tumor-promoting effects.41 This classification framework is also applicable to studies focusing on bone regeneration. N2 neutrophils exert direct anti-inflammatory effects by modulating vascular factors such as ACE and NOS1.42 Additionally, N2 neutrophils play a crucial role in recruiting bone marrow-derived mesenchymal stem cells (BMSCs) to the fracture site. Cai et al. reported induced ectopic endochondral ossification using IL-8 and found a resemblance to the early stage of fracture hematoma. During ectopic endochondral ossification, neutrophils are recruited and polarized into the N2 phenotype, leading to the secretion of SDF-1 (stromal cell-derived factor-1). Through the SDF-1/CXCR1 axis and downstream signaling pathways such as PI3K/Akt and β-catenin, BMSC chemotaxis is induced.43 Another study by the Bastian et al. involved the analysis of human fracture hematomas (FHs) at different time points following bone injury. Within the first 48 hours, FHs exhibited an extracellular matrix rich in fibronectin and neutrophil-derived particles, while stromal cells were only detected on the fifth day post-injury. This finding suggests that neutrophils can synthesize an “emergency extracellular matrix” during the early stages of bone injury, serving as a provisional scaffold for traditional bone repair and promoting subsequent bone regeneration.
In addition to their well-known functions, neutrophils also contribute to the recruitment of monocytes. Depletion of neutrophils in experimental settings has been shown to decrease the recruitment of inflammatory mononuclear cells. The FPR ligand LL-37 has been identified as the primary mediator of this neutrophil-mediated monocyte recruitment.44 Activated neutrophils are capable of secreting CC-chemokines, including CCL3 and CCL4, which bind to CCR1 receptors predominantly expressed on monocytes.45 Furthermore, neutrophils release protease 3 (PR3) that can activate adjacent cells, particularly endothelial cells, triggering the secretion of chemokines and establishing a microenvironment favorable for monocyte recruitment. PR3 is believed to upregulate intercellular adhesion molecule-1 (ICAM-1), which in turn increases the production of monocyte chemoattractant protein-1 (MCP-1) and facilitates the adhesion of neutrophils to endothelial cells.46 Consequently, neutrophils, through the secretion of PR3, may enhance their adhesion to endothelial cells, thus promoting sustained inflammation and providing continuous chemotactic stimulation for monocytes.
2.3 Eosinophils and basophils and fracture repair
Limited research has focused on eosinophils and basophils within the context of bone regeneration, with the majority of studies emphasizing the role of tissue-resident mast cells (MCs) in fracture healing. MCs, a type of basophilic granulocyte, are primarily recognized for their involvement in mediating allergic reactions in vivo. Following a fracture event, MCs have been observed to accumulate, activate, undergo degranulation, and contribute to nociceptive sensitization.47 Although the precise molecular mechanisms underlying the MCs’ involvement in fracture healing remain unclear, their role is believed to be associated with inflammation.48 Overall, MCs have a positive impact on fracture healing, as evidenced by experiments conducted on mice with MC deficiencies, such as KitW-sh and Cpa3Cre/+ mice.49,50 However, in estrogen-deficient conditions, MCs exert a negative influence on bone repair, as MC-deficient mice have been shown to be resistant to ovariectomy-induced bone loss. This phenomenon is likely linked to MC-mediated hyper-inflammation and allergic reactions.51
2.4 T cell and fracture repair
T lymphocytes are responsible for mediating specific immunity, can be classified based on their surface molecules and exhibit varying effects on fracture healing.
CD8+ T cells, also known as cytotoxic T cells, possess the ability to release perforin and granzymes, and activate FAS receptors, thereby inducing apoptosis in target cells. Despite the limited research conducted on the role of CD8+ T cells in bone regeneration, conflicting results have prompted further exploration in this field. Weitzmann et al. reported that T cell receptor knockout experiments conducted in mice demonstrated that CD8+ T cells contribute to trabecular bone loss.52 However, a contrary outcome was observed by Bernhardsson et al. Their experiments involved depleting the corresponding T cells through the injection of CD8 antibodies in rats, revealing an active role for CD8+ cells in cancellous bone healing.53 These conflicting conclusions may be attributed to the use of different animal models in the experiments. Moreover, maintaining a balanced T cell population appears to be a crucial regulator of fracture healing, as an excessively elevated ratio of CD8+ effector T cells to CD4+ regulatory T cells can impair the process of fracture rebuilding (Fig. 3A).54
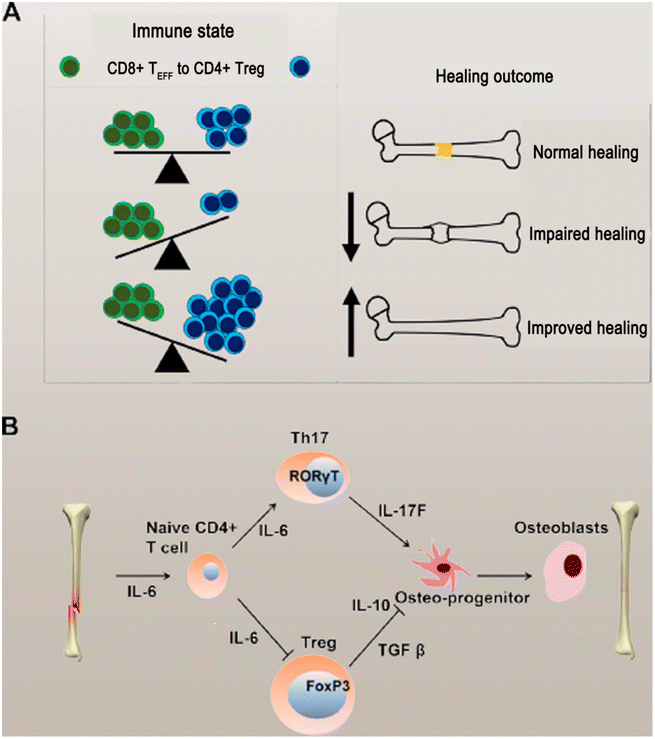 |
| Fig. 3 The significant involvement of T cells in fracture repair. (A) The interrelationship between immune status and the outcome of healing following bone fracture. The balance between CD8+ effector T cells (CD8+ TEFF) and CD4+ regulatory T cells (CD4+ Treg) influences the healing outcome. Adapted with permission.54 Copyright 2019, Frontiers. (B) Proposed mechanistic model illustrating the role of T cells in mediating osteoblast differentiation and maturation. Adapted with permission.55 Copyright 2012, PLOS. | |
Helper T cells play diverse roles in the process of fracture repair, with particular attention given to Th1/17/Treg cells (Fig. 3B).55 Studies have demonstrated that both IFN-γ (primarily secreted by Th1 cells) and IL-17 (mainly secreted by Th17 cells) can promote osteoclastogenesis, as evidenced by the upregulation of related mRNA and protein levels.56 The contribution of Treg cells is indispensable for maintaining self-tolerance and preventing excessive inflammation. Through various pathways, Treg cells inhibit inflammation and promote bone regeneration, particularly by leveraging the capabilities of bone mesenchymal stem cells, thus facilitating the repair and remodeling process following a fracture.57 Mechanistically, Treg cells exert their modulatory effects on neutrophil activity, macrophage polarization, and the cytotoxicity of other cells via pathways involving perforin, granzyme, or Fas-L.58,59 In addition to their anti-inflammatory role in promoting bone regeneration, Treg cells can secrete cytokines such as IL-4 and TGF-β, which inhibit osteoclast differentiation.60,61
γδT cells, a distinct subset of T cells, exhibit innate immune properties and possess diverse biological functions including antigen presentation, cytotoxicity, and immune regulation, making them crucial in bridging innate and adaptive immunity.62 Researchers have also focused on the role of γδT cells in the context of fracture healing. These cells can facilitate bone repair through the secretion of interleukin-17 (IL-17). Experimental evidence demonstrates the proliferation of γδT cells at the site of bone injury, accompanied by the secretion of IL-17A. Additionally, mice deficient in IL-17A exhibit impaired bone repair.63,64 This mechanism is linked to the ability of IL-17A to stimulate the proliferation of mesenchymal progenitor cells and the differentiation of osteoblasts. Moreover, γδT cells may exert inhibitory effects on osteoclastogenesis by downregulating receptor activator of nuclear factor kappa-B ligand (RANKL), thus exerting regulatory control over bone repair.65 While the mechanisms underlying these two pathways have been validated, further investigation is warranted to explore additional pathways through which γδT cells modulate bone repair.
2.5 B cells and fracture repair
B lymphocytes, derived from the bone marrow, are essential cells involved in specific immunity, primarily responsible for mediating humoral immune responses in the body. In the context of fracture healing, there are distinct spatiotemporal variations in the abundance of B cells within the bone tissue, indirectly suggesting their involvement in this process.66 B cells exert their influence on osteoclast maturation through regulation of the RANK/RANKL/OPG axis, thus participating in fracture healing. Moreover, OPG, acting as a decoy receptor for RANKL, indirectly inhibits RANK signaling.67,68 Notably, recent studies have revealed that B cells contribute to a significant portion of OPG production in the bone marrow, accounting for 64% of the total OPG production. This substantial OPG secretion by B cells enables the antagonistic effect of OPG on RANK, thereby impeding osteoclast maturation.69 The physiological effects of OPG have been further substantiated, as its overexpression leads to osteosclerosis, while OPG deficiency results in osteoporosis symptoms.70,71 Despite the significant attention B cells have received in the study of lymphoma-associated tumor diseases, their role in fracture healing remains relatively understudied, necessitating further exploration of the underlying mechanisms.72
Apart from immune cells, there exists another cell type that, although not directly engaged in immune responses, plays a vital role in regulating immune homeostasis during the process of fracture healing. These cells are known as mesenchymal stem cells (MSCs).73 Upon attraction by chemokines such as CXCL12 and SDF-1 at the site of fracture, MSCs interact with diverse immune cells, including T cells, B cells, macrophages, and neutrophils.74 The mechanisms underlying these interactions can be categorized into two main types. Firstly, MSCs can establish direct contact with immune cells, as observed in the interaction between MSCs and T cells. Through the binding of PD-L1 and the ligand PD-1/2, MSCs effectively inhibit T cell proliferation.75 Secondly, MSCs can modulate cytokine secretion in response to the immune microenvironment. For example, they can induce M2-like polarization of macrophages by regulating the secretion of TGF-β.76 When stimulated by pro-inflammatory factors like IFN-γ, TNF-α, IL-1, and IL-17, MSCs exert their anti-inflammatory function through the secretion of specific molecules. Co-culture studies involving MSCs and macrophages have demonstrated that MSCs enhance the expression of PGE2 through the iNOS and COX-2 pathways, subsequently inhibiting the production of inflammatory factors by binding to corresponding receptors on macrophages.77 In summary, through various mechanisms, MSCs and diverse immune cells collaborate to maintain the immune balance during fracture healing, thus facilitating the orderly progression of the healing process.
3 Crosstalk between immune homeostasis and biomaterials in bone regeneration
3.1 Advantages of hydrogels in regulating immune homeostasis
When immune homeostasis is disrupted, the immune system becomes compromised, leading to prevalent pro-inflammatory conditions and potential damage or inflammation within the organism.78 In this regard, immunotherapy has emerged as a promising therapeutic strategy for immune dysregulation-related diseases in various fields such as COVID-19, cancer oncology, organ transplantation, and trauma repair.79,80 To effectively deliver immunotherapeutic drugs, cells, or organs, suitable carriers are required. Hydrogels have garnered significant interest as carriers due to their advantageous properties. These materials consist of a three-dimensional cross-linked network and offer biocompatibility, non-immunogenicity, and non-toxicity.81 Compared with other drug carriers, hydrogels possess several key advantages, including low invasiveness and injectability, controlled release of encapsulated substances, biodegradability with non-toxic degradation products, and responsiveness to external stimuli. Moreover, hydrogels provide a stable immune microenvironment and reliable mechanical support for transplanted cells (Fig. 4). Ongoing research in the field of hydrogels and immunotherapy indicates that the development of highly controlled hydrogels holds great clinical promise for the future.
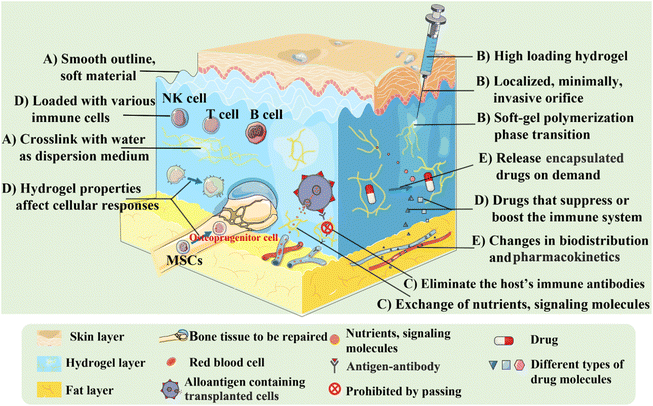 |
| Fig. 4 The advantageous features of hydrogels in the regulation of immune homeostasis. (A) Hydrogels are polymer networks crosslinked with water as the dispersion medium, exhibiting a smooth contour and soft material properties that contribute to excellent biocompatibility. (B) Through minimally invasive surgical procedures, drug-loaded hydrogels can be introduced into the body and subsequently transformed into hydrogels using methods such as polymerization phase transition. (C) Hydrogels serve as semi-permeable barriers, enabling the exchange of nutrients and signaling molecules between host and donor cells while selectively preventing the passage of antibodies. (D) Immunotherapy commonly utilizes hydrogels as carriers for immune cells and immunomodulatory drugs. (E) Drug carriers often influence pharmacokinetics and biological distribution, and hydrogels offer the additional benefits of on-demand release and deep tissue penetration. | |
For implant materials that exhibit poor biocompatibility, immune recognition initiates a series of cellular processes, including sustained inflammation, foreign-body giant cell formation, fibrosis, and tissue damage in the surrounding area. These processes can have detrimental effects on the functionality of the implant material and lead to significant pain and discomfort for the patient. Commonly used implant materials encompass hydrogels, ceramics, metals, and plastics. Hydrogels, specifically, are cross-linked polymers with water as the dispersion medium, closely resembling human tissue and thereby exhibiting remarkable biocompatibility.82 The soft and elastic nature of hydrogels ensures a smooth and complete contour, reducing the inflammatory response and fibrosis of surrounding cells, while promoting cell viability, forming the foundation of the hydrogel's immune functionality. While hydrogels are generally considered non-toxic and non-immunogenic, caution is advised based on clinical studies that suggest the potential for delayed moderate-to-severe immune-mediated adverse effects associated with implanted hydrogels, necessitating further research for validation.83
Non-surgical routes of drug administration, including nasal, vaginal, rectal, and oral delivery, often present challenges due to foreign body reactions and mucosal barriers, resulting in suboptimal outcomes, drug degradation, and severe inflammatory responses.84,85 In contrast, hydrogels offer advantages in terms of localized administration, minimal invasiveness, and high drug-loading capacity, achieved through smaller surgical wounds and syringe-based delivery. Injectable hydrogels, formed through rapid sol–gel phase transition or in situ chemical polymerization, enable the loading of diverse immunotherapeutic drugs, allowing direct injection into the target site for the desired effects, such as targeted modulation of the tumor microenvironment. However, conventional systemic administration typically necessitates high dosages or multiple injections, which can lead to low patient compliance and severe immune-related adverse reactions in individuals with tumors.86
Encapsulated cell therapy involves the transplantation of non-autologous cells, tissues, or organs in an immune-isolated manner, enabling the restoration of lost bodily functions in immune-incompatible recipients without the need for long-term immunosuppressive treatment. Hydrogels can be tailored to mimic tissue properties, facilitating the incorporation of various immune cells and drugs while selectively excluding significant immune antibodies of the host through pore size adjustment. By providing a semipermeable physiological barrier, hydrogels enable the exchange of nutrients and signaling molecules between host and donor cells, while offering mechanical support and protection against immune rejection.87 This approach has shown promise in pancreatic transplantation and encapsulated cell therapy for nerve cells, where encapsulating the transplanted cells within semipermeable hydrogels effectively provides localized nutrition and sustains physiological functions of the pancreas or nerves, all while circumventing the challenges of immune rejection.88
Payloads commonly utilized in immunotherapy encompass immune cells and immunomodulatory drugs, such as immune checkpoint inhibitors, vaccines, immune adjuvants, adoptive cell transfer, and nonspecific immune assessment factors. These payloads play a pivotal role in intelligent drug delivery systems and immune homeostasis regulation (Fig. 5).89,90 The response of immune cells holds significant influence over the success or failure of biomaterials following implantation. Consequently, investigating hydrogels and macrophage encapsulation systems in bone regeneration can offer a means to modulate multiple macrophage functions and enhance the performance of bone tissue engineering.91 Diverse immunomodulatory drugs can either suppress or enhance the immune response to hydrogels. For instance, bile acids possess anti-inflammatory and immunosuppressive properties, contributing to blood pressure reduction and hypertension relief. In cancer immunotherapy, loading immunotherapy drugs can amplify the patient's immune response, leading to tumor cell elimination, inhibition of tumor metastasis and recurrence. Additionally, the payload can indirectly impact the patient's immune homeostasis by modifying the properties of the hydrogel to achieve therapeutic effects. By altering the chirality of the peptide cross-linker to impede enzymatic degradation of the scaffold, the D-amino acid cross-linked microporous annealed particle (MAP) hydrogel demonstrated the ability to activate specific immune responses, enhance the regenerative capacity of biomaterials, and induce skin regeneration.92
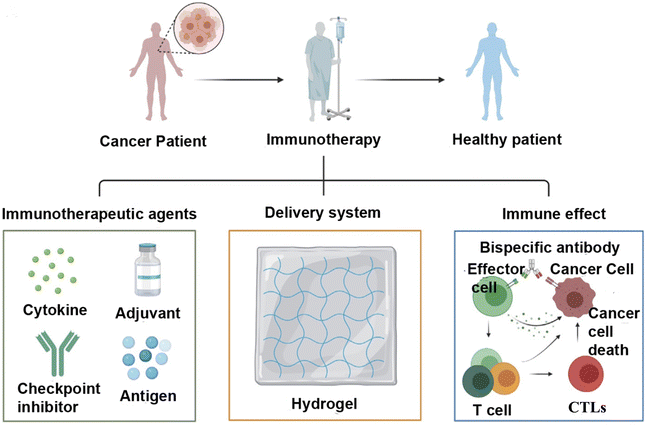 |
| Fig. 5 Hydrogels are applied in the delivery of immunomodulatory molecules, which include cytokines, adjuvants, checkpoint inhibitors, and antigens. Adapted with permission.89 Copyright 2021, Frontiers. | |
Drug carriers, including polymers, lipids, and inorganic materials, possess the ability to modify the pharmacokinetics and biodistribution of small-molecule drugs, leading to enhanced drug bioavailability and prolonged duration of action.93 In addition to these crucial functions, hydrogels exhibit desirable features such as on-demand drug release and deep tissue penetration, which contribute to reducing inflammation and mitigating adverse immune responses, rendering them ideal for drug delivery.94 A clinical study involving 10
008 patients with head injuries demonstrated that the administration of steroids increased mortality rates due to the systemic immunosuppressive effects throughout the body (non-brain), emphasizing the importance of local delivery limited to the injury site.95 However, the cross-linking of the polymer network in hydrogels allows for tunability of their mechanical properties, providing the ability to protect therapeutic drugs and control their release.96 Intelligent hydrogels responsive to endogenous or exogenous stimuli enable sustained and controlled release of immunotherapeutic drugs, minimizing unwanted side effects on normal tissues.97 Thermal therapy facilitated by inorganic materials integrated with hydrogels can enhance cell membrane permeability through precise temperature control at the treatment site, promoting increased cellular uptake of drugs and controlled drug release within the hydrogel matrix. This approach helps to preserve the integrity of the human immune system, mitigating the risk of ablating normal cells and improving chemotherapy outcomes. Encapsulating antibiotics within hydrogels enables a selective and sustained delivery to phagocytes, enhancing cellular penetration and facilitating the treatment of intracellular infections. With their minimally invasive nature, hydrogels can be molded into desired shapes to accommodate irregular lesions and target deep tissue sites, such as those involved in cancer, wound healing, and bone repair, effectively minimizing side effects and adverse immune responses.
3.2 Preparation and characteristics of hydrogels used for local delivery
Hydrogels can be strategically tailored to meet the localized drug delivery requirements for diverse therapeutic agents. A straightforward approach to achieve optimal drug loading within hydrogels involves the integration of drug delivery nanoparticles.98,99 For instance, Kim et al. developed an adhesive hydrogel patch for transdermal drug delivery, where extra-large mesoporous silica nanoparticles (XL-MSNs) were employed as drug carriers.100 Notably, the XL-MSNs played a crucial role in promoting strong adhesion between the hydrogel patch and the skin tissue. Furthermore, the multifunctionalization of drug-loaded nanoparticles within the hydrogel system has emerged as an intriguing avenue of research. For instance, a previous study designed MnO2-coated calcium phosphate microspheres (MMS) with the ability to serve as carriers for fibroblast activating protein inhibitor (FAPi), scavengers of reactive oxygen species (ROS), and generators of oxygen. These FAPi-loaded MMS were effectively immobilized within a methacrylated PGA/methacrylated gelatin (m-PGA/GelMA) hydrogel through hydrogen bonding. Animal experiments convincingly demonstrated the remarkable capability of this multifunctional composite hydrogel to regulate the tissue microenvironment and achieve desirable therapeutic outcomes upon in situ implantation into osteoporotic bone defects.101
Another common way to increase drug delivery efficiency is introducing special microstructures into hydrogels.102,103 The inherent hydrophilicity of hydrogels places limitations on the loading of hydrophobic drugs. To overcome this challenge, researchers have introduced hydrophobic microstructures within the hydrophilic hydrogel network.104,105 An exemplary approach is the development of a supramolecular hydrogel system known as a host–guest macromer (HGM) hydrogel by Bian et al. The crosslinking of this hydrogel is established through the host–guest interaction between cyclodextrin (CD) and the aromatic groups of the gelatin polymer chain.106,107 The molar ratio of cyclodextrin to aromatic groups in the HGM hydrogel exceeds 1, enabling the utilization of spare hydrophobic cavities within cyclodextrin as carriers for small hydrophobic drugs such as dexamethasone and icariin. Another effective strategy involves the use of amphiphilic polymers to construct hydrophobic drug-loaded hydrogels. Tong et al. employed atom transfer radical polymerization (ATRP) to polymerize a poly(ethylene oxide)-block-poly(2-(diisopropylamino) ethyl methacrylate) (PEO-b-PDPA) copolymer. Initially, the amphiphilic PEO-b-PDPA polymers self-assemble into micelle cores to encapsulate hydrophobic drugs. These micelle cores are then mixed with hydrazide-modified carboxymethyl cellulose (CMC-NH2) and oxidized carboxymethyl cellulose (CMC-CHO) to form an injectable hydrogel through a Schiff-base reaction, enabling controlled drug release.108 Firstly, amphiphilic PEO-b-PDPA polymers were self-assembled to micelle cores to encapsulate hydrophobic drugs, and then these micelle cores were mixed with hydrazide-modified carboxymethyl cellulose (CMC-NH2) and oxidized carboxymethyl cellulose (CMC-CHO) to form an injectable hydrogel by a Schiff-base reaction, with controllable drug release. Additionally, electrostatic attraction can be employed for local drug delivery in hydrogels, particularly for electrically charged drugs.109 Jia et al. developed a heparin-decorated hyaluronic acid-based hydrogel particle for the delivery of bone morphogenetic protein 2 (BMP-2).110 The retention ability of the hydrogel particle for BMP-2 was strongly influenced by the concentration of heparin, as the abundant negatively charged sulfated groups of heparin polymers exerted electrostatic attraction, facilitating long-term association with BMP-2.
In contrast, drugs, particularly peptide drugs, can be directly grafted onto the backbone of hydrogel networks.111 Zhang et al. employed methacrylated icariin (Ica-MA), methacrylated hyaluronic acid (HA-MA), and type I collagen (Col I) to fabricate an icariin-conjugated hyaluronic acid/collagen hydrogel for reconstructing the osteochondral interface.112 During the gelation process, icariin was incorporated into the network of the hydrogel through radical polymerization involving the methacrylate groups of Ica-MA and HA-MA. The grafted icariin was found to promote both chondrogenesis and osteogenesis, facilitating the repair of the osteochondral interface. Similarly, Yu et al. grafted a novel calcitonin gene-related peptide (CGRP) onto a photocrosslinking hyaluronic acid-based hydrogel via dynamic covalent hydrazone bonds.113 This enabled the hydrogel to enhance the proliferation and osteogenesis of BMSCs through sustained release of bioactive CGRP peptides. Moreover, besides their therapeutic effects, these grafted drugs also played a crucial role in the crosslinking of the hydrogel. Cai et al. reported an amphoteric copolymer-based hydrogel for bone regeneration.114 The hydrogel was synthesized via one-step radical polymerization involving (N-acyl glycinamide) (NAGA), anionic acrylate alendronate (AcAln), and cationic 2-(acryloyloxy)-N,N,N-trimethylethan-1-aminium (DMAEA-Q). In addition to promoting BMSCs osteogenesis, AcAln contributed abundant bisphosphate groups that formed hydrogen bonds between dual bisphosphate groups, and ionic pairs between bisphosphate groups and the quaternary ammonium groups of DMAEA-Q. These interactions served as the main crosslinking mechanisms, ensuring the mechanical flexibility of the hydrogel.
In addition to enhancing drug loading efficiency, researchers also emphasize the design of controllable drug release strategies.115 Responsive drug release from hydrogels can be achieved through two primary modes. The first mode involves utilizing external stimuli, such as light, heat, and magnetism, to trigger drug release from hydrogels.116 Among these external stimuli, light is the commonest used one because of its easy accessibility and convenient operability.117 Ossipov et al. developed a photosensitive prodrug-grafted hyaluronic acid hydrogel using orthogonal chemistry.117 By employing a photolabile ortho-nitrobenzyl linker, dopamine was grafted as a model drug to the hydrogel backbone. Under UV light exposure, the photosensitive linker rapidly broke, enabling controlled release of the model drug. The second mode is based on the bio-responsiveness of hydrogels to internal physiological signals such as pH, redox potential, enzymes, and glucose.118,119 Wu et al. fabricated a dual-sensitive hydrogel composed of chitosan, hyaluronic acid, and β-sodium glycerophosphate.120 Gelation of this system was triggered by body temperature sensitivity conferred by β-sodium glycerophosphate, while the acid-responsive drug release was controlled by the protonation of chitosan. Glucose-sensitive hydrogels, on the other hand, are often employed to achieve responsive insulin release for diabetes patients. Gu et al. utilized a core–shell microneedle hydrogel for transdermal self-regulated insulin delivery.121 As the glucose concentration increased, the encapsulated glucose oxidase catalyzed its conversion into H2O2. Subsequently, H2O2 triggered the cleavage of the linker N1-(4-boronobenzyl)-N3-(4-boronophenyl)-N1,N1,N3,N3-tetramethylpropane-1,3-diaminium (TSPBA) between the insulin and poly(vinyl alcohol) (PVA), resulting in the release of insulin into the bloodstream as required.
4 Local delivery hydrogels to restore immune homeostasis
4.1 Delivery of small molecules
The use of hydrogels for the delivery of interleukin-4 (IL-4), a cytokine known for its anti-inflammatory properties, has been extensively studied to promote bone regeneration.122 Researchers have reported that local delivery of IL-4 using calcium-enriched gellan gum hydrogel facilitated bone defect regeneration in rats by modulating macrophage polarization, promoting osteogenic differentiation of bone MSCs, and reducing cell apoptosis.123 Another study by Zou et al. involved loading IL-4 and bone morphogenetic protein-2 (BMP-2) onto graphene oxide (GO) and embedding them into a carboxymethyl chitosan (CMC)/poly(ethylene glycol) diacrylate (PEG-DA) hydrogel. This hybrid hydrogel controlled the release of IL-4, thereby transforming M1 macrophages into M2 macrophages.124 In a separate investigation, Krieger et al. demonstrated that delivery of stromal-derived factor-1α (SDF-1α) using PEG-DA hydrogel stimulated chemotaxis of anti-inflammatory monocytes and supported the growth of microvascular networks.125 Lipid mediators, known to regulate inflammation and bone regeneration, have also been utilized.126 It has been demonstrated that collagen/Pluronic F127 hydrogel loaded with resolvin D1 (RvD1), derived from omega-3 docosahexaenoic acid, effectively reduced inflammation and promoted calvarial defect repair in rats.127 Hydrogels have also been employed as delivery carriers for sustained release of anti-inflammatory drugs in bone tissue regeneration. For instance, Chauhan et al. loaded dexamethasone (Dex) into an oxidized pullulan (OP)/PEG hydrazine hydrogel, providing pH-sensitive sustained release and improved chronic inflammatory conditions.128 Moreover, several traditional Chinese medicines have shown promise in immune response regulation and bone healing. Guo et al. demonstrated that delivery of baicalin with a GO-demineralized bone matrix (DBM) hybrid scaffold induced macrophage differentiation into the M2 type, promoting angiogenesis, osteogenesis, and calvarial bone regeneration in vivo.129 Puerarin has been reported to enhance bone formation through inhibition of osteoclast activities and stimulation of osteoblast differentiation.130,131 Recent evidence suggests that delivery of puerarin via GelMA hydrogel reconstructed the pelvic floor, associated with reduced expression of inflammatory cytokines (interleukin-3 (IL-3) and interleukin-6 (IL-6)) and matrix metalloproteinase-2/9 (MMP-2/9), thereby minimizing multiple inflammations.132 However, further research is needed to directly investigate the immunomodulatory effects of puerarin delivery during bone regeneration.
4.2 Delivery of biologics
Biologics, which are produced using biological engineering technology and mimic the structures of antibodies, receptors, and cytokines, have gained significant attention for their ability to modulate signal transduction pathways.133 In the field of skeletal diseases, the utilization of biologics has increased due to their high specificity towards molecular targets.134 In the context of bone regeneration, the negative impact of tumor necrosis factor (TNF)-α overexpression led Timmen et al. to investigate the use of an anti-TNF antibody (infliximab) for fracture treatment, revealing significant improvements in fracture healing upon TNF-α blockade.135 Similarly, Dixit et al. examined the effects of an anti-IL-17 antibody on bone tissue regeneration, observing enhanced expression of osteogenic markers and reduced oxidative stress at the defect site.136 Although these studies demonstrated the potential of biologics to promote bone regeneration, their administration without suitable carriers may not ensure sustained effects. To address this, hydrogels have emerged as effective delivery carriers for enhancing the therapeutic outcomes of biologics. Chen et al. implanted an injectable, self-healing, adhesive HA@SDF-1α/M2D-Exos hydrogel, which synchronously and sustainably releases stromal cell-derived factor-1α (SDF-1α) and M2 macrophage-derived exosomes (M2D-Exos), thereby promoting osteogenesis and angiogenesis, offering a promising modality for accelerating bone repair by addressing the challenges of nonunion fracture (Fig. 6).137 In addition to physical entrapment, chemical conjugation serves as another effective strategy for drug encapsulation and release in hydrogel-based delivery systems for biologics. Notably, recombinant protein cross-linked hydrogels have been explored for bone regeneration. For instance, the incorporation of recombinant collagen peptide microspheres into alginate hydrogels enabled in situ gelation and delivery of BMP-2, inducing bone formation.138 However, the application of hydrogels for delivering recombinant proteins with immunomodulatory activity in bone repair remains limited, warranting further research in this area.
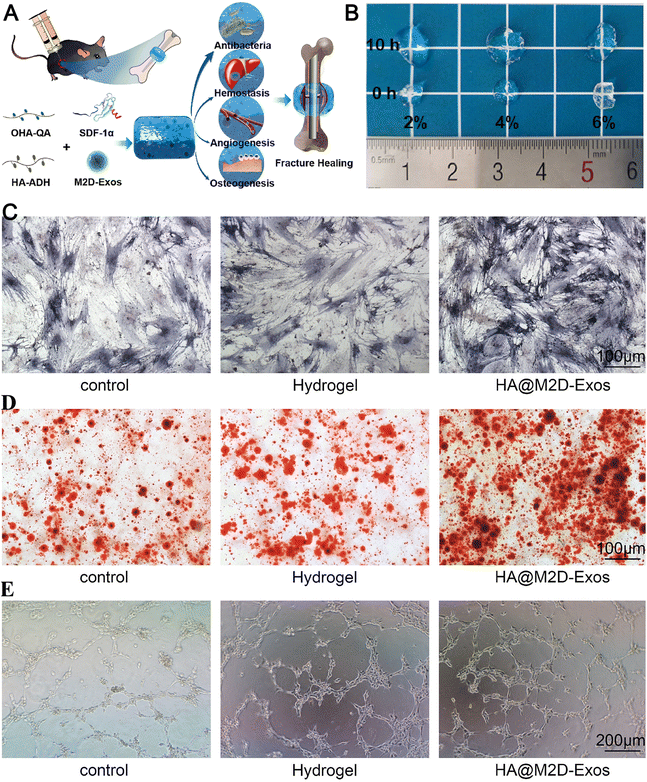 |
| Fig. 6 The immunomodulatory effects of biologics loaded in hydrogels for bone regeneration. (A) Schematic image illustrating the effect of HA@SDF-1α/M2D-Exos hydrogels on fracture repair. (B) Representative image of the swelling equilibrium of an HA-based hydrogel. (C and D) ALP staining and Alizarin Red staining of treated HMSCs after osteogenic induction following different treatments (scale bar = 100 μm). (E) Tube formation assay of HUVECs in different groups. Adapted with permission.137 Copyright 2023, Elsevier B.V. | |
The delivery of biologics using hydrogels shows significant promise due to the highly compatible nature of hydrogels with nucleic acids and proteins, which are susceptible to environmental conditions. However, achieving effective and controlled release of biologics remains a challenge. A key area of research involves the development of stimuli-responsive hydrogels capable of responding to external cues such as pH, reactive oxygen species (ROS), heat, and light, enabling targeted delivery of biologics in a spatially and temporally controlled manner.139 While there are still challenges to address, the investigation of hydrogel systems for delivering biologics with immunoregulatory functions holds great potential for modulating local immune responses and promoting enhanced bone regeneration.
4.3 Delivery of small EVs
EVs are small membrane-encapsulated particles released by cells that serve as intercellular messengers, facilitating the transfer of proteins and genetic information. Their excellent biosafety and stability have recently garnered significant attention in the fields of bone regeneration and immune modulation.140 However, achieving a long-lasting retention and controlled release at defect sites using appropriate carriers remains a challenge. The utilization of hydrogels as delivery vehicles for EVs offers a novel approach to modulating the local immune response and enhancing bone regeneration.
Various sources of EVs have been explored for the treatment of bone defects, with a particular focus on the immunomodulatory properties of mesenchymal stem cell (MSC)-derived EVs.141 Li et al. investigated the therapeutic potential of adipose-derived MSC EVs (ADSC-EVs) loaded with gelatin nanoparticles for traumatic bone defects. They demonstrated that ADSC-EV delivery inhibited M1 macrophage polarization while promoting M2 macrophage polarization, resulting in enhanced bone healing.142 Similarly, Guan et al. utilized EVs derived from BMSCs loaded in extracellular matrix (ECM)-mimic hydrogels to facilitate growth plate injury repair in rats. The EVs inhibited inflammation and stimulated ECM synthesis, leading to successful repair of the injury (Fig. 7A).143 In another study, Shen et al. incorporated EVs derived from dental pulp stem cells (DPSCs) into chitosan hydrogels. The presence of miR-1246 in DPSC exosomes effectively induced M2 macrophage polarization, reduced inflammatory cytokines, and accelerated alveolar bone healing and periodontal epithelium regeneration in mice (Fig. 7B).144 Additionally, EVs derived from macrophages have shown promise in modulating the immune microenvironment and bone regeneration. It has been observed that EVs from M0 and M2 macrophages promote bone repair, while EVs from M1 macrophages inhibit bone regeneration.35 It has been demonstrated that M0 and M2 macrophage-derived EVs promote bone repair and that M1 macrophage-derived EVs inhibit bone regeneration.145 Therefore, delivering EVs derived from M0 and M2 macrophages could serve as a potential strategy to regulate the immune microenvironment and enhance bone regeneration.
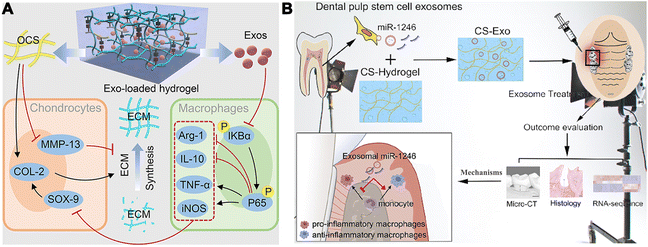 |
| Fig. 7 The immunomodulatory effects of EVs loaded into hydrogels for bone regeneration. (A) The diagram depicts the utilization of hydrogel loaded with exosomes derived from BMSCs to facilitate repair of growth plate injuries by suppressing inflammation. Adapted with permission.143 Copyright 2022, Elsevier. (B) The miR-1246 present in DPSC exosomes stimulates the polarization of M2 macrophages.144 Copyright 2020, Elsevier B.V. | |
4.4 Strategies for cell-based immunomodulation
MSC-based therapy has emerged as a promising strategy for bone regeneration due to the inherent ability of MSCs to maintain tissue homeostasis.146 The regenerative effects of MSCs in bone tissue engineering can be achieved through both endogenous MSC recruitment and exogenous MSC transplantation.147 Recent evidence indicates that MSCs exert immunomodulatory actions, in addition to their role in differentiation and repopulation, further enhancing bone regeneration.148 Apart from BMSCs, MSCs derived from adipose tissue (ADSCs), dental pulp (DPSCs), and umbilical cord Wharton's jelly (WJMSCs) have also been explored for the treatment of bone defects. MSCs possess low immunogenicity and can dampen immune responses. Their immunomodulatory actions involve interactions with various immune cells, including macrophages, lymphocytes, and natural killer (NK) cells, facilitated by the secretion of cytokines such as 2,3-dioxygenase (IDO), IL-6, interleukin-10 (IL-10), and nitric oxide synthase (iNOS) within the inflamed tissue microenvironment.149,150 Hydrogels, with their unique physiochemical properties and three-dimensional structure, offer inherent advantages in mimicking the natural extracellular matrix (ECM) and supporting MSC adhesion and survival on a large scale.151 Consequently, the delivery of MSCs using hydrogel culture systems represents an effective approach for modulating the immune response and enhancing bone regeneration.
For instance, Seebach et al. seeded BMSCs on fibrin hydrogels and implanted these composite constructs into femoral bone defects. Compared with cell-free fibrin hydrogels, the BMSC-seeded fibrin implants exhibited enhanced macrophage and endothelial progenitor cell migration into the hydrogel, leading to accelerated tissue maturation and blood vessel formation during the early stages of healing.152 Ji et al. developed a hybrid scaffold by incorporating BMSC-encapsulated thermosensitive hydroxypropyl chitin hydrogel (HPCH) into a 3D-printed poly(ε-caprolactone) (PCL)/nanohydroxyapatite (nHA) scaffold for the treatment of calvarial defects in rats. This hybrid scaffold effectively promoted BMSC osteogenic differentiation and polarization of macrophages towards the M2 phenotype, thereby facilitating osteoinduction of BMSCs and angiogenesis (Fig. 8).153 To enhance the immunomodulatory effects of MSCs, Ueno et al. engineered lentivirus-transduced BMSCs overexpressing IL-4 and delivered these IL-4-modified BMSCs within a gelatin-based microribbon hydrogel to critical-size long bone defects in mice. The IL-4 BMSCs continuously released IL-4 from the microribbon hydrogel, attracting macrophages to the hydrogel and polarizing them towards the M2 phenotype, while not inhibiting M1 macrophage polarization. This localized immune modulation resulted in enhanced bone formation and regeneration after a 6-week period.154
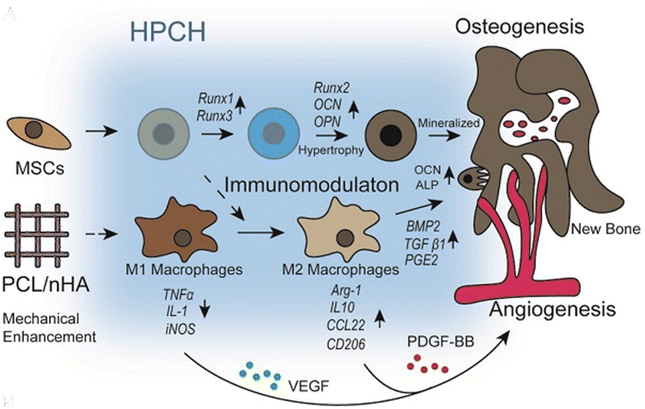 |
| Fig. 8 The diagram depicts the BMSC-encapsulated HPCH/PCL/nHA scaffold designed for bone regeneration. Adapted with permission.153 Copyright 2020, Ivyspring. | |
The effectiveness of MSC-based bone regeneration is not always assured due to the loss of cellular properties, including immunomodulatory activity, during in vitro expansion and the unpredictable behavior of MSCs in diseased microenvironments. To enhance MSC-based bone regeneration, the development of biocompatible hydrogels capable of preserving MSC properties is a promising strategy. Furthermore, it has been discovered that the stiffness of hydrogels can influence the immunomodulatory efficacy of MSCs. MSCs cultured on soft matrices demonstrate superior anti-inflammatory performance compared with those on stiff matrices.155 Therefore, the mechanical response of MSCs to hydrogels must be considered when designing MSC-embedded hydrogels for immune regulation. Additionally, MSCs are a heterogeneous population, and not all MSCs possess the same immunomodulatory abilities.156 Thus, the selection of appropriate cell types is crucial for the success of MSC-based bone regeneration. Recent evidence indicates that Wharton's jelly-derived MSCs (WJMSCs) exhibit enhanced immunomodulatory activity compared with BMSCs, highlighting the potential of delivering WJMSCs with hydrogels to promote bone regeneration.157
5 Combination of immunomodulatory regeneration with other techniques
Combination therapy presents a potential avenue to enhance treatment efficacy and reduce the duration of treatment. In the context of bone regeneration, hydrogel-based immunotherapy offers the potential to improve therapeutic outcomes by modulating macrophage functions to regulate immune responses and prevent bone resorption. However, the integration of immunotherapies with other techniques within novel multifunctional hydrogels holds even greater promise for achieving efficient bone repair through synergistic effects. One of the major challenges in bone tissue engineering is the occurrence of bone infections, which contribute to high morbidity rates and significant healthcare burdens.158 Bacterial infections disrupt the balance of bone-forming osteoblasts and bone-resorbing osteoclasts due to the accumulation of inflammatory mediators, greatly impacting the process of bone regeneration.159 The combination of antibacterial and osteoimmunomodulatory activities represents an innovative strategy that can simultaneously promote osteogenesis and eliminate bacterial presence in defect areas. Ou et al. developed a hybrid hydrogel composed of gelatin methacrylate (GelMA), which closely mimics the natural extracellular matrix (ECM) environment. This hydrogel was loaded with nanosilver (nAg), an effective broad-spectrum antibacterial agent, and halloysite nanotubes (HNTs) to facilitate bone regeneration. The nAg/HNTs/GelMA hydrogel demonstrated notable osteoimmunomodulatory and antibacterial properties (Fig. 9). It exhibited significant inhibition zones against Escherichia coli (E. coli) and Staphylococcus aureus (S. aureus). Moreover, in infected bone defects, the nAg/HNTs/GelMA-treated group showed substantially fewer S. aureus colonies compared with other groups, along with inhibited inflammatory reactions in vivo. The incorporation of nAg in the hydrogel resulted in reduced expression of IL-1β and IL-6, while increasing IL-10 expression compared with other groups. This enhanced osteoimmunomodulatory stability under infectious conditions and mitigated the inflammatory responses. Furthermore, the nAg/HNTs/GelMA group exhibited greater osteogenesis as evaluated by micro-computed tomography (micro-CT), bone mineral density, and bone volume fraction (BV/TV) at the two-month mark. Taking these together, the reported platform demonstrates synergistic antibacterial and osteoimmunomodulatory effects for bone regeneration, particularly in the reconstruction of infected bone defects.160 Another study explored the antibacterial, immunomodulatory, and osteogenic properties of a composite hydrogel consisting of polylactic-glycolic acid (PLGA) microspheres embedded within a porcine small intestinal submucosa (SIS) hydrogel. This platform was designed with sequential drug release capabilities, incorporating LL-37 and W9 peptides. The presence of LL-37 peptide provided multifunctionality, including antibacterial activity, cell recruitment, and immunoregulation, while the W9 peptide promoted calcium deposition and upregulated osteogenic gene expression. Consequently, this prepared platform exhibited great potential for accelerated bone regeneration through immune regulation and antibacterial effects.161
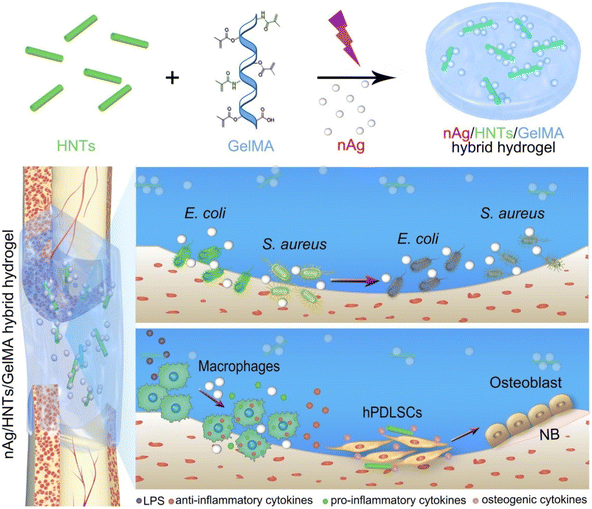 |
| Fig. 9 Schematic illustration of the nAg/HNTs/GelMA hydrogel, including its fabrication procedure, osteoimmunomodulatory properties, and antibacterial activity (LPS: lipopolysaccharide; hPDLSCs: human periodontal ligament stem cells; NB: new bone). Adapted with permission.160 Copyright 2020, Elsevier B.V. | |
In addition to bone infection, the formation of orthopedic biofilms and weak post-surgical osseointegration pose significant challenges in orthopedics, potentially leading to implant failure.162 Addressing both biofilm infections and osseointegration simultaneously has therefore become crucial. To this purpose, Li et al. developed an adhering hydrogel consisting of poly(vinyl alcohol) (PVA) modified with chitosan (Cs) and polydopamine (PDA), referred to as PCP, along with the nitric oxide (NO) donor S-nitrosuccinic acid (RSNO). This hydrogel was then coated on a red phosphorous (RP) nanofilm deposited on a titanium implant (Ti-RP/PCP/RSNO), synergistically combining photothermal therapy (PTT) and immunotherapy to eliminate infection and enhance osteogenesis.163 PTT is a novel local therapy method that utilizes light-to-heat conversion, resulting in the non-invasive and low-toxicity destruction of bacterial cells.164,165 The fabricated hydrogel exhibited significant heat generation (approximately 53.1 °C) under near-infrared (NIR) irradiation, owing to the photothermal activities of RP and PDA. Subsequently, the Ti-RP/PCP/RSNO-treated group achieved over 93.1% removal of an MRSA biofilm mass after NIR irradiation, followed by a 2-day biofilm incubation period. Furthermore, the released NO at a low concentration exhibited antibacterial effects, induced M1 polarization of macrophages, upregulated the expression of osteopontin (Opn) and osteocalcin (Ocn) genes involved in osteoblast differentiation, as well as TNF-α expression, thereby enhancing osteogenic capability. Consequently, the prepared hydrogel was proposed as a novel biomaterial for simultaneous biofilm elimination and promotion of osteogenesis through phototherapy and immunotherapy.163 Additionally, PTT's photothermal effect can generate acoustic waves, facilitating photoacoustic imaging for visualizing targeted sites in bone defects.166 Regarding this property, Jiang et al. developed an injectable hydrogel based on an alginate/sericin/graphene (Alg/Ser/GO) framework with HRP/H2O2 enzymatic cross-linking. Silk sericin promoted macrophage infiltration, M2 polarization, and the transition from the M1 to M2 phenotype, which is associated with anti-inflammatory macrophages that aid angiogenesis and bone regeneration. GO, the oxidized form of graphene, acted as a photothermal agent while also improving osteogenic differentiation and bone regeneration. Monitoring the changes in temperature under NIR irradiation confirmed the higher temperature of the injected hydrogel. The mild heat generated by the photothermal effect under NIR irradiation enhanced the differentiation of BMSCs and facilitated bio-imaging for tracking the location of the injected hydrogel. Moreover, GO exhibited great potential for photoacoustic-based imaging to monitor the hydrogel. Ultimately, the fabricated injectable hydrogel demonstrated a synergistic effect on bone healing after 8 weeks, offering traceability and biocompatibility. By combining imaging modalities with therapeutic agents, enhanced strategies for bone regeneration treatment are realized.116
6 The limitations of current hydrogel delivery strategies and challenges of clinical translational application
6.1 The less-than-desirable mechanical strength of hydrogels
Despite their advantages as a delivery system, hydrogels still face certain limitations that require further attention. One major concern is the inadequate mechanical strength of natural polymers such as chitosan, fibrin, and collagen, despite their excellent biocompatibility. Alginate hydrogels, for instance, are commonly used in bone tissue engineering due to their unique properties such as low toxicity, affordability, and widespread availability. However, meeting the mechanical strength requirements for bone tissue engineering, which typically range from 25 to 70 MPa for compressive strength and around 200 ± 36 MPa for cortical bone, poses a challenge.167 To address this issue, chemical crosslinking networks have been extensively explored to enhance the mechanical properties of hydrogels, resulting in improved strength and durability.168 Synthetic polymers, although they offer certain advantages by circumventing some limitations, such as unpredictable immune responses and poor degradation, still require solutions to overcome these challenges.169 Thus, finding the appropriate polymer composition and crosslinking methods to enhance the properties of hydrogels for bone tissue engineering remains a long-term goal.
6.2 The unstable release efficiency of hydrogels
Achieving a sustained and stable release of therapeutic agents from hydrogels is crucial for promoting the spontaneous bone regeneration process. However, the breakdown of hydrogels by biological factors can hinder this process. To address these limitations, one approach is to enhance the binding affinity of hydrogels through physical and chemical cross-linking methods. In physically cross-linked hydrogels, the release rate of agents is primarily influenced by factors such as viscosity, degradation, and weak interactions. On the other hand, chemical crosslinking involves the formation of covalent modifications between hydrogels and agents. However, it is important to consider that covalent binding might compromise the activity of the agents. To overcome this drawback, stimuli-responsive hydrogels that can undergo changes in response to the local environment offer a potential solution. These hydrogels can exhibit desired modifications without impairing the activity of the agents.170 Some studies have demonstrated the feasibility of independently and simultaneously releasing multiple bioactive proteins from hydrogels through independent affinity interactions.171 In conclusion, achieving precise control over agent release poses a significant challenge in the development of ideal hydrogels.
6.3 The unsetting influence of hydrogels on cells
The application of hydrogels loaded with stem cells for bone regeneration has been reported as promising. However, several challenges must be addressed. Firstly, hydrogels face difficulties in accurately replicating the complex microenvironment of the fracture site, leading to potential deviations in the growth of stem cells. Secondly, the structure of hydrogels may cause undesired differentiation of stem cells into specific cell lineages. Additionally, achieving a precise and targeted delivery of cargo to specific cells within the hydrogel is challenging, potentially impacting the behavior of other cells and introducing unpredictable side effects. Therefore, it is crucial to thoroughly assess the physical and chemical properties of hydrogels, including their morphology, stiffness, and biodegradability, to ensure their suitability for bone regeneration.172
6.4 The clinical research of hydrogels
In recent years, a wide range of hydrogels have been developed for both in vivo and in vitro studies, demonstrating promising results that warrant further investigation in clinical studies. Additionally, researchers have made advancements in modifying hydrogels to meet clinical demands. For instance, microfluidic technology enables the fabrication of micrometer-sized hydrogels with diverse geometries and morphologies, expanding their biomedical applications.173 Despite these advancements, challenges persist in large-scale manufacturing of hydrogels, particularly in maintaining their stability and repeatability at an industrial level. Sterilizing hydrogels presents another challenge due to their hydration properties. Comprehensive sterilization protocols are necessary to ensure that source materials and production processes are free from contamination. Terminal sterilization methods, both physical and chemical, can be employed as long as they do not compromise the performance and therapeutic efficacy of the hydrogels. Careful selection of the appropriate sterilization method and thorough testing of hydrogel activity are imperative.174,175 Another challenge in clinical research on hydrogels is storage. Hydrogels are typically stored in hydrated or dehydrated states based on their properties.176 However, bioactive components within hydrogels may undergo alteration when exposed to environmental temperatures or during long-term storage. Therefore, it is essential to assess the activity of the bioactive components before utilizing the hydrogels.
The utilization of hydrogels necessitates adherence to a series of tests outlined by the International Organization for Standardization (ISO), encompassing assessments of the material's chemical properties, cytotoxicity, and pre-clinical trials.142 These extensive pre-clinical trials and comprehensive clinical evaluations are essential, albeit time-consuming and costly, particularly when hydrogels are loaded with bioactive components.102 Consequently, the financial burden associated with the translational process of hydrogels from laboratory to clinical applications can be substantial.
7 Concluding remarks and future challenges
As researchers continue to advance in their understanding of hydrogels and foster increased collaboration, there has been a remarkable expansion in the application of hydrogels for various diseases. This progress, coupled with the rapid translation of hydrogels into human clinical trials, has ignited enthusiasm within the scientific community.177 However, there are several challenges that need to be addressed for the successful clinical translation of hydrogels and to shape their future perspectives. One major challenge is the limited understanding of the immune environment and the optimal design of hydrogels to achieve desired therapeutic outcomes. Although hydrogels hold promise as immunomodulatory platforms for tissue regeneration, further research is required to unravel the underlying mechanisms of immune response and enhance the effectiveness of hydrogel-based therapies. Drawing inspiration from the extensive research on tumor microenvironments, innovative technologies and perspectives can provide valuable insights for investigating the immune microenvironment during fracture repair. Key tools such as single-cell sequencing and genetic mouse lines will play a crucial role in advancing this field of research. Another crucial aspect to consider is the role of cell-to-cell communication in tissue repair. The viability of stem cells significantly impacts the activation of immune cells, highlighting the importance of designing hydrogels that facilitate effective cell communication. Additionally, the interplay between the immune microenvironment of bone and the overall systemic microenvironment remains poorly understood. It is essential to investigate microenvironmental changes during bone repair in diverse populations, including individuals with specific health conditions, such as osteoporosis, obesity, diabetes, or vitamin deficiencies.
While unraveling the immune microenvironment during bone repair presents significant challenges, it also opens up opportunities for the development of more effective and personalized hydrogels for immunotherapy. Future research should focus on addressing these challenges to advance the clinical translation of hydrogels and maximize their therapeutic potential. By gaining a deeper understanding of immune mechanisms and optimizing hydrogel design, researchers can pave the way for improved treatments and combining personalized approaches to tissue regeneration.
In summary, while the application of hydrogels in immunomodulatory regeneration of bone defects shows promise, addressing the challenges associated with their clinical translation is essential. Hopefully, by bridging the knowledge gaps and embracing innovative technologies, researchers can unlock the full potential of hydrogels in clinical settings, revolutionizing the field of regenerative medicine.
Conflicts of interest
The authors declare no competing interests.
Acknowledgements
This work was supported by the Wuhan Science and Technology Bureau (2022020801020464), Department of Science and Technology of Hubei Province (No. 2021CFB425, 2020BCB004), National Science Foundation of China (No. 82002313, 82072444, 31900963, 82202714), and China Postdoctoral Science Foundation (No. 2021TQ0118). M.-A. S. acknowledges the Incentive Fund from the University of Groningen. Fig. 1 was created using Figdraw (https://www.figdraw.com) and Fig. 4 was partly generated using Servier Medical Art, provided by Servier, licensed under a Creative Commons Attribution 3.0 unported license.
References
- B. G. Matthews, S. Novak, F. V. Sbrana, J. L. Funnell, Y. Cao, E. J. Buckels, D. Grcevic and I. Kalajzic, Heterogeneity of murine periosteum progenitors involved in fracture healing, eLife, 2021, 10, e58534 CrossRef CAS PubMed.
- Y. Zhang, J. Xu, Y. C. Ruan, M. K. Yu, M. O'Laughlin, H. Wise, D. Chen, L. Tian, D. Shi, J. Wang, S. Chen, J. Q. Feng, D. H. Chow, X. Xie, L. Zheng, L. Huang, S. Huang, K. Leung, N. Lu, L. Zhao, H. Li, D. Zhao, X. Guo, K. Chan, F. Witte, H. C. Chan, Y. Zheng and L. Qin, Implant-derived magnesium induces local neuronal production of CGRP to improve bone-fracture healing in rats, Nat. Med., 2016, 22(10), 1160–1169 CrossRef CAS PubMed.
- T. A. Einhorn and L. C. Gerstenfeld, Fracture healing: mechanisms and interventions, Nat. Rev. Rheumatol., 2015, 11(1), 45–54 CrossRef PubMed.
- N. Su, C. Villicana and F. Yang, Immunomodulatory strategies for bone regeneration: A review from the perspective of disease types, Biomaterials, 2022, 286, 121604 CrossRef CAS PubMed.
- S. Yue, H. He, B. Li and T. Hou, Hydrogel as a Biomaterial for Bone Tissue Engineering: A Review, Nanomaterials, 2020, 10(8), 1511 CrossRef CAS PubMed.
- A. Longoni, L. Knezevic, K. Schepers, H. Weinans, A. Rosenberg and D. Gawlitta, The impact of immune response on endochondral bone regeneration, npj Regener. Med., 2018, 3, 22 CrossRef CAS PubMed.
- P. Abdollahiyan, F. Oroojalian, A. Mokhtarzadeh and M. de la Guardia, Hydrogel-Based 3D Bioprinting for Bone and Cartilage Tissue Engineering, Biotechnol. J., 2020, 15(12), e2000095 CrossRef PubMed.
- Y. Jiang, N. Krishnan, J. Heo, R. H. Fang and L. Zhang, Nanoparticle-hydrogel superstructures for biomedical applications, J. Controlled Release, 2020, 324, 505–521 CrossRef CAS PubMed.
- D. Sivaraj, K. Chen, A. Chattopadhyay, D. Henn, W. Wu, C. Noishiki, N. J. Magbual, S. Mittal, A. M. Mermin-Bunnell, C. A. Bonham, A. A. Trotsyuk, J. A. Barrera, J. Padmanabhan, M. Januszyk and G. C. Gurtner, Hydrogel Scaffolds to Deliver Cell Therapies for Wound Healing, Front. Bioeng. Biotechnol., 2021, 9, 660145 CrossRef PubMed.
- P. Khayambashi, J. Iyer, S. Pillai, A. Upadhyay, Y. Zhang and S. D. Tran, Hydrogel Encapsulation of Mesenchymal Stem Cells and Their Derived Exosomes for Tissue Engineering, Int. J. Mol. Sci., 2021, 22(2), 684 CrossRef CAS PubMed.
- Y. Xiong, L. Chen, P. Liu, T. Yu, C. Lin, C. Yan, Y. Hu, W. Zhou, Y. Sun, A. C. Panayi, F. Cao, H. Xue, L. Hu, Z. Lin, X. Xie, X. Xiao, Q. Feng, B. Mi and G. Liu, All-in-One: Multifunctional Hydrogel Accelerates Oxidative Diabetic Wound Healing through Timed-Release of Exosome and Fibroblast Growth Factor, Small, 2022, 18(1), e2104229 CrossRef PubMed.
- Q. Hu, H. Li, E. Archibong, Q. Chen, H. Ruan, S. Ahn, E. Dukhovlinova, Y. Kang, D. Wen, G. Dotti and Z. Gu, Inhibition of post-surgery tumour recurrence via a hydrogel releasing CAR-T cells and anti-PDL1-conjugated platelets, Nat. Biomed. Eng., 2021, 5(9), 1038–1047 CrossRef CAS PubMed.
- D. Zhu, Z. Li, K. Huang, T. G. Caranasos, J. S. Rossi and K. Cheng, Minimally invasive delivery of therapeutic agents by hydrogel injection into the pericardial cavity for cardiac repair, Nat. Commun., 2021, 12(1), 1412 CrossRef CAS PubMed.
- D. Li, K. Chen, H. Tang, S. Hu, L. Xin, X. Jing, Q. He, S. Wang, J. Song, L. Mei, R. D. Cannon, P. Ji, H. Wang and T. Chen, A Logic-Based Diagnostic and Therapeutic Hydrogel with Multistimuli Responsiveness to Orchestrate Diabetic Bone Regeneration, Adv. Mater., 2022, 34(11), e2108430 CrossRef PubMed.
- X. Li, K. Xu, Y. He, B. Tao, K. Li, C. Lin, J. Hu, J. Wu, Y. Wu, S. Liu, L. Peng, H. Wang and K. Cai, ROS-responsive hydrogel coating modified titanium promotes vascularization and osteointegration of bone defects by orchestrating immunomodulation, Biomaterials, 2022, 287, 121683 CrossRef CAS PubMed.
- K. Okamoto, T. Nakashima, M. Shinohara, T. Negishi-Koga, N. Komatsu, A. Terashima, S. Sawa, T. Nitta and H. Takayanagi, Osteoimmunology: The Conceptual Framework Unifying the Immune and Skeletal Systems, Physiol. Rev., 2017, 97(4), 1295–1349 CrossRef CAS PubMed.
- J. R. Arron and Y. Choi, Bone versus immune system, Nature, 2000, 408(6812), 535–536 CrossRef CAS PubMed.
- M. Tsukasaki and H. Takayanagi, Osteoimmunology: evolving concepts in bone-immune interactions in health and disease, Nat. Rev. Immunol., 2019, 19(10), 626–642 CrossRef CAS PubMed.
- J. G. Rurik, H. Aghajanian and J. A. Epstein, Immune Cells and Immunotherapy for Cardiac Injury and Repair, Circ. Res., 2021, 128(11), 1766–1779 CrossRef CAS PubMed.
- M. N. Michalski and L. K. McCauley, Macrophages and skeletal health, Pharmacol. Ther., 2017, 174, 43–54 CrossRef CAS PubMed.
- Y. Shen, Y. Zhang, Z. Zhou, J. Wang, D. Han, J. Sun, G. Chen, Q. Tang, W. Sun and L. Chen, Dysfunction of macrophages leads to diabetic bone regeneration deficiency, Front. Immunol., 2022, 13, 990457 CrossRef CAS PubMed.
- L. J. Raggatt, M. E. Wullschleger, K. A. Alexander, A. C. Wu, S. M. Millard, S. Kaur, M. L. Maugham, L. S. Gregory, R. Steck and A. R. Pettit, Fracture healing via periosteal callus formation requires macrophages for both initiation and progression of early endochondral ossification, Am. J. Pathol., 2014, 184(12), 3192–3204 CrossRef CAS PubMed.
- L. Vi, G. S. Baht, E. J. Soderblom, H. Whetstone, Q. Wei, B. Furman, V. Puviindran, P. Nadesan, M. Foster, R. Poon, J. P. White, Y. Yahara, A. Ng, T. Barrientos, M. Grynpas, M. A. Mosely and B. A. Alman, Macrophage cells secrete factors including LRP1 that orchestrate the rejuvenation of bone repair in mice, Nat. Commun., 2018, 9(1), 5191 CrossRef PubMed.
- J. Zhang, Y. Lin, C. Li, X. Zhang, L. Cheng, L. Dai, Y. Wang, F. Wang, G. Shi, Y. Li, Q. Yang, X. Cui, Y. Liu, H. Wang, S. Zhang, Y. Yang, R. Xiang, J. Li, D. Yu, Y. Wei and H. Deng, IL-35 Decelerates the Inflammatory Process by Regulating Inflammatory Cytokine Secretion and M1/M2 Macrophage Ratio in Psoriasis, J. Immunol., 2016, 197(6), 2131–2144 CrossRef CAS PubMed.
- C. Dou, N. Ding, C. Zhao, T. Hou, F. Kang, Z. Cao, C. Liu, Y. Bai, Q. Dai, Q. Ma, F. Luo, J. Xu and S. Dong, Estrogen Deficiency-Mediated M2 Macrophage Osteoclastogenesis Contributes to M1/M2 Ratio Alteration in Ovariectomized Osteoporotic Mice, J. Bone Miner. Res., 2018, 33(5), 899–908 CrossRef CAS PubMed.
- M. Saraiva and A. O'Garra, The regulation of IL-10 production by immune cells, Nat. Rev. Immunol., 2010, 10(3), 170–181 CrossRef CAS PubMed.
- M. Zhang, Y. Li, P. Rao, K. Huang, D. Luo, X. Cai and J. Xiao, Blockade of receptors of advanced glycation end products ameliorates diabetic osteogenesis of adipose-derived stem cells through DNA methylation and Wnt signalling pathway, Cell Proliferation, 2018, 51(5), e12471 CrossRef PubMed.
- F. Wang, L. Kong, W. Wang, L. Shi, M. Wang, Y. Chai, J. Xu and Q. Kang, Adrenomedullin 2 improves bone regeneration in type 1 diabetic rats by restoring imbalanced macrophage polarization and impaired osteogenesis, Stem Cell Res. Ther., 2021, 12(1), 288 CrossRef CAS PubMed.
- W. Qiao, H. Xie, J. Fang, J. Shen, W. Li, D. Shen, J. Wu, S. Wu, X. Liu, Y. Zheng, K. M. C. Cheung and K. W. K. Yeung, Sequential activation of heterogeneous macrophage phenotypes is essential for biomaterials-induced bone regeneration, Biomaterials, 2021, 276, 121038 CrossRef CAS PubMed.
- D. Huang, K. Xu, X. Huang, N. Lin, Y. Ye, S. Lin, J. Zhang, J. Shao, S. Chen, M. Shi, X. Zhou, P. Lin, Y. Xue, C. Yu, X. Yu, Z. Ye and K. Cheng, Remotely Temporal Scheduled Macrophage Phenotypic Transition Enables Optimized Immunomodulatory Bone Regeneration, Small, 2022, 18(39), e2203680 CrossRef PubMed.
- L. Guo, A. Iida, G. S. Bhavani, K. Gowrishankar, Z. Wang, J. Y. Xue, J. Wang, N. Miyake, N. Matsumoto, T. Hasegawa, Y. Iizuka, M. Matsuda, T. Nakashima, M. Takechi, S. Iseki, S. Yambe, G. Nishimura, H. Koseki, C. Shukunami, K. M. Girisha and S. Ikegawa, Deficiency of TMEM53 causes a previously unknown sclerosing bone disorder by dysregulation of BMP-SMAD signaling, Nat. Commun., 2021, 12(1), 2046 CrossRef CAS PubMed.
- R. L. Huang, Y. Yuan, J. Tu, G. M. Zou and Q. Li, Opposing TNF-alpha/IL-1beta- and BMP-2-activated MAPK signaling pathways converge on Runx2 to regulate BMP-2-induced osteoblastic differentiation, Cell Death Dis., 2014, 5, e1187 CrossRef CAS PubMed.
- L. Vi, G. S. Baht, H. Whetstone, A. Ng, Q. Wei, R. Poon, S. Mylvaganam, M. Grynpas and B. A. Alman, Macrophages promote osteoblastic differentiation in-vivo: implications in fracture repair and bone homeostasis, J. Bone Miner. Res., 2015, 30(6), 1090–1102 CrossRef CAS PubMed.
- J. Stefanowski, A. Lang, A. Rauch, L. Aulich, M. Kohler, A. F. Fiedler, F. Buttgereit, K. Schmidt-Bleek, G. N. Duda, T. Gaber, R. A. Niesner and A. E. Hauser, Spatial Distribution of Macrophages During Callus Formation and Maturation Reveals Close Crosstalk Between Macrophages and Newly Forming Vessels, Front. Immunol., 2019, 10, 2588 CrossRef CAS PubMed.
- A. Liu, S. Jin, C. Fu, S. Cui, T. Zhang, L. Zhu, Y. Wang, S. G. F. Shen, N. Jiang and Y. Liu, Macrophage-derived small extracellular vesicles promote biomimetic mineralized collagen-mediated endogenous bone regeneration, Int. J. Oral Sci., 2020, 12(1), 33 CrossRef CAS PubMed.
- L. Dong and C. Wang, Harnessing the power of macrophages/monocytes for enhanced bone tissue engineering, Trends Biotechnol., 2013, 31(6), 342–346 CrossRef CAS PubMed.
- G. L. Burn, A. Foti, G. Marsman, D. F. Patel and A. Zychlinsky, The Neutrophil, Immunity, 2021, 54(7), 1377–1391 CrossRef CAS PubMed.
- A. Cui, M. Xiang, M. Xu, P. Lu, S. Wang, Y. Zou, K. Qiao, C. Jin, Y. Li, M. Lu, A. F. Chen and S. Chen, VCAM-1-mediated neutrophil infiltration exacerbates ambient fine particle-induced lung injury, Toxicol. Lett., 2019, 302, 60–74 CrossRef CAS PubMed.
- C. L. Holmes, D. Shim, J. Kernien, C. J. Johnson, J. E. Nett and M. A. Shelef, Insight into Neutrophil Extracellular Traps through Systematic Evaluation of Citrullination and Peptidylarginine Deiminases, J. Immunol. Res., 2019, 2019, 2160192 Search PubMed.
- A. Kovtun, S. Bergdolt, R. Wiegner, P. Radermacher, M. Huber-Lang and A. Ignatius, The crucial role of neutrophil granulocytes in bone fracture healing, Eur. Cells Mater., 2016, 32, 152–162 CrossRef CAS PubMed.
- D. F. Quail, B. Amulic, M. Aziz, B. J. Barnes, E. Eruslanov, Z. G. Fridlender, H. S. Goodridge, Z. Granot, A. Hidalgo, A. Huttenlocher, M. J. Kaplan, I. Malanchi, T. Merghoub, E. Meylan, V. Mittal, M. J. Pittet, A. Rubio-Ponce, I. A. Udalova, T. K. van den Berg, D. D. Wagner, P. Wang, A. Zychlinsky, K. E. de Visser, M. Egeblad and P. Kubes, Neutrophil phenotypes and functions in cancer: A consensus statement, J. Exp. Med., 2022, 219(6), e20220011 CrossRef CAS PubMed.
- Z. Zhao, T. Liu, Y. Liang, W. Cui, D. Li, G. Zhang, Z. Deng, M. Chen, K. Sha, W. Xiao, H. Xie and J. Li, N2-Polarized Neutrophils Reduce Inflammation in Rosacea by Regulating Vascular Factors and Proliferation of CD4+ T Cells, J. Invest. Dermatol., 2022, 142(7), 1835–1844 CrossRef CAS PubMed.
- B. Cai, D. Lin, Y. Li, L. Wang, J. Xie, T. Dai, F. Liu, M. Tang, L. Tian, Y. Yuan, L. Kong and S. G. F. Shen, N2-Polarized Neutrophils Guide Bone Mesenchymal Stem Cell Recruitment and Initiate Bone Regeneration: A Missing Piece of the Bone Regeneration Puzzle, Adv. Sci., 2021, 8(19), e2100584 CrossRef PubMed.
- B. Pinegin, N. Vorobjeva and V. Pinegin, Neutrophil extracellular traps and their role in the development of chronic inflammation and autoimmunity, Autoimmun. Rev., 2015, 14(7), 633–640 CrossRef CAS PubMed.
- N. Krohn, S. Kapoor, Y. Enami, A. Follenzi, S. Bandi, B. Joseph and S. Gupta, Hepatocyte transplantation-induced liver inflammation is driven by cytokines-chemokines associated with neutrophils and Kupffer cells, Gastroenterology, 2009, 136(5), 1806–1817 CrossRef CAS PubMed.
- M. E. J. Taekema-Roelvink, C. V. Kooten, S. V. Kooij, E. Heemskerk and M. R. Daha, Proteinase 3 enhances endothelial monocyte chemoattractant protein-1 production and induces increased adhesion of neutrophils to endothelial cells by upregulating
intercellular cell adhesion molecule-1, J. Am. Soc. Nephrol., 2001, 12(5), 932–940 CrossRef CAS PubMed.
- W. W. Li, T. Z. Guo, D. Y. Liang, Y. Sun, W. S. Kingery and J. D. Clark, Substance P signaling controls mast cell activation, degranulation, and nociceptive sensitization in a rat fracture model of complex regional pain syndrome, Anesthesiology, 2012, 116(4), 882–895 CrossRef CAS PubMed.
- D. Ragipoglu, J. Bulow, K. Hauff, M. Voss, M. Haffner-Luntzer, A. Dudeck, A. Ignatius and V. Fischer, Mast Cells Drive Systemic Inflammation and Compromised Bone Repair After Trauma, Front. Immunol., 2022, 13, 883707 CrossRef CAS PubMed.
- D. A. Behrends, L. Cheng, M. B. Sullivan, M. H. Wang, G. B. Roby, N. Zayed, C. Gao, J. E. Henderson and P. A. Martineau, Defective bone repair in mast cell deficient mice with c-Kit loss of function, Eur. Cells Mater., 2014, 28, 209–221 CrossRef CAS PubMed ; discussion 221–202.
- J. L. Ramirez-GarciaLuna, D. Chan, R. Samberg, M. Abou-Rjeili, T. H. Wong, A. Li, T. B. Feyerabend, H. R. Rodewald, J. E. Henderson and P. A. Martineau, Defective bone repair in mast cell-deficient Cpa3Cre/+mice, PLoS One, 2017, 12(3), e0174396 CrossRef PubMed.
- V. Fischer, D. Ragipoglu, J. Diedrich, L. Steppe, A. Dudeck, K. Schutze, M. Kalbitz, F. Gebhard, M. Haffner-Luntzer and A. Ignatius, Mast Cells Trigger Disturbed Bone Healing in Osteoporotic Mice, J. Bone Miner. Res., 2022, 37(1), 137–151 CrossRef CAS PubMed.
- M. N. Weitzmann, T. Vikulina, S. Roser-Page, M. Yamaguchi and I. Ofotokun, Homeostatic Expansion of CD4+ T Cells Promotes Cortical and Trabecular Bone Loss, Whereas CD8+ T Cells Induce Trabecular Bone Loss Only, J. Infect. Dis., 2017, 216(9), 1070–1079 CrossRef CAS PubMed.
- M. Bernhardsson, F. Dietrich-Zagonel, L. Tatting, P. Eliasson and P. Aspenberg, Depletion of cytotoxic (CD8+) T cells impairs implant fixation in rat cancellous bone, J. Orthop. Res., 2019, 37(4), 805–811 CrossRef CAS PubMed.
- C. Schlundt, S. Reinke, S. Geissler, C. H. Bucher, C. Giannini, S. Mardian, M. Dahne, C. Kleber, B. Samans, U. Baron, G. N. Duda, H. D. Volk and K. Schmidt-Bleek, Individual Effector/Regulator T Cell Ratios Impact Bone Regeneration, Front. Immunol., 2019, 10, 1954 CrossRef CAS PubMed.
- D. Nam, E. Mau, Y. Wang, D. Wright, D. Silkstone, H. Whetstone, C. Whyne and B. Alman, T-lymphocytes enable osteoblast maturation via IL-17F during the early phase of fracture repair, PLoS One, 2012, 7(6), e40044 CrossRef CAS PubMed.
- J. Tan, A. Dai, L. Pan, L. Zhang, Z. Wang, T. Ke, W. Sun, Y. Wu, P. H. Ding and L. Chen, Inflamm-Aging-Related Cytokines of IL-17 and IFN-gamma Accelerate Osteoclastogenesis and Periodontal Destruction, J. Immunol. Res., 2021, 2021, 9919024 Search PubMed.
- L. Claes, S. Recknagel and A. Ignatius, Fracture healing under healthy and inflammatory conditions, Nat. Rev. Rheumatol., 2012, 8(3), 133–143 CrossRef CAS PubMed.
- J. Li, S. Y. Kim, N. M. Lainez, D. Coss and M. G. Nair, Macrophage-Regulatory T Cell Interactions Promote Type 2 Immune Homeostasis Through Resistin-Like Molecule alpha, Front. Immunol., 2021, 12, 710406 CrossRef CAS PubMed.
- Y. Zhang, J. Lazarus, N. G. Steele, W. Yan, H. J. Lee, Z. C. Nwosu, C. J. Halbrook, R. E. Menjivar, S. B. Kemp, V. R. Sirihorachai, A. Velez-Delgado, K. Donahue, E. S. Carpenter, K. L. Brown, V. Irizarry-Negron, A. C. Nevison, A. Vinta, M. A. Anderson, H. C. Crawford, C. A. Lyssiotis, T. L. Frankel, F. Bednar and M. Pasca di Magliano, Regulatory T-cell Depletion Alters the Tumor Microenvironment and Accelerates Pancreatic Carcinogenesis, Cancer Discovery, 2020, 10(3), 422–439 CrossRef CAS PubMed.
- L. Zhu, F. Hua, W. Ding, K. Ding, Y. Zhang and C. Xu, The correlation between the Th17/Treg cell balance and bone health, Immun. Ageing, 2020, 17, 30 CrossRef CAS PubMed.
- L. Batoon, S. M. Millard, L. J. Raggatt, C. Sandrock, E. Pickering, K. Williams, L. W. H. Sun, A. C. Wu, K. M. Irvine, P. Pivonka, V. Glatt, M. E. Wullschleger, D. A. Hume and A. R. Pettit, Treatment with a long-acting chimeric CSF1 molecule enhances fracture healing of healthy and osteoporotic bones, Biomaterials, 2021, 275, 120936 CrossRef CAS PubMed.
- P. Vantourout and A. Hayday, Six-of-the-best: unique contributions of gammadelta T cells to immunology, Nat. Rev. Immunol., 2013, 13(2), 88–100 CrossRef CAS PubMed.
- S. Kalyan, It May Seem Inflammatory, but Some T Cells Are Innately Healing to the Bone, J. Bone Miner. Res., 2016, 31(11), 1997–2000 CrossRef CAS PubMed.
- T. Ono, K. Okamoto, T. Nakashima, T. Nitta, S. Hori, Y. Iwakura and H. Takayanagi, IL-17-producing gammadelta T cells enhance bone regeneration, Nat. Commun., 2016, 7, 10928 CrossRef CAS PubMed.
- X. Zhu, Z. Zeng, D. Qiu and J. Chen, Vgamma9Vdelta2 T cells inhibit immature dendritic cell transdifferentiation into osteoclasts through downregulation of RANK, cFos and ATP6V0D2, Int. J. Mol. Med., 2018, 42(4), 2071–2079 CAS.
- H. Zhang, R. Wang, G. Wang, B. Zhang, C. Wang, D. Li, C. Ding, Q. Wei, Z. Fan, H. Tang and F. Ji, Single-Cell RNA Sequencing Reveals B Cells Are Important Regulators in Fracture Healing, Front. Endocrinol., 2021, 12, 666140 CrossRef PubMed.
- B. Li, P. Wang, J. Jiao, H. Wei, W. Xu and P. Zhou, Roles of the RANKL-RANK Axis in Immunity-Implications for Pathogenesis and Treatment of Bone Metastasis, Front. Immunol., 2022, 13, 824117 CrossRef CAS PubMed.
- W. C. Dougall, Molecular pathways: osteoclast-dependent and osteoclast-independent roles of the RANKL/RANK/OPG pathway in tumorigenesis and metastasis, Clin. Cancer Res., 2012, 18(2), 326–335 CrossRef CAS PubMed.
- Y. Li, G. Toraldo, A. Li, X. Yang, H. Zhang, W. P. Qian and M. N. Weitzmann, B cells and T cells are critical for the preservation of bone homeostasis and attainment of peak bone mass in vivo, Blood, 2007, 109(9), 3839–3848 CrossRef CAS PubMed.
- H. Chagraoui, M. Tulliez, T. Smayra, E. Komura, S. Giraudier, T. Yun, N. Lassau, W. Vainchenker and F. Wendling, Stimulation of osteoprotegerin production is responsible for osteosclerosis in mice overexpressing TPO, Blood, 2003, 101(8), 2983–2989 CrossRef CAS PubMed.
- K. S. Lee, J. Lee, H. K. Kim, S. H. Yeom, C. H. Woo, Y. J. Jung, Y. E. Yun, S. Y. Park, J. Han, E. Kim, J. H. Sul, J. M. Jung, J. H. Park, J. S. Choi, Y. W. Cho and D. G. Jo, Extracellular vesicles from adipose tissue-derived stem cells alleviate osteoporosis through osteoprotegerin and miR-21-5p, J. Extracell. Vesicles, 2021, 10(12), e12152 CAS.
- J. A. Burger and A. Wiestner, Targeting B cell receptor signalling in cancer: preclinical and clinical advances, Nat. Rev. Cancer, 2018, 18(3), 148–167 CrossRef CAS PubMed.
- R. F. Wynn, C. A. Hart, C. Corradi-Perini, L. O'Neill, C. A. Evans, J. E. Wraith, L. J. Fairbairn and I. Bellantuono, A small proportion of mesenchymal stem cells strongly expresses functionally active CXCR4 receptor capable of promoting migration to bone marrow, Blood, 2004, 104(9), 2643–2645 CrossRef CAS PubMed.
- M. Huber-Lang, A. Kovtun and A. Ignatius, The role of complement in trauma and fracture healing, Semin. Immunol., 2013, 25(1), 73–78 CrossRef CAS PubMed.
- C. I. Marinescu, M. B. Preda and A. Burlacu, A procedure for in vitro evaluation of the immunosuppressive effect of mouse mesenchymal stem cells on activated T cell proliferation, Stem Cell Res. Ther., 2021, 12(1), 319 CrossRef CAS PubMed.
- F. Liu, H. Qiu, M. Xue, S. Zhang, X. Zhang, J. Xu, J. Chen, Y. Yang and J. Xie, MSC-secreted TGF-beta regulates lipopolysaccharide-stimulated macrophage M2-like polarization via the Akt/FoxO1 pathway, Stem Cell Res. Ther., 2019, 10(1), 345 CrossRef CAS PubMed.
- G. Ren, L. Zhang, X. Zhao, G. Xu, Y. Zhang, A. I. Roberts, R. C. Zhao and Y. Shi, Mesenchymal stem cell-mediated immunosuppression occurs via concerted action of chemokines and nitric oxide, Cell Stem Cell, 2008, 2(2), 141–150 CrossRef CAS PubMed.
- W. J. M. Mulder, J. Ochando, L. A. B. Joosten, Z. A. Fayad and M. G. Netea, Therapeutic targeting of trained immunity, Nat. Rev. Drug Discovery, 2019, 18(7), 553–566 CrossRef CAS PubMed.
- X. Bai, Q. Pei, C. Pu, Y. Chen, S. He and B. Wang, Multifunctional Islet Transplantation Hydrogel Encapsulating A20 High-Expressing Islets, Drug Des., Dev. Ther., 2020, 14, 4021–4027 CrossRef CAS PubMed.
- C. Vitale, M. Marzagalli, S. Scaglione, A. Dondero, C. Bottino and R. Castriconi, Tumor Microenvironment and Hydrogel-Based 3D Cancer Models for In Vitro Testing Immunotherapies, Cancers, 2022, 14(4), 1013 CrossRef CAS PubMed.
- Y. Yin, X. Li, H. Ma, J. Zhang, D. Yu, R. Zhao, S. Yu, G. Nie and H. Wang, In Situ Transforming RNA Nanovaccines from Polyethylenimine Functionalized Graphene Oxide Hydrogel for Durable Cancer Immunotherapy, Nano Lett., 2021, 21(5), 2224–2231 CrossRef CAS PubMed.
- O. Veiseh, J. C. Doloff, M. Ma, A. J. Vegas, H. H. Tam, A. R. Bader, J. Li, E. Langan, J. Wyckoff, W. S. Loo, S. Jhunjhunwala, A. Chiu, S. Siebert, K. Tang, J. Hollister-Lock, S. Aresta-Dasilva, M. Bochenek, J. Mendoza-Elias, Y. Wang, M. Qi, D. M. Lavin, M. Chen, N. Dholakia, R. Thakrar, I. Lacik, G. C. Weir, J. Oberholzer, D. L. Greiner, R. Langer and D. G. Anderson, Size- and shape-dependent foreign body immune response to materials implanted in rodents and non-human primates, Nat. Mater., 2015, 14(6), 643–651 CrossRef CAS PubMed.
- J. Alijotas-Reig and V. Garcia-Gimenez, Delayed immune-mediated adverse effects related to hyaluronic acid and acrylic hydrogel dermal fillers: clinical findings, long-term follow-up and review of the literature, J. Eur. Acad. Dermatol. Venereol., 2008, 22(2), 150–161 CAS.
- S. Ferber, R. J. Gonzalez, A. M. Cryer, U. H. von Andrian and N. Artzi, Immunology-Guided Biomaterial Design for Mucosal Cancer Vaccines, Adv. Mater., 2020, 32(13), e1903847 CrossRef PubMed.
- W. Chen, B. C. Yung, Z. Qian and X. Chen, Improving long-term subcutaneous drug delivery by regulating material-bioenvironment interaction, Adv. Drug Delivery Rev., 2018, 127, 20–34 CrossRef CAS PubMed.
- Y. Liu, Y. Geng, B. Yue, P. C. Lo, J. Huang and H. Jin, Injectable Hydrogel as a Unique Platform for Antitumor Therapy Targeting Immunosuppressive Tumor Microenvironment, Front. Immunol., 2021, 12, 832942 CrossRef CAS PubMed.
- B. Kovacevic, M. Jones, C. Ionescu, D. Walker, S. Wagle, J. Chester, T. Foster, D. Brown, M. Mikov, A. Mooranian and H. Al-Salami, The emerging role of bile acids as critical components in nanotechnology and bioengineering: Pharmacology, formulation optimizers and hydrogel-biomaterial applications, Biomaterials, 2022, 283, 121459 CrossRef CAS PubMed.
- P. Madhusudanan, G. Raju and S. Shankarappa, Hydrogel systems and their role in neural tissue engineering, J. R. Soc., Interface, 2020, 17(162), 20190505 CrossRef CAS PubMed.
- R. Cui, Q. Wu, J. Wang, X. Zheng, R. Ou, Y. Xu, S. Qu and D. Li, Hydrogel-By-Design: Smart Delivery System for Cancer Immunotherapy, Front. Bioeng. Biotechnol., 2021, 9, 723490 CrossRef PubMed.
- C. Wang, J. Wang, X. Zhang, S. Yu, D. Wen, Q. Hu, Y. Ye, H. Bomba, X. Hu, Z. Liu, G. Dotti and Z. Gu, In situ formed reactive oxygen species-responsive scaffold with gemcitabine and checkpoint inhibitor for combination therapy, Sci. Transl. Med., 2018, 10(429), eaan3682 CrossRef PubMed.
- S. Nadine, C. R. Correia and J. F. Mano, Engineering immunomodulatory hydrogels and cell-laden systems towards bone regeneration, Biomater. Adv., 2022, 140, 213058 CrossRef CAS PubMed.
- D. R. Griffin, M. M. Archang, C. H. Kuan, W. M. Weaver, J. S. Weinstein, A. C. Feng, A. Ruccia, E. Sideris, V. Ragkousis, J. Koh, M. V. Plikus, D. Di Carlo, T. Segura and P. O. Scumpia, Activating an adaptive immune response from a hydrogel scaffold imparts regenerative wound healing, Nat. Mater., 2021, 20(4), 560–569 CrossRef CAS PubMed.
- A. Schudel, D. M. Francis and S. N. Thomas, Material design for lymph node drug delivery, Nat. Rev. Mater., 2019, 4(6), 415–428 CrossRef PubMed.
- N. Ma and Z. Yan, Research Progress of Thermosensitive Hydrogel in Tumor Therapeutic, Nanoscale Res. Lett., 2021, 16(1), 42 CrossRef CAS PubMed.
- P. Edwards, M. Arango, L. Balica, R. Cottingham, H. El-Sayed, B. Farrell, J. Fernandes, T. Gogichaisvili, N. Golden, B. Hartzenberg, M. Husain, M. I. Ulloa, Z. Jerbi, H. Khamis, E. Komolafe, V. Laloe, G. Lomas, S. Ludwig, G. Mazairac, L. Munoz Sanchez Mde, L. Nasi, F. Olldashi, P. Plunkett, I. Roberts, P. Sandercock, H. Shakur, C. Soler, R. Stocker, P. Svoboda, S. Trenkler, N. K. Venkataramana, J. Wasserberg, D. Yates, S. Yutthakasemsunt and CRASH trial collaborators, Final results of MRC CRASH, a randomised placebo-controlled trial of intravenous corticosteroid in adults with head injury-outcomes at 6 months, Lancet, 2005, 365(9475), 1957–1959 CrossRef PubMed.
- F. Rizzo and N. S. Kehr, Recent Advances in Injectable Hydrogels for Controlled and Local Drug Delivery, Adv. Healthc. Mater., 2021, 10(1), e2001341 CrossRef PubMed.
- H. Wang, Q. Chen and S. Zhou, Carbon-based hybrid nanogels: a synergistic nanoplatform for combined biosensing, bioimaging, and responsive drug delivery, Chem. Soc. Rev., 2018, 47(11), 4198–4232 RSC.
- Z. Li, G. Li, J. Xu, C. Li, S. Han, C. Zhang, P. Wu, Y. Lin, C. Wang, J. Zhang and X. Li, Hydrogel Transformed from Nanoparticles for Prevention of Tissue Injury and Treatment of Inflammatory Diseases, Adv. Mater., 2022, 34(16), e2109178 CrossRef PubMed.
- J. S. Basuki, F. Qie, X. Mulet, R. Suryadinata, A. V. Vashi, Y. Y. Peng, L. Li, X. Hao, T. Tan and T. C. Hughes, Photo-Modulated Therapeutic Protein Release from a Hydrogel Depot Using Visible Light, Angew. Chem., Int. Ed., 2017, 56(4), 966–971 CrossRef CAS PubMed.
- H. Jung, M. K. Kim, J. Y. Lee, S. W. Choi and J. Kim, Adhesive Hydrogel Patch with Enhanced Strength and Adhesiveness to Skin for Transdermal Drug Delivery, Adv. Funct. Mater., 2020, 30(42), 2004407 CrossRef CAS.
- Q. X. Chen, J. Y. Li, F. Han, Q. C. Meng, H. Wang, W. Qiang, Z. X. Li, F. F. Li, E. Xie, X. Y. Qin, S. Chen, W. S. Wang, C. Y. Liu, B. Li and F. X. Han, A Multifunctional Composite Hydrogel That Rescues the ROS Microenvironment and Guides the Immune Response for Repair of Osteoporotic Bone Defects, Adv. Funct. Mater., 2022, 32(27), 2201067 CrossRef CAS.
- J. Li and D. J. Mooney, Designing hydrogels for controlled drug delivery, Nat. Rev. Mater., 2016, 1(12), 16071 CrossRef CAS PubMed.
- J. Chen, D. Wang, L. H. Wang, W. Liu, A. Chiu, K. Shariati, Q. Liu, X. Wang, Z. Zhong, J. Webb, R. E. Schwartz, N. Bouklas and M. Ma, An Adhesive Hydrogel with “Load-Sharing” Effect as Tissue Bandages for Drug and Cell Delivery, Adv. Mater., 2020, 32(43), e2001628 CrossRef PubMed.
- D. L. Cao, X. Chen, F. Cao, W. Guo, J. Y. Tang, C. Y. Cai, S. Q. Cui, X. W. Yang, L. Yu, Y. Su and J. D. Ding, An Intelligent Transdermal Formulation of ALA-Loaded Copolymer Thermogel with Spontaneous Asymmetry by Using Temperature-Induced Sol-Gel Transition and Gel-Sol (Suspension) Transition on Different Sides, Adv. Funct. Mater., 2021, 31(22), 2100349 CrossRef CAS.
- M. J. Webber and E. T. Pashuck, (Macro)molecular self-assembly for hydrogel drug delivery, Adv. Drug Delivery Rev., 2021, 172, 275–295 CrossRef CAS PubMed.
- Q. Feng, K. Wei, S. Lin, Z. Xu, Y. Sun, P. Shi, G. Li and L. Bian, Mechanically resilient, injectable, and bioadhesive supramolecular gelatin hydrogels crosslinked by weak host-guest interactions assist cell infiltration and in situ tissue regeneration, Biomaterials, 2016, 101, 217–228 CrossRef CAS PubMed.
- Q. Feng, J. Xu, K. Zhang, H. Yao, N. Zheng, L. Zheng, J. Wang, K. Wei, X. Xiao, L. Qin and L. Bian, Dynamic and Cell-Infiltratable Hydrogels as Injectable Carrier of Therapeutic Cells and Drugs for Treating Challenging Bone Defects, ACS Cent. Sci., 2019, 5(3), 440–450 CrossRef CAS PubMed.
- N. Chen, H. Wang, C. Ling, W. Vermerris, B. Wang and Z. Tong, Cellulose-based injectable hydrogel composite for pH-responsive and controllable drug delivery, Carbohydr. Polym., 2019, 225, 115207 CrossRef CAS PubMed.
- Q. Feng, S. Lin, K. Zhang, C. Dong, T. Wu, H. Huang, X. Yan, L. Zhang, G. Li and L. Bian, Sulfated hyaluronic acid hydrogels with retarded degradation and enhanced growth factor retention promote hMSC chondrogenesis and articular cartilage integrity with reduced hypertrophy, Acta Biomater., 2017, 53, 329–342 CrossRef CAS PubMed.
- X. Xu, A. K. Jha, R. L. Duncan and X. Jia, Heparin-decorated, hyaluronic acid-based hydrogel particles for the controlled release of bone morphogenetic protein 2, Acta Biomater., 2011, 7(8), 3050–3059 CrossRef CAS PubMed.
- Y. Liu, J. Yang, Z. Luo, D. Li, J. Lu, Q. Wang, Y. Xiao and X. Zhang, Development of an injectable thiolated icariin functionalized collagen/hyaluronic hydrogel to promote cartilage formation in vitro and in vivo, J. Mater. Chem. B, 2019, 7(17), 2845–2854 RSC.
- J. Yang, Y. Liu, L. He, Q. Wang, L. Wang, T. Yuan, Y. Xiao, Y. Fan and X. Zhang, Icariin conjugated hyaluronic acid/collagen hydrogel for osteochondral interface restoration, Acta Biomater., 2018, 74, 156–167 CrossRef CAS PubMed.
- R. X. Li, Y. Sun, Z. W. Cai, Y. Li, J. Sun, W. Bi, F. Yang, Q. R. Zhou, T. J. Ye and Y. C. Yu, Highly bioactive peptide-HA photo-crosslinking hydrogel for sustained promoting bone regeneration, Chem. Eng. J., 2021, 415, 129015 CrossRef CAS.
- R. Wang, L. Che, Q. Feng and K. Cai, Tough, Flexible, and Bioactive Amphoteric Copolymer-Based Hydrogel for Bone Regeneration without Encapsulation of Seed Cells/Simulating Cues, ACS Appl. Mater. Interfaces, 2022, 14(10), 12038–12049 CrossRef CAS PubMed.
- P. Wang, J. Sun, Z. Lou, F. Fan, K. Hu, Y. Sun and N. Gu, Assembly-Induced Thermogenesis of Gold Nanoparticles in the Presence of Alternating Magnetic Field for Controllable Drug Release of Hydrogel, Adv. Mater., 2016, 28(48), 10801–10808 CrossRef CAS PubMed.
- L. B. Jiang, S. L. Ding, W. Ding, D. H. Su, F. X. Zhang, T. W. Zhang, X. F. Yin, L. Xiao, Y. L. Li, F. L. Yuan and J. Dong, Injectable sericin based nanocomposite hydrogel for multi-modal imaging-guided immunomodulatory bone regeneration, Chem. Eng. J., 2021, 418, 129323 CrossRef CAS.
- D. A. Ossipov, A. B. Romero and E. Ossipova, Light-activatable prodrugs based on hyaluronic acid biomaterials, Carbohydr. Polym., 2018, 180, 145–155 CrossRef CAS PubMed.
- C. Gao, J. Ren, C. Zhao, W. Kong, Q. Dai, Q. Chen, C. Liu and R. Sun, Xylan-based temperature/pH sensitive hydrogels for drug controlled release, Carbohydr. Polym., 2016, 151, 189–197 CrossRef CAS PubMed.
- W. Zhou, Z. Duan, J. Zhao, R. Fu, C. Zhu and D. Fan, Glucose and MMP-9 dual-responsive hydrogel with temperature sensitive self-adaptive shape and controlled drug release accelerates diabetic wound healing, Bioact. Mater., 2022, 17, 1–17 CrossRef CAS PubMed.
- W. Zhang, X. Jin, H. Li, R. R. Zhang and C. W. Wu, Injectable and body temperature sensitive hydrogels based on chitosan and hyaluronic acid for pH sensitive drug release, Carbohydr. Polym., 2018, 186, 82–90 CrossRef CAS PubMed.
- J. Wang, Y. Ye, J. Yu, A. R. Kahkoska, X. Zhang, C. Wang, W. Sun, R. D. Corder, Z. Chen, S. A. Khan, J. B. Buse and Z. Gu, Core-Shell Microneedle Gel for Self-Regulated Insulin Delivery, ACS Nano, 2018, 12(3), 2466–2473 CrossRef CAS PubMed.
- H. Newman, Y. V. Shih and S. Varghese, Resolution of inflammation in bone regeneration: From understandings to therapeutic applications, Biomaterials, 2021, 277, 121114 CrossRef CAS PubMed.
- J. Zhang, H. Shi, N. Zhang, L. Hu, W. Jing and J. Pan, Interleukin-4-loaded hydrogel scaffold regulates macrophages polarization to promote bone mesenchymal stem cells osteogenic differentiation via TGF-beta1/Smad pathway for repair of bone defect, Cell Proliferation, 2020, 53(10), e12907 CrossRef CAS PubMed.
- M. Zou, J. Sun and Z. Xiang, Induction of M2-Type Macrophage Differentiation for Bone Defect Repair via an Interpenetration Network Hydrogel with a GO-Based Controlled Release System, Adv. Healthc. Mater., 2021, 10(6), e2001502 CrossRef PubMed.
- J. R. Krieger, M. E. Ogle, J. McFaline-Figueroa, C. E. Segar, J. S. Temenoff and E. A. Botchwey, Spatially localized recruitment of anti-inflammatory monocytes by SDF-1alpha-releasing hydrogels enhances microvascular network remodeling, Biomaterials, 2016, 77, 280–290 CrossRef CAS PubMed.
- J. P. O'Connor, M. B. Manigrasso, B. D. Kim and S. Subramanian, Fracture healing and lipid mediators, BoneKEy Rep., 2014, 3, 517 Search PubMed.
- X. Jiang, J. Liu, S. Li, Y. Qiu, X. Wang, X. He, T. O. Pedersen, K. Mustafa, Y. Xue, M. Mustafa, A. Kantarci and Z. Xing, The effect of resolvin D1 on bone regeneration in a rat calvarial defect model, J. Tissue Eng. Regener. Med., 2022, 16(11), 987–997 CrossRef CAS PubMed.
- N. Chauhan, P. Gupta, L. Arora, D. Pal and Y. Singh, Dexamethasone-loaded, injectable pullulan-poly(ethylene glycol) hydrogels for bone tissue regeneration in chronic inflammatory conditions, Mater. Sci. Eng., C, 2021, 130, 112463 CrossRef CAS PubMed.
- B. Guo, X. Feng, Y. Wang, X. Wang and Y. He, Biomimetic and immunomodulatory baicalin-loaded graphene oxide-demineralized bone matrix scaffold for in vivo bone regeneration, J. Mater. Chem. B, 2021, 9(47), 9720–9733 RSC.
- Y. Zhang, M. Yan, Q. F. Yu, P. F. Yang, H. D. Zhang, Y. H. Sun, Z. F. Zhang and Y. F. Gao, Puerarin Prevents LPS-Induced Osteoclast Formation and Bone Loss via Inhibition of Akt Activation, Biol. Pharm. Bull., 2016, 39(12), 2028–2035 CrossRef CAS PubMed.
- X. Yang, Y. Yang, S. Zhou, X. Gong, Q. Dai, P. Zhang and L. Jiang, Puerarin Stimulates Osteogenic Differentiation and Bone Formation Through the ERK1/2 and p38-MAPK Signaling Pathways, Curr. Mol. Med., 2018, 17(7), 488–496 CrossRef CAS PubMed.
- M. Qin, J. Jin, Q. Saiding, Y. Xiang, Y. Wang, F. Sousa, B. Sarmento, W. Cui and X. Chen, In situ inflammatory-regulated drug-loaded hydrogels for promoting pelvic floor repair, J. Controlled Release, 2020, 322, 375–389 CrossRef CAS PubMed.
- G. Moroncini, G. Calogera, D. Benfaremo and A. Gabrielli, Biologics in Inflammatory Immune-mediated Systemic Diseases, Curr. Pharm. Biotechnol., 2017, 18(12), 1008–1016 CAS.
- A. Corrado, A. Neve, N. Maruotti and F. P. Cantatore, Bone effects of biologic drugs in rheumatoid arthritis, Clin. Dev. Immunol., 2013, 2013, 945945 Search PubMed.
- M. Timmen, H. Hidding, B. Wieskotter, W. Baum, T. Pap, M. J. Raschke, G. Schett, J. Zwerina and R. Stange, Influence of antiTNF-alpha antibody treatment on fracture healing under chronic inflammation, BMC Musculoskeletal Disord., 2014, 15, 184 CrossRef PubMed.
- M. Dixit, K. B. Singh, R. Prakash and D. Singh, Functional block of IL-17 cytokine promotes bone healing by augmenting FOXO1 and ATF4 activity in cortical bone defect model, Osteoporosis Int., 2017, 28(7), 2207–2220 CrossRef CAS PubMed.
- L. Chen, C. Yu, Y. Xiong, K. Chen, P. Liu, A. C. Panayi, X. Xiao, Q. Feng, B. Mi and G. Liu, Multifunctional hydrogel enhances bone regeneration through sustained release of stromal cell-derived factor-1αand exosomes, Bioact. Mater., 2023, 25, 460–471 CrossRef CAS PubMed.
- S. Fahmy-Garcia, D. Mumcuoglu, L. de Miguel, V. Dieleman, J. Witte-Bouma, B. C. J. van der Eerden, M. van Driel, D. Eglin, J. A. N. Verhaar, S. Kluijtmans, G. van Osch and E. Farrell, Novel In Situ Gelling Hydrogels Loaded with Recombinant Collagen Peptide Microspheres as a Slow-Release System Induce Ectopic Bone Formation, Adv. Healthc. Mater., 2018, 7(24), e1801496 CrossRef PubMed.
- M. Vazquez-Gonzalez and I. Willner, Stimuli-Responsive Biomolecule-Based Hydrogels and Their Applications, Angew. Chem., Int. Ed., 2020, 59(36), 15342–15377 CrossRef CAS PubMed.
- H. C. Yan, T. T. Yu, J. Li, Y. Q. Qiao, L. C. Wang, T. Zhang, Q. Li, Y. H. Zhou and D. W. Liu, The Delivery of Extracellular Vesicles Loaded in Biomaterial Scaffolds for Bone Regeneration, Front. Bioeng. Biotechnol., 2020, 8, 1015 CrossRef PubMed.
- M. Kou, L. Huang, J. Yang, Z. Chiang, S. Chen, J. Liu, L. Guo, X. Zhang, X. Zhou, X. Xu, X. Yan, Y. Wang, J. Zhang, A. Xu, H. F. Tse and Q. Lian, Mesenchymal stem cell-derived extracellular vesicles for immunomodulation and regeneration: a next generation therapeutic tool?, Cell Death Dis., 2022, 13(7), 580 CrossRef CAS PubMed.
- O. Ovrebo, G. Perale, J. P. Wojciechowski, C. Echalier, J. R. T. Jeffers, M. M. Stevens, H. J. Haugen and F. Rossi, Design and clinical application of injectable hydrogels for musculoskeletal therapy, Bioeng. Transl. Med., 2022, 7(2), e10295 Search PubMed.
- P. Guan, C. Liu, D. Xie, S. Mao, Y. Ji, Y. Lin, Z. Chen, Q. Wang, L. Fan and Y. Sun, Exosome-loaded extracellular matrix-mimic hydrogel with anti-inflammatory property Facilitates/promotes growth plate injury repair, Bioact. Mater., 2022, 10, 145–158 CrossRef CAS PubMed.
- Z. Shen, S. Kuang, Y. Zhang, M. Yang, W. Qin, X. Shi and Z. Lin, Chitosan hydrogel incorporated with dental pulp stem cell-derived exosomes alleviates periodontitis in mice via a macrophage-dependent mechanism, Bioact. Mater., 2020, 5(4), 1113–1126 CrossRef PubMed.
- M. Kang, C. C. Huang, Y. Lu, S. Shirazi, P. Gajendrareddy, S. Ravindran and L. F. Cooper, Bone regeneration is mediated by macrophage extracellular vesicles, Bone, 2020, 141, 115627 CrossRef CAS PubMed.
- J. Fu, Y. Wang, Y. Jiang, J. Du, J. Xu and Y. Liu, Systemic therapy of MSCs in bone regeneration: a systematic review and meta-analysis, Stem Cell Res. Ther., 2021, 12(1), 377 CrossRef PubMed.
- B. D. Sui, C. H. Hu, A. Q. Liu, C. X. Zheng, K. Xuan and Y. Jin, Stem cell-based bone regeneration in diseased microenvironments: Challenges and solutions, Biomaterials, 2019, 196, 18–30 CrossRef CAS PubMed.
- R. M. Samsonraj, M. Raghunath, V. Nurcombe, J. H. Hui, A. J. van Wijnen and S. M. Cool, Concise Review: Multifaceted Characterization of Human Mesenchymal Stem Cells for Use in Regenerative Medicine, Stem Cells Transl. Med., 2017, 6(12), 2173–2185 CrossRef PubMed.
- J. Dornen and T. Dittmar, The Role of MSCs and Cell Fusion in Tissue Regeneration, Int. J. Mol. Sci., 2021, 22(20), 10980 CrossRef PubMed.
- K. Zha, X. Li, Z. Yang, G. Tian, Z. Sun, X. Sui, Y. Dai, S. Liu and Q. Guo, Heterogeneity of mesenchymal stem cells in cartilage regeneration: from characterization to application, npj Regener. Med., 2021, 6(1), 14 CrossRef CAS PubMed.
- X. Hu, Z. Xia and K. Cai, Recent advances in 3D hydrogel culture systems for mesenchymal stem cell-based therapy and cell behavior regulation, J. Mater. Chem. B, 2022, 10(10), 1486–1507 RSC.
- E. Seebach, H. Freischmidt, J. Holschbach, J. Fellenberg and W. Richter, Mesenchymal stroma cells trigger early attraction of M1 macrophages and endothelial cells into fibrin hydrogels, stimulating long bone healing without long-term engraftment, Acta Biomater., 2014, 10(11), 4730–4741 CrossRef CAS PubMed.
- X. Ji, X. Yuan, L. Ma, B. Bi, H. Zhu, Z. Lei, W. Liu, H. Pu, J. Jiang, X. Jiang, Y. Zhang and J. Xiao, Mesenchymal stem cell-loaded thermosensitive hydroxypropyl chitin hydrogel combined with a three-dimensional-printed poly(epsilon-caprolactone) /nano-hydroxyapatite scaffold to repair bone defects via osteogenesis, angiogenesis and immunomodulation, Theranostics, 2020, 10(2), 725–740 CrossRef CAS PubMed.
- M. Ueno, C. W. Lo, D. Barati, B. Conrad, T. Lin, Y. Kohno, T. Utsunomiya, N. Zhang, M. Maruyama, C. Rhee, E. Huang, M. Romero-Lopez, X. Tong, Z. Yao, S. Zwingenberger, F. Yang and S. B. Goodman, Interleukin-4 overexpressing mesenchymal stem cells within gelatin-based microribbon hydrogels enhance bone healing in a murine long bone critical-size defect model, J. Biomed. Mater. Res., Part A, 2020, 108(11), 2240–2250 CrossRef CAS PubMed.
- Z. Zhuang, Y. Zhang, X. Yang, T. Yu, Y. Zhang, K. Sun, Y. Zhang, F. Cheng, L. Zhang and H. Wang, Matrix stiffness regulates the immunomodulatory effects of mesenchymal stem cells on macrophages via AP1/TSG-6 signaling pathways, Acta Biomater., 2022, 149, 69–81 CrossRef CAS PubMed.
- L. A. Costa, N. Eiro, M. Fraile, L. O. Gonzalez, J. Saa, P. Garcia-Portabella, B. Vega, J. Schneider and F. J. Vizoso, Functional heterogeneity of mesenchymal stem cells from natural niches to culture conditions: implications for further clinical uses, Cell. Mol. Life Sci., 2021, 78(2), 447–467 CrossRef CAS PubMed.
- M. Dymowska, A. Aksamit, K. Zielniok, M. Kniotek, B. Kaleta, A. Roszczyk, M. Zych, F. Dabrowski, L. Paczek and A. Burdzinska, Interaction between Macrophages and Human Mesenchymal Stromal Cells Derived from Bone Marrow and Wharton’s Jelly-A Comparative Study, Pharmaceutics, 2021, 13(11), 1822 CrossRef CAS PubMed.
- L. C. Mariano, M. H. R. Fernandes and P. S. Gomes, Antimicrobial Biomaterials for the Healing of Infected Bone Tissue: A Systematic Review of Microtomographic Data on Experimental Animal Models, J. Funct. Biomater., 2022, 13(4), 193 CrossRef CAS PubMed.
- I. Marriott, Apoptosis-associated uncoupling of bone formation and resorption in osteomyelitis, Front. Cell Infect. Microbiol., 2013, 3, 101 CAS.
- Q. M. Ou, K. Q. Huang, C. Q. Fu, C. L. Huang, Y. F. Fang, Z. P. Gu, J. Wu and Y. Wang, Nanosilver-incorporated halloysite nanotubes/gelatin methacrylate hybrid hydrogel with osteoimmunomodulatory and antibacterial activity for bone regeneration, Chem. Eng. J., 2020, 382, 123019 CrossRef CAS.
- S. Ma, C. Wang, Y. Dong, W. Jing, P. Wei, C. Peng, Z. Liu, B. Zhao and Y. Wang, Microsphere-Gel Composite System with Mesenchymal Stem Cell Recruitment, Antibacterial, and Immunomodulatory Properties Promote Bone Regeneration via Sequential Release of LL37 and W9 Peptides, ACS Appl. Mater. Interfaces, 2022, 14(34), 38525–38540 CrossRef CAS PubMed.
- D. Ronin, J. Boyer, N. Alban, R. M. Natoli, A. Johnson and B. V. Kjellerup, Current and novel diagnostics for orthopedic implant biofilm infections: a review, APMIS, 2022, 130(2), 59–81 CrossRef CAS PubMed.
- Y. Li, X. Liu, B. Li, Y. Zheng, Y. Han, D. F. Chen, K. W. K. Yeung, Z. Cui, Y. Liang, Z. Li, S. Zhu, X. Wang and S. Wu, Near-Infrared Light Triggered Phototherapy and Immunotherapy for Elimination of Methicillin-Resistant Staphylococcus aureus Biofilm Infection on Bone Implant, ACS Nano, 2020, 14(7), 8157–8170 CrossRef CAS PubMed.
- N. Behzadpour, N. Sattarahmady and N. Akbari, Antimicrobial Photothermal Treatment of Pseudomonas Aeruginosa by a Carbon Nanoparticles-Polypyrrole Nanocomposite, J. Biomed. Phys. Eng., 2019, 9(6), 661–672 CAS.
- S. Fan, W. Lin, Y. Huang, J. Xia, J. F. Xu, J. Zhang and J. Pi, Advances and Potentials of Polydopamine Nanosystem in Photothermal-Based Antibacterial Infection Therapies, Front. Pharmacol., 2022, 13, 829712 CrossRef CAS PubMed.
- Y. Liu, P. Bhattarai, Z. Dai and X. Chen, Photothermal therapy and photoacoustic imaging via nanotheranostics in fighting cancer, Chem. Soc. Rev., 2019, 48(7), 2053–2108 RSC.
- A. C. Hernandez-Gonzalez, L. Tellez-Jurado and L. M. Rodriguez-Lorenzo, Alginate hydrogels for bone tissue engineering, from injectables to bioprinting: A review, Carbohydr. Polym., 2020, 229, 115514 CrossRef CAS PubMed.
- N. Olov, S. Bagheri-Khoulenjani and H. Mirzadeh, Injectable hydrogels for bone and cartilage tissue engineering: a review, Prog. Biomater., 2022, 11(2), 113–135 CrossRef PubMed.
- X. Bai, M. Gao, S. Syed, J. Zhuang, X. Xu and X. Q. Zhang, Bioactive hydrogels for bone regeneration, Bioact. Mater., 2018, 3(4), 401–417 CrossRef PubMed.
- Z. Zheng, C. Yu and H. Wei, Injectable Hydrogels as Three-Dimensional Network Reservoirs for Osteoporosis Treatment, Tissue Eng., Part B, 2021, 27(5), 430–454 CrossRef CAS PubMed.
- C. J. Teal, M. H. Hettiaratchi, M. T. Ho, A. Ortin-Martinez, A. N. Ganesh, A. J. Pickering, A. W. Golinski, B. J. Hackel, V. A. Wallace and M. S. Shoichet, Directed Evolution Enables Simultaneous Controlled Release of Multiple Therapeutic Proteins from Biopolymer-Based Hydrogels, Adv. Mater., 2022, e2202612 CrossRef PubMed.
- Y. H. Tsou, J. Khoneisser, P. C. Huang and X. Xu, Hydrogel as a bioactive material to regulate stem cell fate, Bioact. Mater., 2016, 1(1), 39–55 CrossRef PubMed.
- Z. Wei, S. Wang, J. Hirvonen, H. A. Santos and W. Li, Microfluidics Fabrication of Micrometer-Sized Hydrogels with Precisely Controlled Geometries for Biomedical Applications, Adv. Healthc. Mater., 2022, 11(16), e2200846 CrossRef PubMed.
- E. R. Bentley and S. R. Little, Local delivery strategies to restore immune homeostasis in the context of inflammation, Adv. Drug Delivery Rev., 2021, 178, 113971 CrossRef CAS PubMed.
- R. C. Cooper and H. Yang, Hydrogel-based ocular drug delivery systems: Emerging fabrication strategies, applications, and bench-to-bedside manufacturing considerations, J. Controlled Release, 2019, 306, 29–39 CrossRef CAS PubMed.
- H. Zhang, M. Zhang, X. Zhang, Y. Gao, Y. Ma, H. Chen, J. Wan, C. Li, F. Wang and X. Sun, Enhanced postoperative cancer therapy by iron-based hydrogels, Biomater. Res., 2022, 26(1), 19 CrossRef CAS PubMed.
- F. de la Portilla, S. Dios-Barbeito, M. V. Maestre-Sanchez, J. M. Vazquez-Monchul, A. M. Garcia-Cabrera, I. Ramallo and M. L. Reyes-Diaz, Feasibility and safety of calcium alginate hydrogel sealant for the treatment of cryptoglandular fistula-in-ano: phase I/IIa clinical trial, Colorectal Dis., 2021, 23(6), 1499–1506 CrossRef CAS PubMed.
Footnote |
† These authors contributed equally to this work. |
|
This journal is © The Royal Society of Chemistry 2023 |
Click here to see how this site uses Cookies. View our privacy policy here.