DOI:
10.1039/D3CP00307H
(Review Article)
Phys. Chem. Chem. Phys., 2023,
25, 11883-11891
A brief review on solar charging of Zn–air batteries
Received
19th January 2023
, Accepted 12th April 2023
First published on 18th April 2023
Abstract
This brief review reveals the possibility of solar charging of Zn–air batteries. It describes the various configurations that have been adopted in order to employ solar radiation to directly charge Zn–air batteries, paying particular attention to simple constructions with a minimum number of components. Solar charging is differentiated from solar batteries, which are based on a different concept and mainly depend on the variation of the redox level of added electrolytes.
Introduction
The intermittent nature of renewable energy resources as well as the expansion of mobile electronics and mobile energy demand necessitate parallel development of efficient and low-cost energy storage devices. Batteries are of course the obvious and direct means of energy storage and supply. Li-based batteries are the best known and currently in use devices; however, the cost of Li and some environmental and safety concerns have led to the study of other types of batteries. Zn–air batteries are among the most interesting for several reasons: Zn is abundant in the earth crust, therefore, not expensive and does not introduce any environmental or safety issues. Zn has been used to make a few different types of battery, for example, Zn–I2 and ZnBr2, Zn–Ni(OH)2, Zn–air, etc.1,2 One interesting feature of these batteries is that they are based on aqueous electrolytes,2 which offers a further environmental advantage. In the present work, we will focus on Zn–air batteries, the most interesting and the most popular of all Zn-based batteries.2–14 Zn provides a relatively high theoretical specific energy which mounts up to 1086 W h kg−1 and theoretical specific capacity15 of 820 A h kg−1. Zn–air batteries are in principle rechargeable, as it will be analysed here below. Therefore, they may be used to store electric energy. It is always possible to charge a Zn–air battery by using electricity from the grid or by combining it with a renewable energy source.16 This possibility is practical but involves additional connection circuitry and substantial energy losses. Most interesting is the integration of the light conversion component with the electricity storage component in one single device. This possibility has been studied in several recent works and their results are summarized in the present work.
Operation principle of Zn–air batteries
A schematic illustration of the components of a Zn–air battery is given in Fig. 1. It is made of a Zn foil anode and a gas-diffusion cathode both in contact with an alkaline electrolyte. Zn is oxidized to Zn(OH)42− which is finally decomposed to ZnO, liberating 2 electrons. Electrons move through an external circuit, thanks to a favourable potential difference with the cathode and there they assist oxygen reduction reaction. Oxygen comes from the air, hence the Zn–air functionality. The overall theoretical voltage produced by the battery is 1.65 V but because of losses it is usually 0.3–0.4 V lower. The chemical reactions involved are the following:17 | Zn anode Zn + 4OH− → Zn(OH)42− + 2e− (E0 = −1.25 V) | (1) |
| Zn(OH)42− ↔ ZnO + H2O + 2OH− | (2) |
| Gas-diffusion cathode O2 + 4e− + 2H2O → 4OH− (E0 = +0.40 V) | (3) |
| Overall reaction 2Zn + O2 → 2ZnO (ΔV = 1.65 V) | (4) |
All these reactions as well as the corresponding potentials are written for an alkaline electrolyte, which is the most common case.
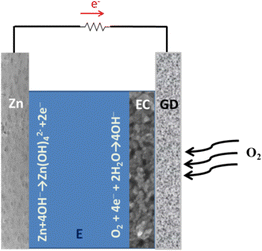 |
| Fig. 1 Schematic illustration of a simple Zn–air battery functioning in the discharging mode: Zn is the zinc foil anode, GD the gas-diffusion electrode (cathode), EC the electrocatalyst deposited on the GD electrode and E the electrolyte. | |
Fig. 1 shows a cell with only one compartment but it is possible to separate the anode from the cathode compartment with an ion-transfer membrane. Reaction (3) is a 4-electron oxygen reduction reaction (ORR) which can take place in the presence of a powerful electrocatalyst. In the lack of such an electrocatalyst, another ORR might take place leading to the production of hydrogen peroxide:
| O2 + 2e− + H2O → H2O2 + 2OH− (E0 = −0.15 V) | (5) |
Even though, hydrogen peroxide hardly survives in an alkaline electrolyte, it may be harmful to the battery and will produce a lower overall voltage (Δ
V = 1.10 V); therefore, a good electrocatalyst is necessary for better battery performance.
Charging of the Zn–air battery corresponds to the reversal of reactions (1)–(3):4
| Zn electrode Zn(OH)42− + 2e− → Zn + 4OH− (Zn2+ → Zn) | (6) |
| Counter electrode 4OH− → O2 + 4e− + 2H2O | (7) |
Charging then of the Zn–air battery means reproduction of Zn at the zinc electrode and oxygen evolution at the gas-diffusion electrode. The minimum electric bias necessary to achieve these reactions is 1.65 V. In reality, it is higher than 2 V
15,16 due to losses and mainly due to the overpotential necessary to achieve the energetically difficult 4-electron oxygen evolution reaction (OER). Various approaches have been adopted in order to decrease this large electric bias.
15–34 They will be reviewed here below.
Bifunctional and photo-assisted electrocatalysts for ORR and OER
In the most common case of a rechargeable Zn–air battery, the cathode electrode is the host of the oxygen reduction reaction in the discharging mode and the oxygen evolution reaction in the charging mode. A straight forward approach, in order to facilitate both charging and discharging functionalities, is to employ an electrode loaded with a bifunctional electrocatalyst capable of carrying out both ORR and OER. There have indeed been published several such cases. Benchmark electrocatalysts for both reactions are, of course, noble metals such as Pt or Ru/Ir oxides.35 However, these electrocatalysts are rare, therefore, expensive and do suffer of some serious problems such as agglomeration or contamination. For this reason, researchers have made a huge effort for developing noble metal substitutes. Electrocatalysts for OER and ORR are of course applicable not only to metal–air batteries but also to a broad range of other devices, notably fuel cells and electrolysers. Bifunctional OER and ORR electrocatalysts may be divided into two major categories: metal free and transition metal-based electrocatalysts.35 However, no clear distinction can be made between the two categories since a large number of works simply refer to electrocatalysts which are made of materials chosen from both categories.
Metal-free electrocatalysts are those based on various carbon nanostructures: graphene, carbon nanotubes, biochar-derived activated carbon or simple graphite nanoparticles. Even though, graphene and carbon nanotubes remain always popular,36–38 in recent years, there is a strong interest expressed towards carbon electrocatalysts obtained by pyrolysis of carbon-rich substrates including biomass-derived wastes.39–48 The three principal properties of any electrocatalyst are electric conductivity, high active surface and presence of active sites which facilitate electron transfer from the solid to the liquid phase. Carbon based materials are conductive, except of course in rare crystalline forms like diamond, and when appropriately treated they are porous and have high active surface. The third property may be obtained by doping. Usual dopants of metal-free electrocatalysts are N, P, F, B and S. For this reason, most recent works focus on dopping porous carbon.36–41,43,45,48 These metal free bifunctional electrocatalysts sometimes outperformed noble metal electrocatalysts and provided long time stability under recyclyning of Zn–air batteries and a small ΔV gap between charging and discharging.39 Biochars obtained by pyrolysis of biomass and further treated with KOH at high temperature obtain a hierarchical porous structure of very high active surface.49 Such materials have so far received small attention as OER/ORR electrocatalysts40,42,50,51 but it is expected that they will become much more popular in the near future. Their hierarchical micro–meso–macro-porous structure (cf.Fig. 2) creates a balance between the need for high active surface and the ability of electrolyte diffusion through the pores and this offers advantage to these materials which may prove effective in their role as electrocatalysts.
 |
| Fig. 2 A typical SEM image of biochar. | |
Transition metal-based materials are the most studied OER/ORR electrocatalysts.35,52 In reality, transition metal-based electrocatalysts always come in combination with carbon, therefore, this case actually concerns hybrid electrocatalysts.6,53–63 Hybrid materials exploit the high active area of porous carbon and the enrichment with active sites offered by the presence of metals. Metals come in as metal particles,56,61,62 alloys,55,57,59 oxides60 or two-metal oxides, like perovskites54,58 or spinels.53,54,63 Spinel oxides are of particular interest for the following reason. Spinels are oxides of two metals with a homonymous crystalline structure, for example, ACo2O4, where A = Mn, Co, Ni, Cu, Zn.63 Substitution of A, in terms of element and percentage, leads to a regulation of the Co3+/Co2+ ratio, therefore to optimization of the OER/ORR capacity of the electrocatalyst.63 The same approach is also adopted in the case of perovskites, which are also oxides of two metals, for example LaMnO3,58 with a different crystalline form from that of the spinels. Again, the combination of two metals allows the regulation of the redox state of the active component in each case resulting in electrocatalyst optimization. There are works that report the employment of such electrocatalysts in Zn–air batteries that have outperformed noble metal electrocatalysts, again providing cycling stability and a small ΔV gap between charging and discharging.54 Similar approach is also adopted in the case of metal alloys, for example NiFe alloys.57 The combination of the two metals also allows regulation of their redox state. Metal alloys are more vulnerable to agglomeration and corrosion. For this reason, they are combined with N-doped carbon. The presence of N provides conditions for metal stabilization.
There is a class of bifunctional electrocatalysts which are photoactive.15,22,23,25,26,29,31,32,34 One such case, is spinel-type Co3O4 which has a direct and indirect band gap in the range 2.2 to 1.35 eV,15 therefore, it absorbs visible light. Co3O4 was employed as electrocatalyst in the air (cathode) electrode of Zn–air batteries and it was observed that the current produced under illumination was 30% larger than in the dark.15 Similar behaviour was also observed with another spinel-type photo-electrocatalyst,29 NiCo2S4, as well with a combination of Ni12P5 with nitrogen-doped carbon nanotubes.22 In general, photoactive materials combined with the air electrode do result in improving the redox properties of a bifunctional electrocatalyst. However, an organic polymer semiconductor, polytritheophene, affected only ORR and had small effect on OER.25 Ref. 6,10 are review papers with a detailed analysis on bifunctional electrocatalysts.
The above discussion shows that an efficient bifunctional electrocatalyst deposited on the air electrode of a Zn–air battery, can facilitate both ORR during battery discharging and the OER during battery charging. A benchmark of electrocatalyst bifunctionality is the small potential difference between galvanostatic charging and discharging and its stability through several charge–discharge cycles,54 as already said. Nevertheless, there is an additional and different approach in dealing with Zn–air battery charging. So far the focus was placed on the electrocatalytic efficiency of the air-electrode. It is also possible to separate the two functions, i.e. the ORR from the OER, in different electrodes and substitute the energetically difficult OER by other processes, as will be detailed in the following section.
Solar charging of Zn–air batteries
Spatial separation of the charging from the discharging process opens the possibility for solar charging of the Zn–air battery.16–20,33Fig. 3 shows a set up for such a case. This time, the cell consists of three electrodes and two compartments separated by an ion-transfer membrane. On the left is a transparent photoelectrode carrying a mesoporous photocatalyst. On the right is an air electrode which carries an ORR electrocatalyst. Zn electrode is in the middle. The two compartments can be filled with the same or different electrolytes. When functioning in the discharging mode, the cell operates as a regular Zn–air battery according to reactions (1)–(4). In the charging mode, the Zn electrode becomes the cathode, receiving electrons and reversing reaction (1), according to reaction (6). Metallic Zn is then deposited on the Zn electrode. These reducing electrons come from the photoanode which produces photogenerated charges by absorption of photons. Photons are absorbed by the photocatalyst generating electrons and holes. Holes oxidize water or, preferably, a sacrificial agent added in the electrolyte of the photoelectrode compartment. Therefore, electron–hole recombination is prevented and electrons are free to move through an external circuit and reach the Zn cathode and produce metallic Zn according to reaction (6). This corresponds to battery charging. The energy for charging comes directly from light; therefore, it may come from sun, hence solar charging. The advantage of this photoelectrocatalytic charging is that OER is in this case substituted by photocatalytic degradation of a sacrificial agent, which is much easier than the OER.16 Such a solar charging model of Zn–air batteries has been applied in ref. 16–20. As a photocatalyst has been used mesoporous titania sensitized in the visible with CdS and CdSe photosensitizers. The bias necessary for solar charging is very small and in certain cases is zero.18 This means that a Zn–air battery can be charged simply by exposing it to solar radiation.18 The sacrificial agent can be any waste of biomass16 or industrial origin.18 This introduces an additional environmental benefit which may be exploited by the above process.
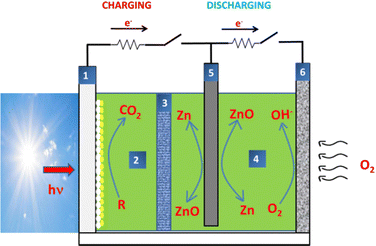 |
| Fig. 3 Schematic illustration of a Zn–air battery with two compartments, three electrodes and separate discharging and solar charging functions: (1) photoelectrode carrying photocatalyst; (2) electrolyte of the photoelectrode compartment; (3) separation membrane; (4) electrolyte of the Zn compartment (5) Zn electrode and (6) air-electrode. | |
Even in the absence of any sacrificial agent, photoelectrocatalytic charging is still more efficient as far as the OER23 is concerned, for the following reason. In the absence of a sacrificial agent, photogenerated holes oxidize water and produce O2, i.e. OER is obtained photocatalytically.23 The valence band potential, which is approximately the potential of the photogenerated holes, is usually much more positive that the OH−/O2 potential (reaction (3)), i.e. 0.40 V in an alkaline electrolyte. Indeed, the valence band of a few characteristic semiconductor photocatalysts in alkaline environment is approximately located at: titania (+2.2 V); CdS (+1.0 V); BiVO4 (+1.5 V), etc. Therefore, they are positive enough to facilitate photocatalytic water oxidation and OER.12,14,23,33 Thus the photocatalytic process eliminates the problem arising with the overpotential for OER.
The driving force which moves photogenerated electrons towards the metal cathode must overcome the opposite bias generated by the presence of the electrolyte. In most cases, both the electrolyte in the photoelectrode compartment and the electrolyte in the Zn compartment are alkaline electrolytes. Therefore, the potential of reaction (1), and (6) is −1.25 V. The conduction band, for example, of photosensitized titania is located around −1.0 V in an alkaline electrolyte. In order then to overcome the opposing bias it is necessary to apply an external bias of at least 0.25 V, in reality, a bit larger. However, when aliphatic alcohols (and other fuels) are used as sacrificial agents, because of a phenomenon called “current doubling”, which is due to injection of electrons by the oxidized fuel into the conduction band of titania, its electronegativity is increased.64 This limits the potential difference and makes the need for external bias much smaller. Thus in Ref. 16 the bias tended to zero for small charging currents and never overpassed 0.5 V for larger currents.
The band structure of other photocatalysts may not be as favourably located and the necessary external bias is then much larger.23 It is concluded, that with the above photoelectrocatalytic system, it is possible to solar-charge a Zn–air battery with minimal energy expenditure.
The disadvantage with the cell of Fig. 3, if any, is the need for employing 3 electrodes. If instead of three, two electrodes are used,23 as in Fig. 4, solar charging is possible with a small external bias but discharging in the same device would necessitate for the photoelectrode to act as an air electrode. Indeed, during charging, the photoanode produces electrons which carry reduction reactions (6) on the metal cathode. During discharging, Zn becomes the anode while the photoelectrode becomes the cathode, i.e. the air electrode. This is not at all efficient with a simple photoelectrode. This matter has been dealt with several studies.2,12,13,23,32,33,65–71 Solar charging with the cell of Fig. 4 is in many ways associated with photo-assisted promotion of OER/ORR electrocatalysis discussed in the previous section.15,22,23,25,26,29,31,32,34 OER is presently substituted by photocatalytic oxidation of water or, preferably, an added sacrificial agent. The main issue remains ORR on the photoelectrode. This problem can be partially solved by adding a ORR co-catalyst.19 However, it remains an open question calling for further research.
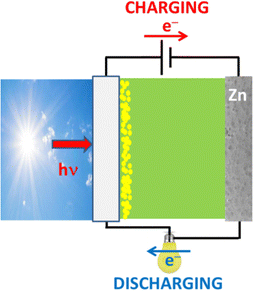 |
| Fig. 4 Schematic illustration of discharging and solar-assisted charging of a Zn–air battery made of a photoelectrode (left) and a Zn electrode (right). | |
In most cases related with Zn–air batteries, no issues arise with electrolyte, since the employed electrolyte is more or less a standard alkaline electrolyte. This is not the case with other type of batteries, for example, potassium metal batteries72 or the redox batteries described in the next section.73–76 Nevertheless, the composition of the electrolyte may play a role concerning Zn dendrite formation. Solar charging as well as any electric charging process faces a common problem of dendrite growth during metal Zn deposition.7,21,24,27,77–83 Dendrite growth limits battery life and for this reason measures must be undertaken, such as use of mechanical obstacles, choice of the electrolyte, additives in the electrolyte, additives on the Zn surface etc., in order to prevent dendrite growth. Table 1 gives a collection of recent publications and lists a series of methods which have been undertaken in order to prevent dendrite growth. Nevertheless, the low tension applied between the photoelectrode and the Zn electrode during solar charging may limit dendrite growth. This is a matter of investigation. An example of Zn deposition images following solar charging can be seen in Ref. 16.
Table 1 List of recently applied methods to prevent dendrite growth
Method |
Ref. |
porous nano-CaCO3 coating |
21
|
ZnO/graphene oxide coating |
24
|
Synthesis of Zn-sponge electrodes |
27
|
Preparation of a Zn–Sn alloy |
77
|
Application of a magnetic field |
78
|
Use of quasi-solid state CO2-decorated highly conductive cellulose electrolyte |
79
|
LDH-Array@PVA Gel Electrolyte and MXene/Zn Anode |
80
|
Deposition of Zn on brass |
81
|
Sn hollow microspheres on Zn and gel polymer electrolyte |
82
|
Sn–Cu/reduced graphene oxide coating |
83
|
Solar batteries
The term solar battery refers to a different configuration and operation principle from that of the above described solar charging or photo-assisted electrocatalysis. A solar battery may be schematically represented by Fig. 5. It is made of two compartments separated by an ion-transfer membrane.69 The compartment on the left comprises two electrodes, a photoelectrode and a counter electrode, in contact with an electrolyte containing a redox couple Cox/Cred (catholyte). The compartment on the right comprises one electrode, the anode, in contact with an electrolyte containing a redox couple Aox/Ared (anolyte). Preferably, the redox potential of C is more positive than that of A. The photoelectrode can be a transparent electrode bearing a semiconductor photocatalyst. Upon absorption of photons, electron–hole pairs are generated. Holes are consumed by oxidizing Cred → Cox and thus lowering the potential to more positive levels. Electrons move to the anode through an external circuit and reduce Aox → Ared raising the potential to more negative levels. This operation corresponds to photo-assisted charging of the battery. In the discharging mode, electrons move from the anode to the counter electrode. The bias for this current flow is provided by the difference in the redox potential between the two electrolytes. Variants of the solar battery of Fig. 5 may involve a cathode electrode on the left side and a photoelectrode with a counter electrode on the right side or involve a tandem configuration with photoelectrodes on both sides.69 It is also possible to drop the counter electrode and use only a photoelectrode combined with an anode (or cathode) electrode.8 Most recently, the solar battery structure is further simplified by very effective 2-electrode configurations, such as the so-called photoelectrochemical redox battery of Ref. 73. The main difference with the devices of Fig. 3 and 4 is the fact that a solar battery contains redox species which reversibly store or release electrochemical energy by regressing between different redox levels. In the case of Zn–air batteries, for example, oxygen is consumed irreversibly during discharging while charging may not necessarily reproduce the consumed oxygen. Theoretically, the two cases are similar but in practice may be very different.
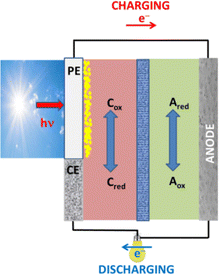 |
| Fig. 5 Schematic Illustration of a solar battery: (PE) photoelectrode; (CE) counter electrode. C (Catholyte) and A (anolyte) represent redox couples of different redox potential. | |
The first solar batteries were developed in the seventies using CdSe and Ag2S/Ag as photoelectrode and anode electrode respectively, together with a polysulfide electrolyte.74 The redox potential of the polysulfide electrolyte is −0.69 V while that of Ag2S/Ag is −0.45 V, therefore, their combination is thermodynamically favorable.69 Since then, this field has enormously evolved and has produced a large number of publications which cannot be efficiently managed by this brief review. Nevertheless, it is worth mentioning some recent studies on solar batteries, not necessarily involving Zn. One such remarkable case is an all-vanadium photoelectrochemical flow cell, which combines a vanadium redox flow battery with a photoelectrochemical flow cell75 as well as the above mentioned photoelectrochemical redox battery.73 Utilization of all-vanadium redox couples76 certainly simplifies solar battery construction possibilities.
Conclusions and perspectives
The present brief review has shown that it is possible to use solar energy to charge a Zn–air battery by employing a photoelctrochemical cell integrating both the solar conversion and energy storage components.
The main challenge in this type of integrated devices is the need for an external bias to overcome part of the opposing bias created by zinc electrode. An obvious suggestion is to employ a tandem electrode structure which may eliminate the need for an external bias, even though, this will necessitate additional components.
It is expected that research on bifunctional electrocatalysts will continue to lead the pace, as it is shown in recent publications.84–87
The problem of Zn dendrite formation during solar charging needs to be further elaborated since the related conditions present several particularities. Zn dendrite formation during battery charging depends on the applied voltage and the ensuing current. In the case of solar charging, the applied voltages are relatively small; therefore, dendrite formation in those cases needs specific elaboration.
Finally, solar charging of Zn–air batteries is so far distinguished from solar batteries which are based on photoelectrochemical redox functionalities.
Conflicts of interest
There are no conflicts to declare.
Notes and references
- P. Li, C. Li, X. Guo, X. Li and C. Zhi, Metal-Iodine and Metal-Bromine Batteries: A Review, Bull. Chem. Soc. Jpn., 2021, 94, 2036–2042 CrossRef CAS.
-
J. Yi and Y. Xia, Advanced aqueous batteries: Status and challenges, MRS Energy & Sustainability, 2022, Vol. 9, pp. 106–128 Search PubMed.
- Y. Li and H. Dai, Recent advances in zinc–air batteries, Chem. Soc. Rev., 2014, 43, 5257–5275 RSC.
- P. Gu, M. Zheng, Q. Zhao, X. Xiao, H. Xue and H. Pang, Rechargeable zinc–air batteries: a promising way to green energy, J. Mater. Chem. A, 2017, 5, 7651–7666 RSC.
- A. R. Mainar, E. Iruin, L. C. Colmenares, A. Kvasha, I. de Meatza, M. Bengoechea, O. Leonet, I. Boyano, Z. Zhang and J. A. Blazquez, An overview of progress in electrolytes for secondary zinc-air batteries and other storage systems based on zinc, J. Energy Storage, 2018, 15, 304–3028 CrossRef.
- J. Fu, R. Liang, G. Liu, A. Yu, Z. Bai, L. Yang and Z. Chen, Recent Progress in Electrically Rechargeable Zinc–Air Batteries, Adv. Mater., 2019, 31, 1805230 CrossRef PubMed.
- Z. Zhao, X. Fan, J. Ding, W. Hu, C. Zhong and J. Lu, Challenges in Zinc Electrodes for Alkaline Zinc–Air Batteries: Obstacles to Commercialization, ACS Energy Lett., 2019, 4, 2259–2270 CrossRef CAS.
- Z. Fang, X. Hu and D. Yu, Integrated Photo-Responsive Batteries for Solar Energy Harnessing: Recent Advances, Challenges, and Opportunities, ChemPlusChem, 2020, 85, 600–612 CrossRef CAS PubMed.
- M. Kaya, S. Hussaini and S. Kursunoglu, Critical review on secondary zinc resources and their recycling technologies, Hydrometallurgy, 2020, 195, 105362 CrossRef CAS.
- L. Wei, E. H. Ang, Y. Yang, Y. Qin, Y. Zhang, M. Ye, Q. Liu and C. C. Li, Recent advances of transition metal based bifunctional electrocatalysts for rechargeable zinc-air batteries, J. Power Sources, 2020, 477, 228696 CrossRef CAS.
- Y. Yu and S. Hu, The applications of semiconductor materials in air batteries, Chin. Chem. Lett., 2021, 32, 3277–3287 CrossRef CAS.
- O. Ola, N. Wang, G. Walker, Y. Zhu and D. Grant, Engineering the next generation of photorechargeable zinc-air batteries, Curr. Opin. Electrochem., 2022, 35, 101040 CrossRef CAS.
- C. Rodríguez-Seco, Y.-S. Wang, K. Zaghib and D. Ma, Photoactive nanomaterials enabled integrated photo-rechargeable batteries, Nanophotonics, 2022, 11, 1443–1484 CrossRef.
- N. Yan and X. Gao, Photo-assisted Rechargeable Metal Batteries for Energy Conversion and Storage, Energy Environ. Mater., 2022, 5, 439–451 CrossRef CAS.
- C. Tomon, S. Sarawutanukul, S. Duangdangchote, A. Krittayavathananon and M. Sawangphruk, Photoactive Zn–air batteries using spinel-type cobalt oxide as a bifunctional photocatalyst at the air cathode, Chem. Commun., 2019, 55, 5855–5858 RSC.
- M. Katsaiti, E. Papadogiannis, V. Dracopoulos, A. Keramidas and P. Lianos, Solar charging of a Zn-air battery, J. Power Sources, 2023, 555, 232384 CrossRef CAS.
- T. Santos Andrade, M. Cesar Pereira and P. Lianos, High voltage gain in photo-assisted charging of a metal-air battery, J. Electroanal. Chem., 2020, 878, 114559 CrossRef.
- T. Santos Andrade, V. Dracopoulos, M. César Pereira and P. Lianos, Unmediated photoelectrochemical charging of a Zn-air battery: The realization of the photoelectrochemical battery, J. Electroanal. Chem., 2020, 878, 114709 CrossRef.
- T. Santos Andrade, I. Campos Sena, L. C. Alves de Oliveira, P. Lianos and M. Cesar Pereira, Decreasing the charging voltage of a zinc-air battery using a bifunctional W:BiVO4/V2O5 photoelectrode and sulfite as a sacrificial agent, Mater. Today Commun., 2021, 28, 102546 CrossRef.
- T. Santos Andrade, A. R. S.Neto, F. G. E. Nogueira, L. C. Alves de Oliveira, M. Cesar Pereira and P. Lianos, Photo-Charging a Zinc-Air Battery Using a Nb2O5-CdS Photoelectrode, Catalysts, 2022, 12, 1240 CrossRef.
- L. Kang, M. Cui, F. Jiang, Y. Gao, H. Luo, J. Liu, W. Liang and C. Zhi, Nanoporous CaCO3 Coatings Enabled Uniform Zn Stripping/Plating for Long-Life Zinc Rechargeable Aqueous Batteries, Adv. Energy Mater., 2018, 8, 1801090 CrossRef.
- J. Lv, S. C. Abbas, Y. Huang, Q. Liu, M. Wu, Y. Wang and L. Dai, A photo-responsive bifunctional electrocatalyst for oxygen reduction and evolution reactions, Nano Energy, 2018, 43, 130–137 CrossRef CAS.
- X. Liu, Y. Yuan, J. Liu, B. Liu, X. Chen, J. Ding, X. Han, Y. Deng, C. Zhong and W. Hu, Utilizing solar energy to improve the oxygen evolution reaction kinetics in zinc–air battery, Nat. Commun., 2019, 10, 4767 CrossRef PubMed.
- Z. Zhou, Y. Zhang, P. Chen, Y. Wu, H. Yang, H. Ding, Y. Zhang, Z. Wang, X. Du and N. Liu, Graphene oxide-modified zinc anode for rechargeable aqueous batteries, Chem. Eng. Sci., 2019, 194, 142–147 CrossRef CAS.
- D. Zhu, Q. Zhao, G. Fan, S. Zhao, L. Wang, F. Li and J. Chen, Photoinduced Oxygen Reduction Reaction Boosts the Output Voltage of a Zinc–Air Battery, Angew. Chem., 2019, 131, 12590–12594 CrossRef.
- D. Du, S. Zhao, Z. Zhu, F. Li and J. Chen, Photo-excited Oxygen Reduction and Oxygen Evolution Reactions Enable a High-Performance Zn–Air Battery, Angew. Chem., Int. Ed., 2020, 59, 18140–18144 CrossRef CAS PubMed.
- B. J. Hopkins, C. N. Chervin, M. B. Sassin, J. W. Long, D. R. Rolison and J. F. Parker, Low-cost green synthesis of zinc sponge for rechargeable, sustainable batteries, Sustainable Energy Fuels, 2020, 4, 3363–3369 RSC.
- A. Mathur, R. Kaushik and A. Halder, Visible-light-driven photo-enhanced zinc–air batteries using synergistic effect of different types of MnO2 nanostructures, Catal. Sci. Technol., 2020, 10, 7352–7364 RSC.
-
S. Sarawutanukul, C. Tomon, S. Duangdangchote, N. Phattharasupakun and M. Sawangphruk, Rechargeable Photoactive Zn-Air Batteries Using NiCo2S4 as an Efficient Bifunctional Photocatalyst towards OER/ORR at the Cathode.
- A. Mathur, R. Kaushik and A. Halder, Photoenhanced performance of Cobalt-intercalated 2-D manganese oxide sheets for rechargeable zinc-air batteries, Mater. Today Energy, 2021, 19, 100612 CrossRef CAS.
- D. Bu, M. Batmunkh, Y. Zhang, Y. Li, B. Qian, Y. Lan, X. Hou, S. Li, B. Jia, X.-M. Song and T. Ma, Rechargeable sunlight-promoted Zn-air battery constructed by bifunctional oxygen photoelectrodes: Energy-band switching between ZnO/Cu2O and ZnO/CuO in charge-discharge cycles, Chem. Eng. J., 2022, 433, 133559 CrossRef CAS.
- H. Feng, C. Zhang, Z. Liu, J. Sang, S. Xue and P. K. Chu, A light-activated TiO2@In2Se3@Ag3PO4 cathode for high-performance Zn-Air batteries, Chem. Eng. J., 2022, 434, 134650 CrossRef CAS.
- L. Kong, J. Qiao, Q. Ruan, H. Wang, X. Xi, W. Zha, Z. Zhou, W. He, W. Zhang and Z. M. Sun, A very low charge potential for zinc-air battery promoted by photochemical effect of triazine-based conjugated polymer nanolayer coated TiO2, J. Power Sources, 2022, 536, 231507 CrossRef CAS.
- R. Ren, G. Liu, J. Y. Kim, R. E. A. Ardhi, M. X. Tran, W. Yang and J. K. Lee, Photoactive g-C3N4/CuZIF-67 bifunctional electrocatalyst with staggered p-n heterojunction for rechargeable Zn-air batteries, Appl. Catal., B, 2022, 306, 121096 CrossRef CAS.
- W. Fang, J. Zhao, W. Zhang, P. Chen, Z. Bai and M. Wu, Recent progress and future perspectives of flexible Zn-Air batteries, J. Alloys Compd., 2021, 869, 158918 CrossRef CAS.
- X. Wang, G.-L. Li, Z.-F. Lu, S. Cao, C. Hao, S. Wang and G. Sun, In situ coating of a N, S co-doped porous carbon thin film on carbon nanotubes as an advanced metal-free bifunctional oxygen electrocatalyst for Zn–air batteries, Catal. Sci. Technol., 2022, 12, 181–191 RSC.
- X. Zhang, X. Wen, C. Pan, X. Xiang, C. Hao, Q. Meng, Z. Q. Tian, P. K. Shen and S. P. Jiang, N species tuning strategy in N, S co-doped graphene nanosheets for electrocatalytic activity and selectivity of oxygen redox reactions, Chem. Eng. J., 2022, 431, 133216 CrossRef CAS.
- J. Zhang and L. Dai, Nitrogen, Phosphorus, and Fluorine Tri-doped Graphene as a Multifunctional Catalyst for Self-Powered Electrochemical Water Splitting, Angew. Chem., Int. Ed., 2016, 55, 13296–13300 CrossRef CAS PubMed.
- X. Xiao, X. Li, Z. Wang, G. Yan, H. Guo, Q. Hu, L. Li, Y. Liu and J. Wang, Robust template-activator cooperated pyrolysis enabling hierarchically porous honeycombed defective carbon as highly-efficient metal-free bifunctional electrocatalyst for Zn-air batteries, Appl. Catal., B, 2020, 265, 118603 CrossRef CAS.
- M. Tan, Q. Wang, S. Wang, W. Liu, D. Wang, S. Luo, P. Hou, M. Zhou, Y. Zhang, S. Yan and X. Liu, Ternary (N, B, F)-Doped Biocarbon Derived from Bean Residues as Efficient Bifunctional Electrocatalysts for Oxygen Reduction and Evolution Reactions, J. Electrochem. Soc., 2022, 169, 096517 CrossRef.
- F. Gui, Q. Jin, D. Xiao, X. Xu, Q. Tan, D. Yang, B. Li, P. Ming, C. Zhang, Z. Chen, S. Siahrostami and Q. Xiao, High-Performance Zinc-Air Batteries Based on Bifunctional Hierarchically Porous Nitrogen-Doped Carbon, Small, 2022, 18, 2105928 CrossRef CAS PubMed.
- M. A. A. Mahbub, A. Mulyadewi, C. G. Adios and A. Sumboja, Sustainable Chicken Manure-derived Carbon as a Metal free Bifunctional Electrocatalyst in Zn-air Battery, AIP Conf. Proc., 2022, 2652, 040011 CrossRef.
- Y. Wang, R. Gan, S. Zhao, W. Ma, X. Zhang, Y. Song, C. Ma and J. Shi, B, N, F tri-doped lignin-derived carbon nanofibers as an efficient metal-free bifunctional electrocatalyst for ORR and OER in rechargeable liquid/solid-state Zn-air batteries, Appl. Surf. Sci., 2022, 598, 153891 CrossRef CAS.
- J. Zhang, Z. Zhao, Z. Xia and L. Dai, A metal-free bifunctional electrocatalyst for oxygen reduction and oxygen evolution reactions, Nat. Nanotechnol., 2015, 10, 444–452 CrossRef CAS PubMed.
- J. Zhang, L. Qu, G. Shi, J. Liu, J. Chen and L. Dai, N,P-Codoped Carbon Networks as Efficient Metal-free Bifunctional Catalysts for Oxygen Reduction and Hydrogen Evolution Reactions, Angew. Chem., Int. Ed., 2016, 55, 2230–2234 CrossRef CAS PubMed.
- S. Zhao, D.-W. Wang, R. Amal and L. Dai, Carbon-Based Metal-Free Catalysts for Key Reactions Involved in Energy Conversion and Storage, Adv. Mater., 2019, 31, 1801526 CrossRef PubMed.
- S. S. Shinde, C.-H. Lee, A. Sami, D.-H. Kim, S.-U. Lee and J.-H. Lee, Scalable 3-D Carbon Nitride Sponge as an Efficient Metal-Free Bifunctional Oxygen Electrocatalyst for Rechargeable Zn-Air Batteries, ACS Nano, 2017, 11(1), 347–357 CrossRef CAS PubMed.
- L. Zong, W. Wu, S. Liu, H. Yin, Y. Chen, C. Liu, K. Fan, X. Zhao, X. Chen, F. Wang, Y. Yang, L. Wang and S. Feng, Metal-free, active nitrogen-enriched, efficient bifunctional oxygen electrocatalyst for ultrastable zinc-air batteries, Energy Storage Mater., 2020, 27, 514–521 CrossRef.
- S. Giannakopoulos, J. Vakros, V. Dracopoulos, I. D. Manariotis, D. Mantzavinos and P. Lianos, Enhancement of the photoelectrocatalytic degradation rate of a pollutant in the presence of a supercapacitor, J. Cleaner Prod., 2022, 377, 134456 CrossRef CAS.
- Y. Li, Y. Qu, C. Liu, J. Cui, K. Xu, Y. Li, H. Shen, Z. Lu, H. Pan, T. Xu and D. Liu, Processing Agricultural Cornstalks toward High-Efficient Stable Bifunctional ORR/OER Electrocatalysts, Adv. Sustainable Syst., 2022, 6, 2100343 CrossRef CAS.
- L.-L. Ma, X. Hu, W.-J. Liu, H.-C. Li, P. K. S. Lam, R. J. Zeng and H.-Q. Yu, Constructing N, P-dually doped biochar materials from biomass wastes for high-performance bifunctional oxygen electrocatalysts, Chemosphere, 2021, 278, 130508 CrossRef CAS PubMed.
- A. Kundu, S. Mallick, S. Ghora and C. Retna Raj, Advanced Oxygen Electrocatalyst for Air-Breathing Electrode in Zn-Air Batteries, ACS Appl. Mater. Interfaces, 2021, 13, 40172–40199 CrossRef CAS PubMed.
- M. Faraji, S. Yousefzadeh, M. F. Nassar and M. M. Abdul Zahra, MnCo2O4/N-doped graphene quantum dot vigorously coupled to MXene nanosheet: A bifunctional Oxygen electrocatalyst outperforms Pt/IrO2 benchmark electrocatalysts in metal-air batteries, J. Alloys Compd., 2022, 927, 167115 CrossRef CAS.
- B. He, Y. Deng, H. Wang, R. Wang, J. Jin, Y. Gong and L. Zhao, Metal organic framework derived perovskite/spinel heterojunction as efficient bifunctional oxygen electrocatalyst for rechargeable and flexible Zn-air batteries, J. Colloid Interface Sci., 2022, 625, 502–511 CrossRef CAS PubMed.
- H. Lei, L. Ma, Q. Wan, Z. Huangfu, S. Tan, Z. Wang and W. Mai, Porous carbon nanofibers confined NiFe alloy nanoparticles as efficient bifunctional electrocatalysts for Zn-air batteries, Nano Energy, 2022, 104, 107941 CrossRef CAS.
- W. Li, F. Wang, Z. Zhang and S. Min, Graphitic carbon layer-encapsulated Co nanoparticles embedded on porous carbonized wood as a self-supported chainmail oxygen electrode for rechargeable Zn-air batteries, Appl. Catal., B, 2022, 317, 121758 CrossRef CAS.
- S.-Y. Lin, X. Zhang, S.-Y. Sang, L. Zhang, J.-J. Feng and A.-J. Wang, Bio-derived FeNi alloy confined in N-doped carbon nanosheets as efficient air electrodes for Zn–air battery, J. Colloid Interface Sci., 2022, 628, 499–507 CrossRef CAS PubMed.
- M. García-Rodríguez, J. X. Flores-Lasluisa, D. Cazorla-Amorós and E. Morallón, Metal oxide Perovskite-Carbon composites as electrocatalysts for zinc-air batteries. Optimization of ball-milling mixing parameters, J. Colloid Interface Sci., 2023, 630, 269–280 CrossRef PubMed.
- X. Liang, H. Xiao, T. Zhang, F. Zhang and Q. Gao, A unique nanocomposite with FeCo nanoalloy anchored on S, N co-doped carbonaceous matrix for high bifunctional oxygen reduction reaction/oxygen evolution reaction electrocatalytic property in Zn-air battery, J. Colloid Interface Sci., 2023, 630, 170–181 CrossRef CAS PubMed.
- X. Wu, Z. Zhang, C. He, Y. Shen, X. Wu, H. Wang, Z. Ma and Q. Li, Mixed-valence cobalt oxides bifunctional electrocatalyst with rich oxygen vacancies for aqueous metal-air batteries, Chem. Eng. J., 2023, 453, 139831 CrossRef CAS.
- T. Yu, H. Xu, Z. Jin, Y. Zhang and H.-J. Qiu, Noble metal-free high-entropy oxide/Co-N-C bifunctional electrocatalyst enables highly reversible and durable Zn-air batteries, Appl. Surf. Sci., 2023, 610, 155624 CrossRef CAS.
- Z. Li, X. Lin, W. Xi, M. Shen, B. Gao, Y. Chen, Y. Zheng and B. Lin, Bamboo-like N,S-doped carbon nanotubes with encapsulated Co nanoparticles as high-performance electrocatalyst for liquid and flexible all-solid-state rechargeable Zn-air batteries, Appl. Surf. Sci., 2022, 593, 153446 CrossRef CAS.
- Z. Wang, J. Huang, L. Wang, Y. Liu, W. Liu, S. Zhao and Z.-Q. Liu, Cation-Tuning Induced d-Band Center Modulation on Co-Based Spinel Oxide for Oxygen Reduction/Evolution Reaction, Angew. Chem., Int. Ed., 2022, 61, e202114696 CAS.
- E. Kalamaras and P. Lianos, Current Doubling effect revisited: Current multiplication in a PhotoFuelCell, J. Electroanal. Chem., 2015, 751, 37–42 CrossRef CAS.
- A. C. Brooks, K. Basore and S. Bernhard, Photon-Driven Reduction of Zn2+ to Zn Metal, Inorg. Chem., 2013, 52, 5794–5800 CrossRef CAS PubMed.
- Z. Tian, Z. Sun, Y. Shao, L. Gao, R. Huang, Y. Shao, R. B. Kaner and J. Sun, Ultrafast rechargeable Zn micro-batteries endowing a wearable solar charging system with high overall efficiency, Energy Environ. Sci., 2021, 14, 1602 RSC.
- X. Xie, Z. Fang, M. Yang, F. Zhu and D. Yu, Harvesting Air and Light Energy via “All-in-One” Polymer Cathodes for High-Capacity, Self-Chargeable, and Multimode-Switching Zinc Batteries, Adv. Funct. Mater., 2021, 31, 2007942 CrossRef CAS.
- P. Chen, T.-T. Li, Y.-B. Yang, G.-R. Li and X.-P. Gao, Coupling aqueous zinc batteries and perovskite solar cells for simultaneous energy harvest, conversion and storage, Nat. Commun., 2022, 13, 64 CrossRef CAS PubMed.
- M. Yu, W. D. McCulloch, Z. Huang, B. B. Trang, J. Lu, K. Amine and Y. Wu, Solar-powered electrochemical energy storage: an alternative to solar fuels, J. Mater. Chem. A, 2016, 4, 2766–2782 RSC.
- J. Park, K.-Y. Yoon, M.-J. Kwak, J.-E. Lee, J. Kang and J.-H. Jang, Sn-Controlled Co-Doped Hematite for Efficient Solar-Assisted Chargeable Zn–Air Batteries, ACS Appl. Mater. Interfaces, 2021, 13, 54906–54915 CrossRef CAS PubMed.
- X. Wang, Y. Li, M. Yang, C. Liu, J. Li and D. Yu, Unique Step-Scheme Heterojunction Photoelectrodes for Dual-Utilization of Light and Chemical Neutralization Energy in Switchable Dual-Mode Batteries, Adv. Funct. Mater., 2022, 32, 2205518 CrossRef CAS.
- L. Fan, H. Xie, Y. Hu, Z. Caixiang, A. M. Rao, J. Zhou and B. Lu, A tailored electrolyte for safe and durable potassium ion batteries, Energy Environ. Sci., 2023, 16, 305 RSC.
- H. Feng, D. Liu, Y. Zhang, X. Shi, O. C. Esan, Q. Li, R. Chen and L. An, Advances and Challenges in Photoelectrochemical Redox Batteries for Solar Energy Conversion and Storage, Adv. Energy Mater., 2022, 12, 2200469 CrossRef CAS.
- G. Hodes, J. Manassen and D. Cahen, Photoelectrochemical energy conversion and storage using polycrystalline chalcogenide electrodes, Nature, 1976, 261, 403–404 CrossRef CAS.
- J. Li, Y. Lin, R. Chen, X. Zhu, D. Ye, Y. Yang, Y. Yu, D. Wang and Q. Liao, Solar energy storage by a microfluidic all-vanadium photoelectrochemical flow cell with self-doped TiO2 photoanode, J. Energy Storage, 2021, 43, 103228 CrossRef.
- T. Santos Andrade, V. Dracopoulos, A. Keramidas, M. Cesar Pereira and P. Lianos, Charging a vanadium redox battery with a photo(catalytic) fuel cell, Sol. Energy Mater. Sol. Cells, 2021, 221, 110889 CrossRef.
- Y. Peng, C. Lai, M. Zhang, X. Liu, Y. Yin, Y. Li and Z. Wu, Zn–Sn alloy anode with repressible dendrite grown and meliorative corrosion resistance for Zn-air battery, J. Power Sources, 2022, 526, 231173 CrossRef CAS.
- P. Liang, Q. Li, L. Chen, Z. Tang, Z. Li, Y. Wang, Y. Tang, C. Han, Z. Lan, C. Zhi and H. Li, The magnetohydrodynamic effect enables a dendrite-free Zn anode in alkaline electrolytes, J. Mater. Chem. A, 2022, 10, 11971 RSC.
- Q. Liu, X. Ou, L. Li, X. Wang, J. Wen, Y. Zhou and F. Yan, Recyclable and CO2-retardant Zn–air batteries based on CO2-decorated highly conductive cellulose electrolytes, J. Mater. Chem. A, 2022, 10, 12235 RSC.
- X. Hui, P. Zhang, J. Li, D. Zhao, Z. Li, Z. Zhang, C. Wang, R. Wang and L. Yin, In Situ Integrating Highly Ionic Conductive LDH-Array@PVA Gel Electrolyte and MXene/Zn Anode for Dendrite-Free High-Performance Flexible Zn–Air Batteries, Adv. Energy Mater., 2022, 12, 2201393 CrossRef CAS.
- T. Nagy, L. Nagy, Z. Erdélyi, E. Baradács, G. Deák, M. Zsuga and S. Kéki, “In Situ” Formation of Zn Anode from Bimetallic Cu–Zn Alloy (Brass) for Dendrite-Free Operation of Zn-Air Rechargeable Battery, Batteries, 2022, 8, 212 CrossRef.
- H. Li, J. Guo, Y. Mao, G. Wang, J. Liu, Y. Xu, Z. Wu, Z. Mei, W. Li, Y. He and X. Liang, Regulation of Released Alkali from Gel Polymer Electrolyte in Quasi-Solid State Zn–Air Battery, Small, 2023, 19, 2206814 CrossRef CAS PubMed.
- H. Zhao, Z. Chi, Q. Zhang, D. Kong, L. Li, Z. Guo and L. Wang, Dendrite-free Zn anodes enabled by Sn-Cu bimetal/rGO functional protective layer for aqueous Zn-based batteries, Appl. Surf. Sci., 2023, 613, 156129 CrossRef CAS.
- S. Zheng, M. Chen, K. Chen, Y. Wu, J. Yu, T. Jiang and M. Wu, Solar-Light-Responsive Zinc–Air Battery with Self-Regulated Charge–Discharge Performance based on Photothermal Effect, ACS Appl. Mater. Interfaces, 2023, 15, 2985–2995 CrossRef CAS PubMed.
- J. Ge, L. Fan, A. M. Rao, J. Zhou and B. Lu, Surface-substituted Prussian blue analogue cathode for sustainable potassium-ion batteries, Nat. Sustainability, 2022, 5, 225–234 CrossRef.
- K. Yuan, D. Lützenkirchen-Hecht, L. Li, L. Shuai, Y. Li, R. Cao, M. Qiu, X. Zhuang, M. K. H. Leung, Y. Chen and U. Scherf, Boosting Oxygen Reduction of Single Iron Active Sites via Geometric and Electronic Engineering: Nitrogen and Phosphorus Dual Coordination, J. Am. Chem. Soc., 2020, 142, 2404–2412 CrossRef CAS PubMed.
- Y. Wu, X. Tang, F. Zhang, L. Li, W. Zhai, B. Huang, T. Hu, D. Lützenkirchen-Hecht, K. Yuan and Y. Chen, Manipulating the electronic configuration of Fe–N4 sites by an electron-withdrawing/donating strategy with improved oxygen electroreduction performance, Mater. Chem. Front., 2022, 6, 1209 RSC.
|
This journal is © the Owner Societies 2023 |
Click here to see how this site uses Cookies. View our privacy policy here.