DOI:
10.1039/D3CS00475A
(Tutorial Review)
Chem. Soc. Rev., 2023,
52, 6344-6358
Strategies to convert organic fluorophores into red/near-infrared emitting analogues and their utilization in bioimaging probes
Received
12th July 2023
First published on 23rd August 2023
Abstract
Organic fluorophores aided by current microscopy imaging modalities are essential for studying biological systems. Recently, red/near-infrared emitting fluorophores have attracted great research efforts, as they enable bioimaging applications with reduced autofluorescence interference and light scattering, two significant obstacles for deep-tissue imaging, as well as reduced photodamage and photobleaching. Herein, we analyzed the current strategies to convert key organic fluorophores bearing xanthene, coumarin, and naphthalene cores into longer wavelength-emitting derivatives by focussing on their effectiveness and limitations. Together, we introduced typical examples of how such fluorophores can be used to develop molecular probes for biological analytes, along with key sensing features. Finally, we listed several critical issues to be considered in developing new fluorophores.
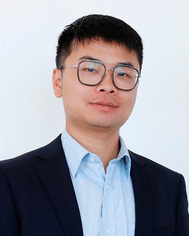
Mingchong Dai
| Dr Mingchong Dai received his BS from the South-Central University for Nationalities in 2012 and his MS from Hunan University in 2015. Later, he joined Prof. Kyo Han Ahn's group at POSTECH and earned his PhD in 2020. He was a postdoctoral scholar at POSTECH until 2022. Currently, he is a postdoctoral scholar at Cancer Early Detection Advanced Research Center, OHSU Knight Cancer Institute, his research is focused on fluorophore chemistry, fluorescence-related technology, molecular probes in early cancer detection, etc. |
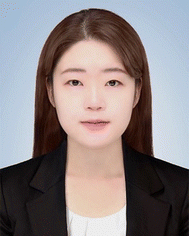
Yun Jae Yang
| Yun Jae Yang was born and raised in the Republic of Korea. She received her BS degree in Chemistry from the Sookmyung Women's University. She is now a 5th year PhD student working with Prof. Kyo Han Ahn at POSTECH, South Korea. Her research interest includes fluorescent organic molecular probes and their bioimaging applications. |
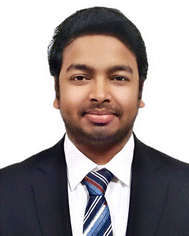
Sourav Sarkar
| Dr Sourav Sarkar received his BS from the University of Calcutta in 2013 and MS from IIT Kharagpur in 2015. Later, he joined Prof. Kyo Han Ahn's group at POSTECH and earned his PhD in 2020. He was appointed as a research assistant professor at POSTECH in 2022. His research is focused on molecular probes, environment-insensitive fluorophores, redox biology, organelle dynamics etc. |
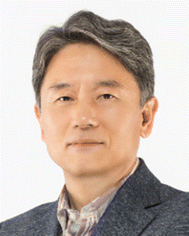
Kyo Han Ahn
| Dr Kyo Han Ahn currently holds the title of Specially Designated/Emeritus Professor at POSTECH. His educational journey includes the attainment of a BS degree from Seoul National University in 1980 and a PhD degree in Organic Chemistry from KAIST in 1985. His research efforts garnered him several distinguished awards, including the Oder of Science and Technological Merit bestowed by the Republic of Korea, along with the Academic Excellence Prize granted by the Korean Chemical Society. He holds the title of Fellow of the Korean Academy of Science and Technology. Professor Ahn's research journey has traversed diverse terrains, initially encompassing organic synthesis and organometallic catalysts, and subsequently expanding into the realms of molecular recognition, luminescent materials, and molecular probes for bioimaging. |
Key learning points
1. Importance of red/NIR emitting fluorophores.
2. Feasibility and challenges to convert conventional fluorophores into their red/NIR emitting analogues.
3. Molecular-level understanding of the correlation between the structural modifications and the bathochromic emission shifts.
4. Bio-relevant applications (utilization in bioimaging probes) of red/NIR emitting fluorophores.
5. Scope and limitations of conventional structural modification methods.
|
1. Introduction
Organic fluorophores are essential for bioimaging applications due to their distinctive characteristics, such as non-invasiveness, high sensitivity and selectivity, and capability for real-time imaging. Such advantages also hold immense promise for clinical applications.1 Fluorophores emitting in the longer wavelengths, preferably in the red/near-infrared (NIR) region, are in great demand for bioimaging of tissues with reduced autofluorescence interference and at deeper imaging depths. The issue of autofluorescence, arising from innate biomolecules, presents a significant concern in tissue imaging at deeper depths. Due to the emission of fluorescent biomolecules, including NAD(P)H, flavins, lipofuscin, etc., mostly in the blue, green, and orange wavelengths,2 utilizing fluorophores that emit in the red (>630 nm) or near-infrared (NIR) region is essential for bioimaging applications (Fig. 1a–d). Failing to do so could lead to substantial autofluorescence interference in tissue imaging.3 The magnitude of autofluorescence varies across different tissue types, exhibiting notable levels in the green and yellow emission channels but diminishing beyond approximately 630 nm (Fig. 1e–g).4 Additionally, the excitation of fluorophores at longer wavelengths leads to reduced photo-bleaching of them and reduced photo-damaging of biological samples. Accordingly, great efforts have been made to develop longer-emitting dyes from typical organic fluorophores, leading to significant progress in this field.5 Hence, it is crucial to prioritize the development of fluorophores with emissions in the red/NIR region. At this point, it is worth noting that currently there is no consensus on using the terminology “NIR” among scientists working in the field of fluorescent probes. A dye is frequently claimed to be a NIR dye when its emission spectra cover some portion above 700 nm or so, even though the maximum is below 700 nm. The “red” wavelength region ranges from 620 to 750 nm and that for the NIR from 700 to 1500 nm (according to the IEC 60050-845:2007 standard), generating an overlapping region between red and NIR, from 700 to 750 nm. It may be acceptable to claim a NIR dye if its emission maximum is above 700 nm.
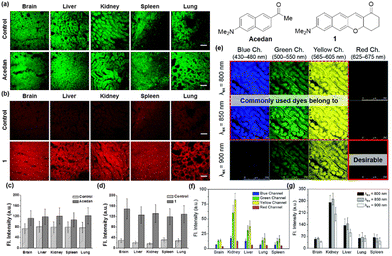 |
| Fig. 1 Fluorescent images of mouse tissues (brain, liver, kidneys, spleen, and lungs from the left) stained with (a) acedan (10 μM) and (b) its π-extended analogue, observed under two-photon excitation at 780 and 900 nm, respectively. (c) and (d) Relative fluorescence intensities of the images of (a) and (b), respectively. (e) Autofluorescence images of mouse kidney tissues, obtained under two-photon excitation at 800, 850, and 900 nm, and emission collection through blue (430–480 nm), green (500–550 nm), yellow (565–605 nm), and red (625–675 nm) channels. (f) Comparison of the total autofluorescence intensities from the four emission channels between different organ tissues (brain, kidneys, liver, lungs, and spleen); (g) comparison of the total autofluorescence intensities between the organ tissues depending on the excitation wavelength. Reproduced permission: (a)–(d) Copyright©2015, American Chemical Society. (e)–(g) Licensed under a Creative Commons Attribution-Non Commercial 3.0 Unported Licence. | |
In this tutorial review, we wish to introduce key strategies that convert several common organic fluorophores into analogues that emit in longer wavelengths, preferably in the red/NIR wavelength region. Commonly used strategies involve π-conjugation extension, modification of the electron-donor and -acceptor groups, and replacement of the central heteroatom in xanthene dyes, which can be best demonstrated with the fluorophores based on xanthene, coumarin, and naphthalene cores. Organic fluorophores having xanthene, coumarin, and naphthalene cores can be readily functionalized and thus have been widely used for biological applications. These fluorophores also provide good starting points for the development of longer-emitting derivatives. The structural modifications lead to variable emission bathochromic shifts, which are also dependent on the molecular geometry. Understanding the “effective” directions for the structural modifications toward the bathochromic emission spectral shift will provide valuable design guidance to new organic fluorophores with desirable features for bioimaging applications. Herein we analyzed the key structural modifications of the fluorophores for the bathochromic shift categorized by the core structures. Besides, we briefly introduced how these fluorophores can find their applications in studying biological issues.
2. Xanthene derivatives
The widely used rhodamine and fluorescein dyes are xanthene (10H-9-oxa-anthracene) derivatives with nitrogen and oxygen substitutions both at C-2 and C-7, respectively, together with the (o-carboxy)phenyl substituent at C-9. Rhodamine and fluorescein analogues simply with a phenyl substituent at C-9 are called rosamine and fluorone dyes, respectively (here denoted as CFPX). A hybrid form between rhodamine and fluorescein is named rhodol, and that between rosamine and fluorone is named rosol (Fig. 2a). Among them, the carboxyphenyl-containing dyes can exist in their spiro-lactone forms in equilibration with the ring-opened zwitterionic forms. The spiro-lactone ring-opening and -closing conversion process offers fluorescence on–off signalling in the fluorescence sensing of biological systems (Fig. 2b).6 The photophysical properties, particularly, the emission wavelengths, of the xanthene dyes are affected by: (a) the amino substituent (in red, Fig. 2c), (2) the C-9 central atom (in orange, Fig. 2c), and (3) the conjugation length (in blue, Fig. 2c).
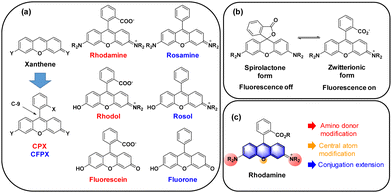 |
| Fig. 2 (a) Structures of C-9 phenyl-substituted xanthene dyes. (b) Spiro-lactone and zwitterionic forms of rhodamines. (c) Modifications to extend the emission wavelengths of rhodamines. | |
2.1.
o-Carboxyphenyl-substituted xanthene dyes
2.1.1. The amino group effect.
The alkyl amines of the push–pull type fluorophores act as electron-donating groups in conjugation with their iminium forms, which significantly affect the emission properties of the fluorophore.4 When going from rhodamine 123 (–NH2) to rhodamine 6G (–NHEt) and rhodamine B (–NEt2), the emission maximum shows bathochromic shifts from 532 nm to 553 nm and 576 nm (in ethanol), respectively. The emission wavelengths of the dialkylamine-substituted rhodamines (rhodamines B, rhodamine 101, and rhodamine 630) are in the order: diethylamine (576 nm) < julolidine (595 nm) < ethyl-indoline (616 nm) (Fig. 3). From the results, it is concluded that a better electron-donating amino group is preferred for the bathochromic emission shift of such rhodamine dyes. However, the maximum emission wavelengths of those “monoamine-substituted” rhodamines are still not reached in the deep-red region by modifying the monoamine substituent alone. Zhang and co-workers disclosed that using xanthene dyes with “diamino” substituents, instead of the mono-amino donors, is an effective way to induce significant bathochromic shifts in their absorption and emission maxima, as demonstrated with new deep-red/NIR emitting pyronine and silapyronine analogues.8–10
 |
| Fig. 3 The effect of the amino group on the absorption and emission wavelengths of rhodamine dyes,7 observed in ethanol. | |
2.1.2. Central atom replacement.
The C-9 central atom of the xanthene dyes can be chosen from the carbon family (group 14: C, Si, Ge, and Sn), leading to various xanthene analogues,11–23 as well as from the nitrogen family (group 15; N and P)16,24 or chalcogen family (group 16; O, S, Se, and Te)16,25–28 (Fig. 4). The replacement of the central oxygen atom in the xanthene dyes leads to variable bathochromic emission shifts, and the underlying mechanisms are also different. For the atoms of groups 14 and 15, it was explained that the X atom (Si, Ge, Sn, and P) can stabilize the LUMO energy levels by the σ*–π* conjugation between the σ* orbitals of the exocyclic X–C or X
O bond (for example, –SiMe2, –P(
O)OH, –P(
O)Me, and –P(
O)Ph) and the π* orbital of the fluorophore nearby.29,30 For the atoms of group 16, the heteroatom lone pair occupancy and resonance stability are found to be the major determinants of the excitation energetics: the X atom of S, Se, or Te showed increased lone pair occupancy, which lowers the excitation energy and thus leads to longer absorption wavelengths.27
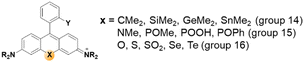 |
| Fig. 4 Candidate elements/groups for the replacement of central atom X. | |
A systematic variation of the central oxygen atom of rhodamines has been conducted by Lavis and co-workers.16 The maximum absorption and emission wavelengths of rhodamine analogues with amine (nitrogen), sulfur, sulfone, carbon, silicon, phosphinate, or phosphine oxide at the central C-9 are listed in Fig. 5. By the substitution of the central atom, the bathochromic emission wavelength shift increased in the order of N, O, S, C, Si, and P. Together with the known red-shift effect by the azetidine group, the maximum emission wavelength of 723 nm (pH 7.3 HEPES buffer) was realized with the –P(
O)Ph analogue. Note that the replacement of the C-9 oxygen atom with silyl (–SiR2) groups to obtain deep-red/NIR-emitting dyes has received much attention, plausibly owing to the synthetic feasibility. The replacement of the C-9 oxygen atom with the dimethylsilyl group, for example, leads to increased electrophilicity at C-10. Accordingly, the spiro-lactone ring-opening takes place in media of rather higher polarities compared to the case of the corresponding rhodamine analogues. Recently, a rosamine analogue with a boron substituent at C-9 (B-(OH2)) was also reported; the rosamine borinic acid emits at around 650 nm.31
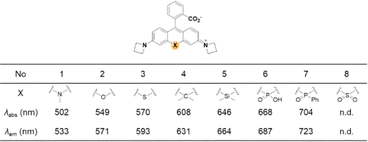 |
| Fig. 5 Effect of the replacement of the central atom (X) on the maximum absorption/emission wavelengths of a rhodamine dye,16 observed in HEPES at pH 7.3 (10 mM). | |
2.1.3. π-Conjugation extension.
The extension of the π-conjugation length of a fluorophore is a common way to shift its absorption/emission maxima. Herein, rhodamine derivatives with a fused benzene ring, called benzorhodamine dyes, are discussed. Depending on the site of ring fusion, they can be categorized into linear- and bent-shape benzorhodamine dyes.
Up to now, four types of geometrically different benzorhodamines have been reported: benzorhodamine a-I,32b-II,33c-I34 and c-II35 types. These benzorhodamines showed significantly red-shifted emission wavelengths at more than 635 nm, reaching 785 nm in the case of linear b-II. Recently, Liu and co-workers disclosed a novel π-extension approach based on computational calculations, which led to dual-emission rhodamine analogues from single-emission rhodamines. The π-extended rhodamines composed of selected fragments with matched FMO energy levels avoided the otherwise possible inter-fragment PeT.36
At this point, it is worthwhile to mention a synthetic challenge encountered in the context of linear benzocoumarin dyes (Fig. 6b). A condensation reaction of an amino-naphthol such as 1 with keto-benzoic acid 2 does not lead to the linear type but the a-I type bent benzorhodamine exclusively because the C-1 site of compound 1 is more nucleophilic than the C-3 site toward electrophiles. The regioselectivity issue was solved by introducing steric hindrance near the electrophilic C-1 site, leading to linear benzorhodamines.33 Linearly conjugated dipolar dyes usually absorb and emit wavelengths longer than the corresponding bent analogues.37
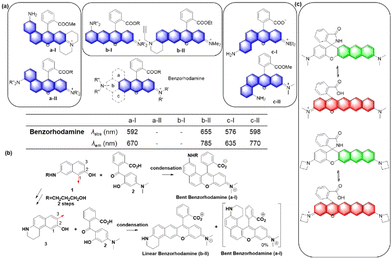 |
| Fig. 6 (a) Structures of benzorhodamines. (b) Development of linear b-II type benzorhodamines. (c) Dual-emission π-extended rhodamines. | |
2.1.4. CPX-based bioimaging probes, selected examples.
The rhodamine and rhodol analogues can be developed into fluorescent probes, mostly activatable or reaction-based probes, by introducing reactive groups at the spiro-lactone or the N/O-donor groups, similar to the case of their parent dyes. The rhodamine spiro-lactam ring-opening process triggered by target analytes is an established strategy to realize fluorescence off–on signalling.6 For example, rhodamine lactams F1 and F2 undergo the spirolactam ring-opening triggered by a reactive oxygen species, hypochlorous acid (F1, Fig. 7a),38 and Cu2+(F2, Fig. 7a), respectively,39 followed by hydrolysis to generate the corresponding fluorescent rhodamines. On the other hand, introducing a reactive group for the target analyte at the hydroxyl or amino (–OH or –NHR) donors in the rhodamine/rhodol/fluorescein dyes results in the corresponding nonfluorescent spiro-lactone forms, which, upon the treatment with target analytes, can regenerate the original fluorescent dyes. For example, the hydroxyl donor of the rhodol analogue protected with β-galactose reacts with β-galactosidase to generate the rhodol analogue. This process is accompanied by fluorescence off–on signalling.20 Similarly, F4 bearing a quinone substrate specific to human NAD(P)H quinone oxidoreductase-1 (hNQO1) undergoes enzyme-catalyzed reduction to give rise to hydroquinone, which triggers intramolecular cyclization followed by amide bond cleavage to regenerate the amino donor.40 In this case, the quinone moiety can also act as a fluorescence quencher through photoinduced electron transfer (PeT); hence, the removal of the quinone moiety can cause the observed off–on fluorescence signal change.
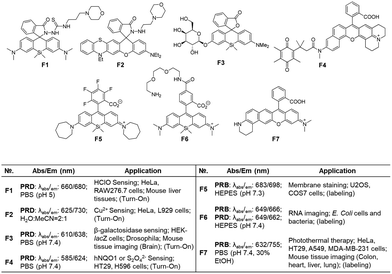 |
| Fig. 7 Selected examples of fluorescent probes based on rhodamine and rhodol analogues. | |
The fluorophores can be also applied to the labelling of the cell membrane (F5, Fig. 7d)19 and RNA (F6, Fig. 7d).21 As the dyes are in the fluorescence-on state, washing-out steps are required in such applications.
Recently, Dai and co-workers have developed a synthetic route to obtain linear benzorhodamines. In the free carboxylic acid form such as F7, it absorbs light efficiently but emits weaker fluorescence compared to its ester form, which has the potential for photothermal therapy.33 The linear benzorhodamine in the ester form is useful for NIR imaging.
2.2. Carboxy-free, phenyl-substituted xanthene fluorophores
2.2.1. Amino donor effect in rosols.
Chin and co-workers reported that stronger electron-donating amino groups cause emission bathochromic shifts in rosol derivatives.41 A similar trend was observed among the different amino donors in the case of rhodamine and rosamine dyes. In the case of the julolidine (a bicyclic amino donor) derivative Jul-Rosol, the emission maximum reached 574 nm, which is still away from deep-red wavelengths. Interestingly, a diamine-substituted analogue THQ-Rosol emits in the NIR region (λem = 710 nm) with a large bathochromic shift (Δλ = 136 nm) from that of Jul-Rosol (Fig. 8), an important observation for the future development of NIR-emitting dyes.
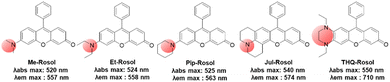 |
| Fig. 8 The amino donor effect on the photophysical properties of rosol dyes.41 The data were obtained in pH 7.4 phosphate buffer (50 mM, 150 mM NaCl). | |
2.2.2. Central X atom replacement.
Both the rosol (Fig. 9a) and rosamine (Fig. 9b) systems show a similar level of spectral shifts to the case of rhodamines. Together with the data in Fig. 5, we can make rosol, rosamine, and rhodamine dyes emit in the deep-red or even in the NIR wavelength region by replacing their central oxygen atom with Si, POPh, POOH, POMe, or SO2.
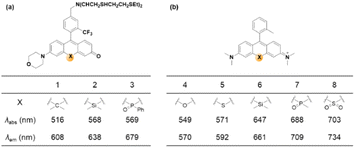 |
| Fig. 9 Effects of central atom replacement on the photophysical properties of rosol (left) and rosamine (right). Data for dyes 1–3 are measured in HEPES buffer (25 mM, pH 7.4),42 and those for dyes 4–8 are measured in PBS (20 mM, pH 7.4).43 | |
As noted above, the replacement of the central oxygen atom of rhodamines with silyl groups affords the corresponding sila-rhodamine analogues (Si-rhodamines), which offer several advantageous features for bioimaging applications, such as deep-red emission44 and enhanced two-photon (TP) properties.45,46 Si-rhodamines have been also used for super-resolution imaging.47 The replacement of the C-10 phenyl group of Si-rhodamines with an amine leads to the so-called amino-Si-pyronins that show unique optical properties. In contrast to the parent Si-rhodamines that show narrow Stokes shifts, amino-Si-pyronins exhibit large Stokes shifts. In addition, amino-Si-pyronins have excellent two-photon imaging capability, which is lacking in the case of Si-rhodamines. Si-rhodamines undergo a medium polarity-dependent spiro-lactone ring-opening and -closing equilibrium: they are highly fluorescent in the ring-opened form in highly polar media but nonfluorescent in the ring-closed form in nonpolar media. Amino-Si-pyronins can also exist in the fluorescence-on and -off states depending on the medium polarity. In highly polar media, amino-Si-pyronins are strongly fluorescent in the enamine form: contrarily, in nonpolar media, they are nonfluorescent in the imine form. This enamine–imine equilibrium is also dependent on the 2- and 7-amine substituents.46 A promising aspect of amino-Si-pyronins is that we can further functionalize the C-10 amino group, such as via the introduction of an acyl or analogous group onto it. In this case, the electrophilicity of C-10 also increases; hence, biothiols such as cysteine can react with some acylated amino-Si-pyronins. This chemical stability issue can be solved by the julolidine-derived amino-Si-pyronins that are stable in cells. The resulting acyl analogues now emit in the NIR region, leading to a novel type of NIR dyes. Notably, amino-Si-pyronins and their NIR analogues constitute a novel sensing platform for the development of activity-based (activatable or reaction-based) probes. For example, two-photon ratiometric probes can be developed by introducing H2O2- or NTR-responsive moieties onto the C-10 amino group. In this case, the emission maxima change from a NIR to a deep-red region (hypochromic shift, Fig. 10b). Also, ratiometric sensing systems that show signal changes from the deep-red to NIR region (bathochromic shift, Fig. 10c) can be conceivable, as exemplified by an HOCl probe (more examples are provided in the application section below).
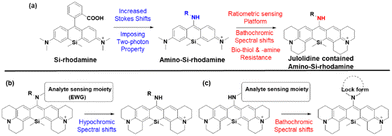 |
| Fig. 10 (a) Development of chemically stable amino-Si-pyronins. Activatable sensing platforms based on the julolidine-derived amino-Si-pyronins for the ratiometric sensing of analytes with (b) bathochromic spectral shifts and (c) hypochromic spectral shifts. | |
2.2.3. Conjugation extension.
Strongin and co-workers first developed all six types of benzofluorones (named SNAFR, Fig. 11a),48 whose photophysical properties are altered similarly to the case of benzorhodamines. Among the benzofluorones, the b-I type has poor solubility and also shows marginal red-shifts. Other five types of benzofluorones emit in the red region (a-I, 653 nm; c-I, 629 nm) or even in the NIR region (a-II, 713 nm; b-II, 733 nm; c-II, 757 nm). Such benzene-fused dyes are expected to have enhanced photo- and chemo-stability compared to the highly π-conjugated dyes with vinyl units, such as cyanine and hemicyanine dyes, which can undergo addition and oxidation side reactions with highly nucleophilic or reactive oxygen species (HSO3−, HOCl, ONOO−, etc.). Ahn and co-workers synthesized the linear-shaped benzene-fused rosol or rosamine analogues. The synthetic route, which is different from the case of rhodamines, required the triarylmethanol intermediate 3 (Fig. 11b), which, upon demethylation and intramolecular cyclization, afforded the desired benzorosol.49,50 Furthermore, a free amine-containing benzorosamine, which provides a tunable arm for probe development, was also synthesized. Notably, the linear benzorosol and benzorosamine dyes have high chemo- and photo-stability and emit in the NIR region in cells. Recently, Yang and co-workers unveiled an innovative ring-fusion strategy for the construction of highly intricate fluorophores with extensive ring structures. Employing a symmetrical approach to ring-fusion, they successfully synthesized a set of exquisitely π-conjugated dyes, namely ECX, ESi5 and EC7 (Fig. 11c).51ECX, with its five fused rings, outperformed the benzorosamine that emits at ∼740 nm by significantly pushing the emission maximum to ∼920 nm. ESi5 dyes, which have a modifiable arm attached to the amine sides and bear a central Si atom, display emissions across the range of 895–940.52 Subsequently, the same group further extended the core to seven fused rings, resulting in EC7 dyes,53 which pushed the emission wavelength even up to 1285 nm. These dyes have immense potential for clinical applications, allowing for enhanced light penetration within biological systems.
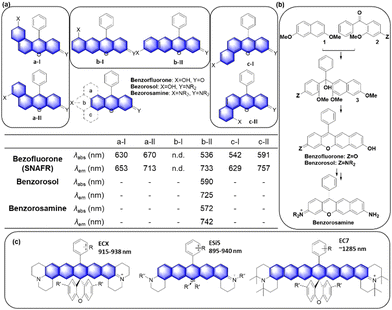 |
| Fig. 11 (a) Structures of the benzofluorone, benzorosol and benzorosamine dyes. (b) Synthetic scheme of the linear benzofluorone, benzorosol and benzorosamine dyes. (c) Structures of ECX, ESi5 and EC7 dyes featuring extensive ring fusion. | |
2.2.4. CFPX-based bioimaging probes, selected examples.
Developing activatable probes based on the carboxyl-free phenyl-substituted xanthene dyes, such as rosol and rosamine dyes, requires an extra group that can quench or change their fluorescence signals. A popular approach is to introduce a reactive group, which also acts as a quencher through the PeT process, at the N/O site or phenyl site. Upon interaction with a target analyte, the attached group undergoes a cleavage reaction, restoring the dye's fluorescence. Such turn-on type fluorescence probes have been developed for cuprous ions (F8–F10, Fig. 12a),42 formaldehyde (F11, Fig. 12a),54 azoreductase (F12, Fig. 12a),55 and peroxynitrite (F13, Fig. 12a).56
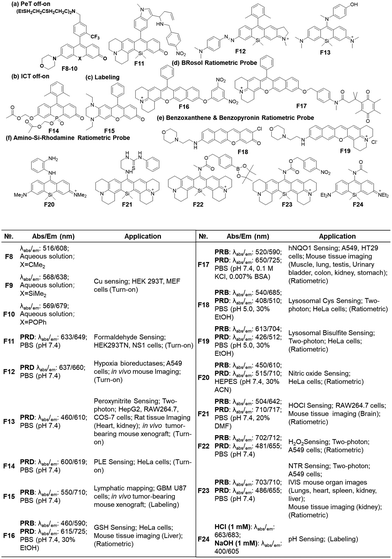 |
| Fig. 12 Selected examples of fluorescent probes developed from the rosol, rosamine, fluorone, benzoxanthene, benzopyronin and amino-Si-rhodamine dyes: probes’ basic optical properties and target analytes. | |
Another popular approach is to introduce an activatable group into the dyes in such a way that its introduction causes a change in intramolecular charge-transfer (ICT). The detachment of the reactive group by the target analyte can cause fluorescence signal changes. When the hydroxyl group of rosol and fluorone dyes is protected by the reactive group (Rg), the resulting probes mostly become nonfluorescent (ICT-off state). The activation/cleavage of Rg upon the interaction with the target analyte leads to a turn-on fluorescence response (ICT-on), as exemplified by an esterase probe F14 (Fig. 12b).57 The ICT modulation approach can be also explored for the labelling of biomolecules, as exemplified by rosol F15 (Fig. 12c), which allows lymphatic mapping.41 The commonly observed ICT- or PeT-off state of O-blocked rosol dyes can be tuned into the ICT- or PeT-on state by extending the conjugation length of dyes. The benzorosol (BRosol) dye system developed by Ahn and co-workers provides emissive derivatives even when the hydroxyl group is functionalized into its O-acyl or O-ethereal derivatives49 or connected with a typical PeT quencher (nitrophenyl or quinone groups).49,58 The further π-conjugation keeps the localized excited states of the O-functionalized derivatives still emissive. Also, π-conjugation causes a lowering of the LUMO energy, effectively prohibiting the otherwise feasible occurrence of the PeT process. Notably, there is a large difference in emission wavelength between the phenolic (λem = 590 nm) and phenolate (λem = 725 nm) forms of BRosol, which offers an excellent ratiometric sensing platform to sense GSH (F16, Fig. 12d)49 and hNQO1 (F17, Fig. 12d).58
In 2019, Gryko and co-workers reviewed the synthesis, photophysical properties and applications of rhodol (and rosol) as fluorescent probes. They predicted the development of a π-extended rhodol compound (Fig. 12e, benzoxanthene), which was successfully prepared in 2020, along with its amine analogue (Fig. 12e, benzopyronin). Taking advantage of the fact that the skeleton can be readily attacked by nucleophilic analytes through 1,6-conjugate addition, they were developed into fluorescent probes for cysteine (Cys) (F18, Fig. 12e) and59 bisulfite (F19, Fig. 13e).60
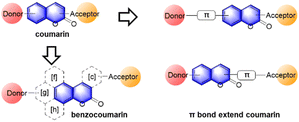 |
| Fig. 13 Strategies to make coumarin absorption/emission wavelength bathochromic-shift. | |
As we have mentioned above, the probes based on the amino-Si-rhodamine system can be developed into probes that show either hypsochromic (Fig. 10b) or bathochromic (Fig. 10c) emission spectral shifts, depending on the probe design: the probes for H2O2 (F20, Fig. 12f),46 NTR (F21, Fig. 12f),61 and pH (F22, Fig. 12f)62 exhibit hypsochromic signal changes, whereas those for nitric oxide (F23, Fig. 12f)63 and HOCl (F24, Fig. 12f) exhibit bathochromic signal changes.64
2.3. Xanthene derivatives: summary
In the above subchapters, we have overviewed and discussed strategies toward the emission bathochromic shift applied to the xanthene fluorophores, such as rhodamine, fluorescein, and their analogues. Commonly used approaches are ring fusion, central atom replacement, and variation of the amino donor. The replacement of the primary amino group (rhodamine 123) with a tertiary amino group (rhodamine 630) results in the emission maximum shift from 532 nm to 616 nm (Δλ = 84 nm; ethanol). The central O atom replacement of a rhodamine dye to Si and P results in the emission maximum shift from 571 nm to 664 nm (Δλ = 93 nm) and 723 nm (Δλ = 152 nm) in pH 7.3 HEPES, respectively (Fig. 5). The linear fusion of one benzene ring to rhodamine B led to a benzorhodamine (b-II, Fig. 6), which resulted in the emission maximum shift from 576 nm to 785 nm (Δλ = 209 nm; rhodamine B in EtOH, benzorhodamine in 30% EtOH/PBS). The ring-fusion approach is highly effective for the emission bathochromic shift, as highlighted by the seven ring-fused EC7 dyes that emit at ∼1285 nm.
3. Coumarin
Considering that the coumarins are typical ICT dyes, we can induce bathochromic shifts in their emission wavelength by increasing the donor/acceptor's ability, in addition to extending the π-conjugation length.
In 1984, Jones and co-workers investigated the photophysical properties of coumarin dyes with several different electron-donor and -acceptor groups.65 However, the modification of the donor and acceptor alone is not sufficient to make the coumarin emit at more than 600 nm (λem). Therefore, π extension is required to generate coumarin derivatives emitting in the red/far-red or NIR region.
3.1. Benzocoumarins
3.1.1 Robust ring-fusion stratagem.
Various benzocoumarin dyes have been reported,66–68 which can be categorized by the ring-fusion geometry: benzo[c]coumarins, benzo[f]coumarins, benzo[g]coumarins and benzo[h]coumarins (Fig. 13a). Among them, benzo[g]coumarins emit in longer wavelengths than the others, and are thus promising for bioimaging applications.68
In 2017, Ahn and co-workers designed a novel strategy to modify a typical D–A coumarin dye to far-red emitting benzo[g]coumarins.4 The emission maximum data (Fig. 13b) indicate that the π-system extension causes a greater spectral bathochromic shift compared to donor/acceptor modifications. Through a series of modifications (Fig. 14b), a bathochromic emission spectral shift of 241 nm was obtained (in EtOH) compared to that of the original coumarin.
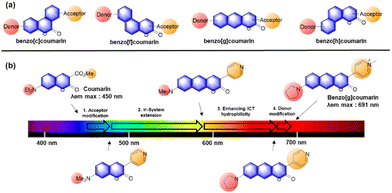 |
| Fig. 14 (a) Different types of benzocoumarin. (b) Structural modification of a coumarin dye (data collected in EtOH). | |
3.1.2. Bioimaging probes based on benzocoumarins.
The pyridinium-benzocoumarin dyes emit in the near-infrared (NIR) wavelength region and have good TP properties and thus have been used for deep-tissue imaging (F25, Fig. 15a).4 By introducing a mitochondria-targeting moiety such as triphenylphosphonium (–PPh3+), a benzocoumarin dye has been used for monitoring mitochondrial trafficking (F26, Fig. 15a).69 In 2020, a systematic study on the photophysical brightness of benzocoumarin dyes in different media revealed that benzothiazole–benzocoumarin dyes are super-bright in cells in comparison with typical conventional dyes (F27, Fig. 14a).70 The work also highlights that the cellular environment offers the third space for organic fluorophores in addition to organic and aqueous media.71 The dipolar nature of benzocoumarin dyes, specifically in the case of F28, makes it useful in detecting lipid droplets.72
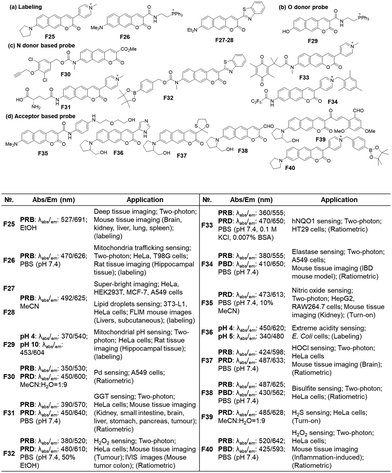 |
| Fig. 15 Applications of benzo[g]coumarins to develop fluorescent probes and their basic optical properties and target analytes summarized. | |
Because the benzocoumarin core is rather difficult to functionalize, the probe design is mainly conducted by modification of the donor (hydroxyl or amino) or acceptor (carboxyl) groups. In 2016, a hydroxy-benzocoumarin dye was utilized for pH sensing, as its hydroxyl and alkoxy (–OH/–O−) forms exhibit different photophysical properties (F29, Fig. 15b).73
In the case of benzocoumarins bearing an amino donor (–NRR′) at the 7-position, modification of them into amide or carbamate derivatives commonly leads to a change in their ICT properties. These properties can be used to develop reaction-based ratiometric probes: accordingly, several ratiometric probes have been developed for sensing Pd (F30, Fig. 15c),74 GGT (F31, Fig. 15c),75 H2O2 (F32, Fig. 15c),76 hNQO1 (F33, Fig. 15c),77 and elastase (F34, Fig. 15c).78
Similarly, ratiometric probes can be developed by modifying the acceptor site, leading to probes for sensing nitric oxide (F35, Fig. 15d),79 extreme acidity (F36, Fig. 15d),80 HOCl (F37, Fig. 15d),81 bisulfite (F38, Fig. 15d),82 H2S (F39, Fig. 15d),83 and H2O2 (F40, Fig. 15d).84
3.2 Vinyl-extended coumarins
3.2.1. Vinyl extension stratagem.
Instead of the ring fusion to extend the electron delocalization as shown with benzocoumarins, we can also extend pi-conjugation simply by inserting vinyl groups between the C-3 substituent and the coumarin ring, although such an approach does not guarantee that the vinyl-containing acceptors have sufficient stability to certain reactive biological species (reactive oxygen and nitrogen species) and also to photochemical reactions.85 Not only the vinyl group86 but also other groups are possible for the pi-extension, such as alkynyl,87 thiazole,88 thiophene,89 benzothiadiazole,90 or a combination of them.91 For example, when a coumarin was subjected to the vinyl extension strategy with a pyridinium acceptor, its emission maximum was red-shifted from 450 nm to 650 nm. An extensive vinyl extension reported by Avhad and co-workers further pushed the emission maximum up to 756 nm (Fig. 16).89 Note that for such an extensive vinyl extension, a powerful electron-acceptor group is required to induce sufficient ICT and thus significant emission red-shift.
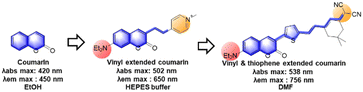 |
| Fig. 16 Transforming coumarin into a NIR-emitting dye through an extensive vinyl extension. | |
As noted above, the vinyl-extension strategy (Fig. 16) can make the resulting electron-acceptor moiety vulnerable to both nucleophilic and electrophilic species, such as bisulfite, biothiols, hypochlorous acid, peroxynitrite, etc. The chemical and photochemical stability of the vinyl-containing acceptors including hemicyanine moieties seems to be dependent on the whole dye system: the vinyl-extended coumarins bearing 2-indolinum or 2-benzothiazolium groups are not chemically and photochemically stable, whereas those of the 4-pyridinium group have sufficient chemo- and photo-stability.85 Besides, the free bond rotation around the vinyl group provides the resulting dyes with viscosity- and polarity-sensitive emission behaviour, leading to fluorescent probes for these environmental parameters.92
3.2.2 Bioimaging probes based on vinyl-extended coumarins.
The vinyl-extended coumarin fluorophores can be classified into four types based on their application modes. They can be used for labelling purposes; in this case, there are no structural changes during fluorescence monitoring (Fig. 17a). They are used for staining DNA and related biomolecules (F41–F43, F48 & F49, Fig. 17a),93–98 binding-induced heparin sensing (F44, Fig. 17a),99 and polarity sensing (F45 & F46, Fig. 17a).100 If there is a basic nitrogen-containing heterocycle in the electron-acceptor side, such as indole or quinolone, protonation leads to a change in the fluorescence signal and thus such dyes can be used for pH sensing (F50–F52, Fig. 17b).101,102 Besides, a vinyl-extended coumarin bearing a 2,3-dihydroindole, which can react with hydroxyl radicals to form a more stable 2-indolinum was used for monitoring of hydroxyl radicals (F53 & F54, Fig. 17b).103,104
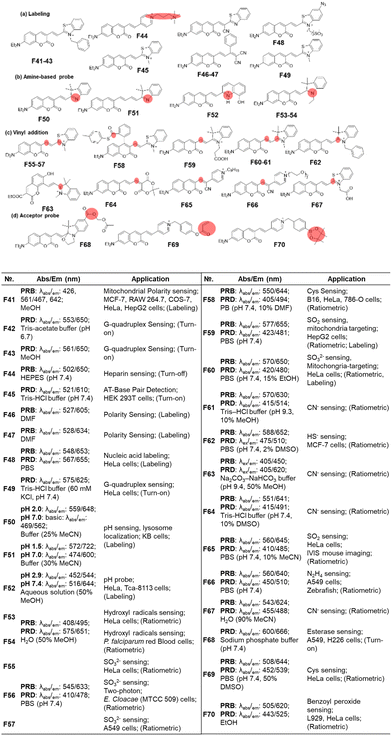 |
| Fig. 17 Vinyl-extended coumarins as fluorescent probes for bioanalytes: basic optical properties and target analytes. | |
The most popular use of such vinyl-extended coumarins is found in sensing of nucleophilic species, such as bisulfite (F55–F57, F59, F60 & F65, Fig. 17c),105–110 cysteine (F58, Fig. 17c),111 cyanide (F61, F63, F64 & F67, Fig. 17c),112–115 hydrogen sulfide (F62, Fig. 17c),116 and hydrazine (F66, Fig. 17c).117 Note that these hemicyanine moieties may also undergo electrophilic reactions with hypochlorous acid or peroxynitrite,85 which warrant careful interference analysis against these species.
For the last type, it is also possible to cause a fluorescence signal change by further decorating the acceptor part (Fig. 17d), as shown in the detection of esterase (F68, Fig. 17d),118 cysteine (F69, Fig. 17d)119 and benzoyl peroxide (F70, Fig. 17d).120
4. Naphthalene
4.1. Extended naphthalene
In previous work, we have disclosed how the donor group affects the emission properties of donor–acceptor type naphthalene compounds including ACEDAN: an amino donor that exerts little allylic 1,3-strain to the naphthalene ring and has high electron-donating capability. This endows the resulting dipolar dye with higher fluorescence in water as well as in cells.121 By fixing the amino donor with pyrrolidine, we have changed the acceptor part into a different heterocycle. Considering the chemo- and photo-stability of the resulting hemicyanine dyes, the dye with 4-pyridinium is the dye of choice for biological applications. With such modifications, the resulting napthylvinylpridinium (NVP) derivative emits at 692 nm (in ethanol), dramatically displaying a red-shift compared to naphthalene's emission at 334 nm in cyclohexane (Fig. 18). Later, Song replaced the pyridinium moiety of NVP with a tricyanofuranyl ring to form TCFAN (F75 in Fig. 19). This conversion even pushes the emission maximum up to 800 nm.92
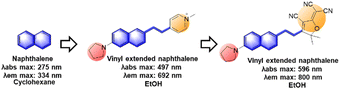 |
| Fig. 18 Transformation of naphthalene to an NIR-emitting dye through an extensive vinyl extension. | |
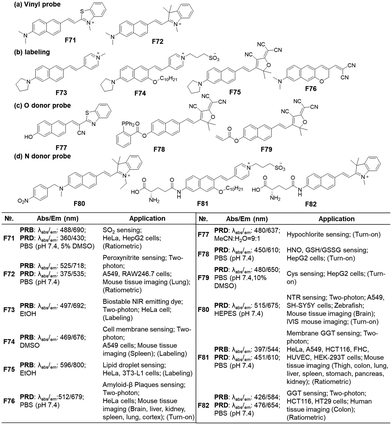 |
| Fig. 19 Vinylnaphthalene-based fluorescent probes for bioanalytes: basic optical properties and target analytes. | |
4.2. Bioimaging probes based on vinylnaphthalene dyes
As mentioned above, the vinylnaphthalene-based hemicyanine dyes bearing an electron acceptor such as 2-indolinum or 2-benzothiazolium moieties have been widely used in monitoring nucleophilic analytes like bisulfite (an aqueous equilibrium species of SO2; F71, Fig. 19a),122 peroxynitrite (F72, Fig. 19a), etc.,123 which suggest that they are susceptible to these species. Also, such hemicyanine dyes are not photochemically stable. In contrast, their 4-pyridinium analogues (NVP dyes) have good stability against many bio-analytes, including Cys, GSH, H2O2, HSO3−, HOCl, and H2S (F73, Fig. 19b) as well as good photostability.85 The NVP system was used to develop a membrane-localizing fluorophore (F74, Fig. 19b) and other probes.124,125 Instead of the charged heterocycles, a charge-neutral but strong electron-withdrawing group, such as 2-(3-cyano-4,5,5-trimethyl-2(5H)-furanylidene)malononitrile (TCF), can be introduced to develop a NIR-emitting probe for lipid droplets, which provide a nonpolar and viscous environment to the dipolar dye (F75, Fig. 19b).92 Essentially, any dipolar dyes, which show polarity (and also viscosity) sensitive emission behaviour, can be used to sense lipid droplets. Also, environment-sensitive π-extended dipolar dyes can be used to detect amyloid-β (Aβ) plaques, which provide a much less polar and congested environment to the dipolar dye compared to the case where the dye resides outside the plaques. This effect is evidenced by a robust deep red-emitting Aβ probe (F76, Fig. 19b).3
Instead of the amino donor, the hydroxyl group can act as an electron donor. Additionally, the dyes bearing such a hydroxyl donor show emission changes depending on the medium pH that governs their equilibrium between the hydroxyl and alkoxy forms. Accordingly, such 6-hydroxy-naphthalene dyes have been used to develop fluorescent probes for hypochlorite (F77, Fig. 19c),126 HNO + GSH/GSSG (F78, Fig. 19c),127 and Cys (F79, Fig. 19c).128 On the other hand, as the fluorescent probes based on aryl alcohol (ArOH) or phenolic dyes generally show pH-dependent emission behaviour, their use in the quantitative analysis should be conducted carefully by measuring the pH-dependent emission signal change.
In the case of dipolar dyes bearing an amino donor, we can develop activatable probes by introducing an analyte-reactive moiety onto the amino group. Such functionalization of the amino donor causes a significant change in the ICT of the dyes; this property allows us to develop activatable probes, as demonstrated for NTR (F80, Fig. 19d)129 and GGT (F81 & F82, Fig. 19d).130,131 When the reactive group is a fluorescence quencher, such as nitroaryl, we may observe a turn-on type fluorescence signal change. However, we may generate a ratiometric probe even in such a case wherein it could modulate the PeT energy levels by choosing an appropriate fluorophore.77
In the above three sections, we have described known strategies to have bathochromic emission shifts of the common fluorophores (benzene-substituted xanthene, coumarin, and naphthalene). Note that other hybrid dye systems are developed as well. Two important hemicyanine types are the CS dye132 and HD dye133 developed by Lin's group, which have been widely used in probe development as well as other bio-applications.134–136 Such a hybridization approach is also a useful method to obtain deep-red/NIR-emitting fluorophores; however, none of the original fluorophore's structure has been conserved in those dye systems and thus they are excluded in this review.
5. Molecular probe design
If we set aside the probes used to detect environmental parameters such as pH, polarity, viscosity, etc. (for example, F26, F48, F75), we can classify the molecular probes into two types based on the target detection mode: (1) binding-induced and (2) reaction/activity-induced. The latter detection mode has been widely explored recently, leading to a vast number of reaction-based/activatable probes.137–139 Note that such activatable probes provide time-lapsed accumulated concentration/activity levels, whereas the binding-based probes allow us to monitor the dynamic concentration/activity information on the target analyte. To develop the binding-based probes, we explore fluorescence signal changes (wavelength or intensity) upon binding with the target analyte. Accordingly, for developing such probes, fluorophores whose photophysical properties are sensitive to environmental changes, such as the electron push–pull type, dipolar dyes, are generally utilized. However, the rationale design of binding-based probes, in particular for enzymes, is challenging because we should consider a substrate that binds specifically to the target enzyme and the binding process should cause a fluorescence signal change. On the other hand, reaction-based probes have flourished recently, as benefited from a variety of chemical conversions that can be selectively executed by target analytes. We have provided selected examples of such activatable probes, which sense a variety of biologically important analytes. To develop activatable probes, we introduce a reactive group (Rg) typically at the donor or acceptor site (Fig. 20). The reactive group can be introduced to interrupt the spirolactone ring-opening process in the case of the CPX families (P1, P2 in Fig. 20). A part of a fluorophore, such as a vinyl unit, can also be the reactive site for certain analytes (P6, P7 in Fig. 20).
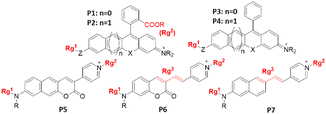 |
| Fig. 20 Potential sites for a reactive group (Rg) in several activatable probes selected. | |
When the reactive group undergoes an analyte-specific chemical conversion (cleavage or conversion into another structure), it involves an ICT change, thus resulting in fluorescence signal changes. The fluorescence change can be turn-off, turn-on, or ratiometric modes depending on the reactive group and fluorophore. Even if the reactive group acts as a quencher, we can realize turn-on or ratiometric signalling by choosing an appropriate fluorophore (for example, F4vs.F17, F32vs.F33).
Another general issue to be mentioned is that as the π-conjugation length increases (for example, P2 and P4), the probe may show aggregation-caused quenching (ACQ) behaviour even at the micromolar concentrations. Thus, it is always required to check the cellular aggregation behaviour of a probe for biological applications.
Also, it is necessary to secure the chemical stability of an activatable probe toward various biological analytes, particularly those containing “reactive” functional groups. For example, probes bearing a Michael acceptor are known to undergo conjugation addition reactions with nucleophilic biothiols (F62: hydrogen sulfide, F69: cysteine, F78: glutathione, etc.) and bisulfite anions (F55–F57, F59, F60, and F65). Also, probes containing an electron-rich vinyl unit are known to undergo oxidation reactions with reactive oxygen species, such as peroxynitrite (F72), hypochlorous acid (F77), etc.
6. Conclusion
We have analyzed the conversion strategies employed to achieve emission bathochromic shifts into the red/NIR region, starting from typical organic fluorophores based on three core structures—xanthene, coumarin, and naphthalene. Also, we have listed selected examples of how to apply these fluorophores to molecular probes for biological analytes. We believe that the strategies and their effectiveness compared here will motivate scientists to develop organic fluorophores even emitting in farther wavelengths, such as in the NIR-II region, and also serve as a valuable guide for designing red- and NIR-emitting fluorophores in the future, in addition to choosing a fluorophore in demand.
7. Perspective
(1) The red/NIR emitting fluorophores shown here have the potential for imaging biological analytes at deeper depths with less autofluorescence interference as well as reduced photodamage.
(2) We have analyzed the strategies to make three typical types of fluorophores emit in longer wavelengths, which involve electron-donor/-acceptor modifications, central atom replacement (for xanthene dyes), and conjugation length extension. A proper combination of these strategies to realize further emission wavelength shifts of known dyes and the design of new fluorophore skeletons will pave the way for the translation of fluorescent materials into medical practices in the future.
(3) Some of the known fluorophores emitting in the NIR-I or further in the NIR-II region have conformationally flexible vinyl units in their structures, which make them vulnerable to degradation by photons and chemicals. Also, the conformationally flexible nature makes them poorly emissive in aqueous media, as known with cyanine dyes. These issues should be carefully evaluated in developing fluorophores using the vinyl extension approach. In the pursuit of aromatic ring fusion, a key consideration lies in ensuring adequate solubility in biological media while mitigating the aggregation-caused quenching phenomenon. Furthermore, we must also explore cost-effective synthetic routes to complement these endeavours.
(4) A fluorophore's brightness is influenced by environmental factors, such as viscosity, polarity, pH, and hydrophobicity/hydrophilicity. As a result, it is inadequate to judge a fluorophore's potential for bioimaging application solely based on its quantum yield measured in solution. Indeed, it is not uncommon to see fluorophores with low quantum yields offering bright cellular images.
(5) In designing fluorescent probes, it is highly recommended to predict their optical behaviour (turn-on, turn-off or ratiometric response) through molecular orbital calculations before synthetic efforts. Even with a reactive group that can act as a PeT quencher, we can alleviate the quenching possibility by properly choosing a fluorophore with “mismatched” orbital energies for the PeT process.
(6) The selection of a fluorophore must consider compatibility with currently available optical devices. For instance, in the clinical instrument for fluorescence-guided surgery, lasers operating at 780–800 nm are extensively utilized (based on the FDA-approved ICG dye). Thus, fluorophores that can be excited within this spectral range and emit strong fluorescence have the potential for clinical experiments.
(7) Furthermore, our optimistic outlook for the future of this field encompasses advancements in multiplex imaging and sensing multiple analytes. Currently, multiplex imaging heavily relies on commonly-used emission channels, notably including wavelengths such as 488, 555, 647, and 750 nm. To enhance convenience and efficiency in this area, we envision the incorporation of one or two additional NIR-emitting fluorophores, alongside the utilization of compatible optical instruments. This progressive approach will significantly improve the efficiency of cyclic immunofluorescence imaging, while also enabling the simultaneous staining of a greater number of biomarkers for flow cytometry. As a result, the entire process will be streamlined. Another future direction will be the development of probes for sensing multiple analytes. The current probes mostly target single analytes. Looking ahead, our focus will be on designing probes capable of detecting multiple analytes in a sense of the AND logic gate, with which we could mitigate the false-positive or -negative errors in disease diagnosis. These advancements in probe technology hold immense potential for precise disease diagnosis and detection, facilitating more accurate and comprehensive assessments in clinical settings.
Author contributions
M. D.: writing original draft; writing review & editing; investigation. Y. J. Y.: investigation. S. S.: investigation. K. H. A.: supervision, writing review & editing.
Conflicts of interest
There are no conflicts to declare.
Acknowledgements
This research was supported by the Basic Science Research Program through the National Research Foundation of Korea (NRF) funded by the Ministry of Education (NRF2019R1A2C2085438); this research was also supported by funding from the Cancer Early Detection Advanced Research (CEDAR) centre at the Oregon Health & Science University's Knight Cancer Institute. M. D. gratefully acknowledges Professor Lei G. Wang (BME department, OHSU) for the helpful discussions.
References
- R. Liu, Y. Xu, K. Xu and Z. Dai, Aggregate, 2021, 2, e23 CAS.
- M. Monici, Biotechnol. Annu. Rev., 2005, 11, 227–256 CrossRef CAS PubMed.
- D. Kim, H. Moon, S. H. Baik, S. Singha, Y. W. Jun, T. Wang, K. H. Kim, B. S. Park, J. Jung, I. Mook-Jung and K. H. Ahn, J. Am. Chem. Soc., 2015, 137, 6781–6789 CrossRef CAS PubMed.
- Y. W. Jun, H. R. Kim, Y. J. Reo, M. Dai and K. H. Ahn, Chem. Sci., 2017, 8, 7696–7704 RSC.
- X. Zhang, S. Bloch, W. Akers and S. Achilefu, Curr. Protocol. Cytom., 2012, Chapter 12, 1–12 Search PubMed.
- M. Beija, C. A. Afonso and J. M. Martinho, Chem. Soc. Rev., 2009, 38, 2410–2433 RSC.
- J. Arden, G. Deltau, V. Huth, U. Kringel, D. Peros and K. H. Drexhage, J. Lumin., 1991, 48–49, 352–358 CrossRef CAS.
- G. Jiang, T. B. Ren, E. D'Este, M. Xiong, B. Xiong, K. Johnsson, X. B. Zhang, L. Wang and L. Yuan, Nat. Commun., 2022, 13, 2264 CrossRef CAS PubMed.
- T. B. Ren, W. Xu, W. Zhang, X. X. Zhang, Z. Y. Wang, Z. Xiang, L. Yuan and X. B. Zhang, J. Am. Chem. Soc., 2018, 140, 7716–7722 CrossRef CAS PubMed.
- G. Jiang, X. F. Lou, S. Zuo, X. Liu, T. B. Ren, L. Wang, X. B. Zhang and L. Yuan, Angew. Chem., Int. Ed. Engl., 2023, 62, e202218613 CrossRef CAS PubMed.
- J. B. Grimm, A. J. Sung, W. R. Legant, P. Hulamm, S. M. Matlosz, E. Betzig and L. D. Lavis, ACS Chem. Biol., 2013, 8, 1303–1310 CrossRef CAS PubMed.
- K. Kolmakov, V. N. Belov, C. A. Wurm, B. Harke, M. Leutenegger, C. Eggeling and S. W. Hell, Eur. J. Org. Chem., 2010, 3593–3610, DOI:10.1002/ejoc.201000343.
- Q. Zheng, A. X. Ayala, I. Chung, A. V. Weigel, A. Ranjan, N. Falco, J. B. Grimm, A. N. Tkachuk, C. Wu, J. Lippincott-Schwartz, R. H. Singer and L. D. Lavis, ACS Cent. Sci., 2019, 5, 1602–1613 CrossRef CAS PubMed.
- L. Wang, M. Tran, E. D'Este, J. Roberti, B. Koch, L. Xue and K. Johnsson, Nat. Chem., 2020, 12, 165–172 CrossRef CAS PubMed.
- J. B. Grimm, A. K. Muthusamy, Y. Liang, T. A. Brown, W. C. Lemon, R. Patel, R. Lu, J. J. Macklin, P. J. Keller, N. Ji and L. D. Lavis, Nat. Methods, 2017, 14, 987–994 CrossRef CAS PubMed.
- J. B. Grimm, A. N. Tkachuk, L. Xie, H. Choi, B. Mohar, N. Falco, K. Schaefer, R. Patel, Q. Zheng, Z. Liu, J. Lippincott-Schwartz, T. A. Brown and L. D. Lavis, Nat. Methods, 2020, 17, 815–821 CrossRef CAS PubMed.
- J. L. Bachman, C. I. Pavlich, A. J. Boley, E. M. Marcotte and E. V. Anslyn, Org. Lett., 2020, 22, 381–385 CrossRef CAS PubMed.
- J. Arden-Jacob, J. Frantzeskos, N. U. Kemnitzer, A. Zilles and K. H. Drexhage, Spectrochim. Acta, Part A, 2001, 57, 2271–2283 CrossRef CAS PubMed.
- J. B. Grimm, T. A. Brown, A. N. Tkachuk and L. D. Lavis, ACS Cent. Sci., 2017, 3, 975–985 CrossRef CAS PubMed.
- H. Ito, Y. Kawamata, M. Kamiya, K. Tsuda-Sakurai, S. Tanaka, T. Ueno, T. Komatsu, K. Hanaoka, S. Okabe, M. Miura and Y. Urano, Angew. Chem., Int. Ed. Engl., 2018, 57, 15702–15706 CrossRef CAS PubMed.
- R. Wirth, P. Gao, G. U. Nienhaus, M. Sunbul and A. Jaschke, J. Am. Chem. Soc., 2019, 141, 7562–7571 CrossRef CAS PubMed.
- G. Lukinavicius, K. Umezawa, N. Olivier, A. Honigmann, G. Yang, T. Plass, V. Mueller, L. Reymond, I. R. Correa, Jr., Z. G. Luo, C. Schultz, E. A. Lemke, P. Heppenstall, C. Eggeling, S. Manley and K. Johnsson, Nat. Chem., 2013, 5, 132–139 CrossRef CAS PubMed.
- L. G. Wang, A. R. Montano, J. R. Combs, N. P. McMahon, A. Solanki, M. M. Gomes, K. Tao, W. H. Bisson, D. A. Szafran, K. S. Samkoe, K. M. Tichauer and S. L. Gibbs, Nat. Chem., 2023, 15, 729–739 CrossRef CAS PubMed.
- X. Zhou, R. Lai, J. R. Beck, H. Li and C. I. Stains, Chem. Commun., 2016, 52, 12290–12293 RSC.
- T. C. Binns, A. X. Ayala, J. B. Grimm, A. N. Tkachuk, G. A. Castillon, S. Phan, L. Zhang, T. A. Brown, Z. Liu, S. R. Adams, M. H. Ellisman, M. Koyama and L. D. Lavis, Cell Chem. Biol., 2020, 27, 1063–1072 CrossRef CAS PubMed.
- T. M. McCormick, B. D. Calitree, A. Orchard, N. D. Kraut, F. V. Bright, M. R. Detty and R. Eisenberg, J. Am. Chem. Soc., 2010, 132, 15480–154803 CrossRef CAS PubMed.
- B. Calitree, D. J. Donnelly, J. J. Holt, M. K. Gannon, C. L. Nygren, D. K. Sukumaran, J. Autschbach and M. R. Detty, Organometallics, 2007, 26, 6248–6257 CrossRef CAS.
- A. Chmyrov, J. Arden-Jacob, A. Zilles, K. H. Drexhage and J. Widengren, Photochem. Photobiol. Sci., 2008, 7, 1378–1385 CrossRef CAS PubMed.
- Y. Koide, Y. Urano, K. Hanaoka, T. Terai and T. Nagano, ACS Chem. Biol., 2011, 6, 600–608 CrossRef CAS PubMed.
- X. Chai, X. Cui, B. Wang, F. Yang, Y. Cai, Q. Wu and T. Wang, Chemistry, 2015, 21, 16754–16758 CrossRef CAS PubMed.
- Y. Komori, S. Sugimoto, T. Sato, H. Okawara, R. Watanabe, Y. Takano, S. Kitaoka and Y. Egawa, Sensors, 2023, 23, 1528 CrossRef CAS PubMed.
- M. Sibrian-Vazquez, J. O. Escobedo, M. Lowry and R. M. Strongin, Pure Appl. Chem., 2012, 84, 2443–2456 CrossRef CAS PubMed.
- M. Dai, H. Lee, Y. J. Yang, M. Santra, C. W. Song, Y. W. Jun, Y. J. Reo, W. J. Kim and K. H. Ahn, Chemistry, 2020, 26, 11549–11557 CrossRef CAS PubMed.
- L. G. Wang, I. Munhenzva, M. Sibrian-Vazquez, J. O. Escobedo, C. H. Kitts, F. R. Fronczek and R. M. Strongin, J. Org. Chem., 2019, 84, 2585–2595 CrossRef CAS PubMed.
- L. Wang, C. W. Barth, M. Sibrian-Vazquez, J. O. Escobedo, M. Lowry, J. Muschler, H. Li, S. L. Gibbs and R. M. Strongin, ACS Omega, 2017, 2, 154–163 CrossRef CAS PubMed.
- X. Wu, Y. Gao, W. Chi, C. Wang, Z. Xu and X. Liu, Mater. Chem. Front., 2023, 7, 1137–1145 RSC.
- D. Kim, Q. P. Xuan, H. Moon, Y. W. Jun and K. H. Ahn, Asian J. Org. Chem., 2014, 3, 1089–1096 CrossRef CAS.
- G. J. Mao, Z. Z. Liang, J. Bi, H. Zhang, H. M. Meng, L. Su, Y. J. Gong, S. Feng and G. Zhang, Anal. Chim. Acta, 2019, 1048, 143–153 CrossRef CAS PubMed.
- C. Liu, X. Jiao, Q. Wang, K. Huang, S. He, L. Zhao and X. Zeng, Chem. Commun., 2017, 53, 10727–10730 RSC.
- Q. A. Best, A. E. Johnson, B. Prasai, A. Rouillere and R. L. McCarley, ACS Chem. Biol., 2016, 11, 231–240 CrossRef CAS PubMed.
- K. S. Hettie, J. L. Klockow, T. E. Glass and F. T. Chin, Anal. Chem., 2019, 91, 3110–3117 CrossRef CAS PubMed.
- S. Jia, K. M. Ramos-Torres, S. Kolemen, C. M. Ackerman and C. J. Chang, ACS Chem. Biol., 2018, 13, 1844–1852 CrossRef CAS PubMed.
- F. Deng, L. Liu, W. Huang, C. Huang, Q. Qiao and Z. Xu, Spectrochim. Acta, Part A, 2020, 240, 118466 CrossRef CAS PubMed.
- Y. Koide, Y. Urano, K. Hanaoka, T. Terai and T. Nagano, J. Am. Chem. Soc., 2011, 133, 5680–5682 CrossRef CAS PubMed.
- Z. Mao, H. Jiang, X. Song, W. Hu and Z. Liu, Anal. Chem., 2017, 89, 9620–9624 CrossRef CAS PubMed.
- K. H. Kim, S. Singha, Y. W. Jun, Y. J. Reo, H. R. Kim, H. G. Ryu, S. Bhunia and K. H. Ahn, Chem. Sci., 2019, 10, 9028–9037 RSC.
- Y. Kushida, T. Nagano and K. Hanaoka, Analyst, 2015, 140, 685–695 RSC.
- Y. Yang, M. Lowry, X. Xu, J. O. Escobedo, M. Sibrian-Vazquez, L. Wong, C. M. Schowalter, T. J. Jensen, F. R. Fronczek, I. M. Warner and R. M. Strongin, Proc. Natl. Acad. Sci. U. S. A., 2008, 105, 8829–8834 CrossRef CAS PubMed.
- M. Dai, Y. J. Reo, C. W. Song, Y. J. Yang and K. H. Ahn, Chem. Sci., 2020, 11, 8901–8911 RSC.
- Y. M. Poronik, K. V. Vygranenko, D. Gryko and D. T. Gryko, Chem. Soc. Rev., 2019, 48, 5242–5265 RSC.
- Z. Lei, X. Li, X. Luo, H. He, J. Zheng, X. Qian and Y. Yang, Angew. Chem., Int. Ed. Engl., 2017, 56, 2979–2983 CrossRef CAS PubMed.
- J. Li, Y. Dong, R. Wei, G. Jiang, C. Yao, M. Lv, Y. Wu, S. H. Gardner, F. Zhang, M. Y. Lucero, J. Huang, H. Chen, G. Ge, J. Chan, J. Chen, H. Sun, X. Luo, X. Qian and Y. Yang, J. Am. Chem. Soc., 2022, 144, 14351–14362 CrossRef CAS PubMed.
- R. Wei, Y. Dong, X. Wang, J. Li, Z. Lei, Z. Hu, J. Chen, H. Sun, H. Chen, X. Luo, X. Qian and Y. Yang, J. Am. Chem. Soc., 2023, 145, 12013–12022 CrossRef CAS PubMed.
- A. Roth, H. Li, C. Anorma and J. Chan, J. Am. Chem. Soc., 2015, 137, 10890–10893 CrossRef CAS PubMed.
- K. Hanaoka, Y. Kagami, W. Piao, T. Myochin, K. Numasawa, Y. Kuriki, T. Ikeno, T. Ueno, T. Komatsu, T. Terai, T. Nagano and Y. Urano, Chem. Commun., 2018, 54, 6939–6942 RSC.
- H. Zhang, J. Liu, L. Wang, M. Sun, X. Yan, J. Wang, J. P. Guo and W. Guo, Biomaterials, 2018, 158, 10–22 CrossRef CAS PubMed.
- Y. Fang, G. N. Good, X. Zhou and C. I. Stains, Chem. Commun., 2019, 55, 5962–5965 RSC.
- Y. J. Yang, M. Dai, Y. J. Reo, C. W. Song, S. Sarkar and K. H. Ahn, Anal. Chem., 2021, 93, 7523–7531 CrossRef CAS PubMed.
- U. Tamima, C. W. Song, M. Santra, Y. J. Reo, H. Banna, M. R. Islam and K. H. Ahn, Sens. Actuators, B, 2020, 322, 128588 CrossRef CAS.
- U. Tamima, M. Santra, C. W. Song, Y. J. Reo and K. H. Ahn, Anal. Chem., 2019, 91, 10779–10785 CrossRef CAS PubMed.
- S. Sarkar, H. Lee, H. G. Ryu, S. Singha, Y. M. Lee, Y. J. Reo, Y. W. Jun, K. H. Kim, W. J. Kim and K. H. Ahn, ACS Sens., 2021, 6, 148–155 CrossRef CAS PubMed.
- P. Horváth, P. Šebej, D. Kovář, J. Damborský, Z. Prokop and P. Klán, ACS Omega, 2019, 4, 5479–5485 CrossRef.
- J. Tang, Z. Guo, Y. Zhang, B. Bai and W. H. Zhu, Chem. Commun., 2017, 53, 10520–10523 RSC.
- K. H. Kim, S. J. Kim, S. Singha, Y. J. Yang, S. K. Park and K. H. Ahn, ACS Sens., 2021, 6, 3253–3261 CrossRef CAS PubMed.
- B. P. Czech and R. A. Bartsch, J. Org. Chem., 1984, 49, 4076–4078 CrossRef CAS.
- H. N. Lv, P. F. Tu and Y. Jiang, Mini. Rev. Med. Chem., 2014, 14, 603–622 CrossRef CAS.
- M. Tasior, D. Kim, S. Singha, M. Krzeszewski, K. H. Ahn and D. T. Gryko, J. Mater. Chem. C, 2015, 3, 1421–1446 RSC.
- Y. Jung, J. Jung, Y. Huh and D. Kim, J. Anal. Methods Chem., 2018, 2018, 5249765 Search PubMed.
- A. R. Sarkar, C. H. Heo, H. W. Lee, K. H. Park, Y. H. Suh and H. M. Kim, Anal. Chem., 2014, 86, 5638–5641 CrossRef CAS PubMed.
- Y. J. Reo, Y. W. Jun, S. W. Cho, J. Jeon, H. Roh, S. Singha, M. Dai, S. Sarkar, H. R. Kim, S. Kim, Y. Jin, Y. L. Jung, Y. J. Yang, C. Ban, J. Joo and K. H. Ahn, Chem. Commun., 2020, 56, 10556–10559 RSC.
- K. H. Ahn and Y. W. Jun, Phys. Sci., 2022, 126–129, DOI:10.26904/RF-141-2648143398.
- T. Yoshihara, R. Maruyama, S. Shiozaki, K. Yamamoto, S. I. Kato, Y. Nakamura and S. Tobita, Anal. Chem., 2020, 92, 4996–5003 CrossRef CAS PubMed.
- A. R. Sarkar, C. H. Heo, L. Xu, H. W. Lee, H. Y. Si, J. W. Byun and H. M. Kim, Chem. Sci., 2016, 7, 766–773 RSC.
- S. W. Cho, Y. J. Reo, S. Sarkar and K. H. Ahn, Bull. Korean Chem. Soc., 2020, 42, 135–139 CrossRef.
- Y. J. Reo, Y. W. Jun, S. Sarkar, M. Dai and K. H. Ahn, Anal. Chem., 2019, 91, 14101–14108 CrossRef CAS PubMed.
- S. W. Cho, Y. W. Jun, Y. J. Reo, S. Sarkar and K. H. Ahn, Results Chem., 2021, 3, 100117 CrossRef CAS.
- M. Dai, C. W. Song, Y. J. Yang, H. R. Kim, Y. J. Reo and K. H. Ahn, Sens. Actuators, B, 2021, 330, 129277 CrossRef CAS.
- S. Sarkar, A. Shil, M. Nandy, S. Singha, Y. J. Reo, Y. J. Yang and K. H. Ahn, Anal. Chem., 2022, 94, 1373–1381 CrossRef CAS PubMed.
- Z. Mao, H. Jiang, Z. Li, C. Zhong, W. Zhang and Z. Liu, Chem. Sci., 2017, 8, 4533–4538 RSC.
- H. Kim, S. Sarkar, M. Nandy and K. H. Ahn, Spectrochim. Acta, Part A, 2021, 248, 119088 CrossRef CAS PubMed.
- Y. W. Jun, S. Sarkar, S. Singha, Y. J. Reo, H. R. Kim, J. J. Kim, Y. T. Chang and K. H. Ahn, Chem. Commun., 2017, 53, 10800–10803 RSC.
- U. Tamima, S. Singha, H. R. Kim, Y. J. Reo, Y. W. Jun, A. Das and K. H. Ahn, Sens. Actuators, B, 2018, 277, 576–583 CrossRef CAS.
- M. Santra, S. Sarkar, Y. W. Jun, Y. J. Reo and K. H. Ahn, Tetrahedron Lett., 2018, 59, 3210–3213 CrossRef CAS.
- H. R. Kim, S. Sarkar and K. H. Ahn, Chem. – Asian
J., 2022, 17, e202101317 CrossRef PubMed.
- H. J. Park, C. W. Song, S. Sarkar, Y. W. Jun, Y. J. Reo, M. Dai and K. H. Ahn, Chem. Commun., 2020, 56, 7025–7028 RSC.
- K. Hara, T. Sato, R. Katoh, A. Furube, Y. Ohga, A. Shinpo, S. Suga, K. Sayama, H. Sugihara and H. Arakawa, J. Phys. Chem. B, 2002, 107, 597–606 CrossRef.
- É. Torres, S. Sequeira, P. Parreira, P. Mendes, T. Silva, K. Lobato and M. J. Brites, J. Photochem. Photobiol., A, 2015, 310, 1–8 CrossRef.
- L. Han, R. Kang, X. Zu, Y. Cui and J. Gao, Photochem. Photobiol. Sci., 2015, 14, 2046–2053 CrossRef CAS PubMed.
- K. C. Avhad, D. S. Patil, Y. K. Gawale, S. Chitrambalam, M. C. Sreenath, I. H. Joe and N. Sekar, ChemistrySelect, 2018, 3, 4393–4405 CrossRef CAS.
- K. D. Seo, I. T. Choi, Y. G. Park, S. Kang, J. Y. Lee and H. K. Kim, Dyes Pigm., 2012, 94, 469–474 CrossRef CAS.
- H. Feng, R. Li, Y. Song, X. Li and B. Liu, J. Power Sources, 2017, 345, 59–66 CrossRef CAS.
- C. W. Song, U. Tamima, Y. J. Reo, M. Dai, S. Sarkar and K. H. Ahn, Dyes Pigm., 2019, 171, 107718 CrossRef CAS.
- N. Jiang, J. Fan, F. Xu, X. Peng, H. Mu, J. Wang and X. Xiong, Angew. Chem., Int. Ed. Engl., 2015, 54, 2510–2514 CrossRef CAS PubMed.
- P. S. Deore and R. A. Manderville, Analyst, 2020, 145, 1288–1293 RSC.
- P. S. Deore, D. S. Coman and R. A. Manderville, Chem. Commun., 2019, 55, 3540–3543 RSC.
- N. Narayanaswamy, M. Kumar, S. Das, R. Sharma, P. K. Samanta, S. K. Pati, S. K. Dhar, T. K. Kundu and T. Govindaraju, Sci. Rep., 2014, 4, 6476 CrossRef CAS PubMed.
- A. Eordogh, J. Steinmeyer, K. Peewasan, U. Schepers, H. A. Wagenknecht and P. Kele, Bioconjugate Chem., 2016, 27, 457–464 CrossRef CAS PubMed.
- J. W. Yan, Y. G. Tian, J. H. Tan and Z. S. Huang, Analyst, 2015, 140, 7146–7149 RSC.
- P. Jana, M. Radhakrishna, S. Khatua and S. Kanvah, Phys. Chem. Chem. Phys., 2018, 20, 13263–13270 RSC.
- S. K. Lanke and N. Sekar, J. Fluoresc., 2016, 26, 497–511 CrossRef CAS PubMed.
- X. D. Liu, Y. Xu, R. Sun, Y. J. Xu, J. M. Lu and J. F. Ge, Analyst, 2013, 138, 6542–6550 RSC.
- S. Zhu, W. Lin and L. Yuan, Dyes Pigm., 2013, 99, 465–471 CrossRef CAS.
- L. Yuan, W. Lin and J. Song, Chem. Commun., 2010, 46, 7930–7932 RSC.
- F. Dubar, C. Slomianny, J. Khalife, D. Dive, H. Kalamou, Y. Guerardel, P. Grellier and C. Biot, Angew. Chem., Int. Ed. Engl., 2013, 52, 7690–7693 CrossRef CAS PubMed.
- Y. Q. Sun, J. Liu, J. Zhang, T. Yang and W. Guo, Chem. Commun., 2013, 49, 2637–2639 RSC.
- Y. Venkatesh, K. S. Kiran, S. S. Shah, A. Chaudhuri, S. Dey and N. D. P. Singh, Org. Biomol. Chem., 2019, 17, 2640–2645 RSC.
- K. A. Pardeshi, G. Ravikumar and H. Chakrapani, Org. Lett., 2018, 20, 4–7 CrossRef CAS PubMed.
- T. Zhang, C. Yin, Y. Zhang, J. Chao, G. Wen and F. Huo, Spectrochim. Acta, Part A, 2020, 234, 118253 CrossRef CAS PubMed.
- W. Xu, C. L. Teoh, J. Peng, D. Su, L. Yuan and Y. T. Chang, Biomaterials, 2015, 56, 1–9 CrossRef CAS PubMed.
- T. Zhang, L. Li, F. Huo, W. Zhang, J. Chao and C. Yin, Sens. Actuators, B, 2021, 342, 130041 CrossRef CAS.
- J. Liu, Y.-Q. Sun, H. Zhang, Y. Huo, Y. Shi, H. Shi and W. Guo, RSC Adv., 2014, 4, 64542–64550 RSC.
- X. Lv, J. Liu, Y. Liu, Y. Zhao, Y. Q. Sun, P. Wang and W. Guo, Chem. Commun., 2011, 47, 12843–12845 RSC.
- M.-J. Peng, Y. Guo, X.-F. Yang, F. Suzenet, J. Li, C.-W. Li and Y.-W. Duan, RSC Adv., 2014, 4, 19077–19085 RSC.
- H. Li, Z. Wen, L. Jin, Y. Kan and B. Yin, Chem. Commun., 2012, 48, 11659–11661 RSC.
- Z. Yang, Z. Liu, Y. Chen, X. Wang, W. He and Y. Lu, Org. Biomol. Chem., 2012, 10, 5073–5076 RSC.
- C. T. Yang, Y. Wang, E. Marutani, T. Ida, X. Ni, S. Xu, W. Chen, H. Zhang, T. Akaike, F. Ichinose and M. Xian, Angew. Chem., Int. Ed. Engl., 2019, 58, 10898–10902 CrossRef CAS PubMed.
- J. Li, Y. Cui, C. Bi, S. Feng, F. Yu, E. Yuan, S. Xu, Z. Hu, Q. Sun, D. Wei and J. Yoon, Anal. Chem., 2019, 91, 7360–7365 CrossRef CAS PubMed.
- H. Fujioka, S. N. Uno, M. Kamiya, R. Kojima, K. Johnsson and Y. Urano, Chem. Commun., 2020, 56, 5617–5620 RSC.
- D. Zhu, X. Yan, A. Ren, W. Xie and Z. Duan, Anal. Chim. Acta, 2019, 1058, 136–145 CrossRef CAS PubMed.
- X. Wu, L. Zeng, B. Q. Chen, M. Zhang, J. Rodrigues, R. Sheng and G. M. Bao, J. Mater. Chem. B, 2019, 7, 5775–5781 RSC.
- S. Singha, D. Kim, B. Roy, S. Sambasivan, H. Moon, A. S. Rao, J. Y. Kim, T. Joo, J. W. Park, Y. M. Rhee, T. Wang, K. H. Kim, Y. H. Shin, J. Jung and K. H. Ahn, Chem. Sci., 2015, 6, 4335–4342 RSC.
- Z. Zhan, R. Liu, L. Chai, Y. Dai and Y. Lv, Anal. Chem., 2019, 91, 11461–11466 CrossRef CAS PubMed.
- H. Huang, W. Liu, X. J. Liu, Y. Q. Kuang and J. H. Jiang, Talanta, 2017, 168, 203–209 CrossRef CAS PubMed.
- Y. J. Reo, S. Sarkar, M. Dai, Y. J. Yang and K. H. Ahn, ACS Appl. Bio Mater., 2021, 4, 2089–2096 CrossRef CAS PubMed.
- Y. J. Yang, M. Dai and K. H. Ahn, ACS Sens., 2023, 8(7), 2791–2798 CrossRef CAS PubMed.
- Z. Yu, W. Huang, S. Xu and S. Ke, Microchem. J., 2021, 164, 106009 CrossRef CAS.
- L. Nie, C. Gao, T. Shen, J. Jing, S. Zhang and X. Zhang, Anal. Chem., 2019, 91, 4451–4456 CrossRef CAS PubMed.
- J. Zhou, C. Yu, Z. Li, P. Peng, D. Zhang, X. Han, H. Tang, Q. Wu, L. Li and W. Huang, Anal. Methods, 2019, 11, 1312–1316 RSC.
- Y. Wang, X. Han, X. Zhang, L. Zhang and L. Chen, Analyst, 2020, 145, 1389–1395 RSC.
- Y. J. Reo, M. Dai, Y. J. Yang and K. H. Ahn, Anal. Chem., 2020, 92, 12678–12685 CrossRef CAS PubMed.
- Y. J. Kim, S. J. Park, C. S. Lim, D. J. Lee, C. K. Noh, K. Lee, S. J. Shin and H. M. Kim, Anal. Chem., 2019, 91, 9246–9250 CrossRef CAS PubMed.
- L. Yuan, W. Lin, Y. Yang and H. Chen, J. Am. Chem. Soc., 2012, 134, 1200–1211 CrossRef CAS PubMed.
- L. Yuan, W. Lin, S. Zhao, W. Gao, B. Chen, L. He and S. Zhu, J. Am. Chem. Soc., 2012, 134, 13510–13513 CrossRef CAS PubMed.
- H. Chen, B. Dong, Y. Tang and W. Lin, Acc. Chem. Res., 2017, 50, 1410–1422 CrossRef CAS PubMed.
- Z. Zeng, S. S. Liew, X. Wei and K. Pu, Angew. Chem., Int. Ed. Engl., 2021, 60, 26454–26475 CrossRef CAS PubMed.
- Y. Wen, N. Jing, F. Huo and C. Yin, Analyst, 2021, 146, 7450–7463 RSC.
- M. E. Jun, B. Roy and K. H. Ahn, Chem. Commun., 2011, 47, 7583–7601 RSC.
- S. Singha, Y. W. Jun, S. Sarkar and K. H. Ahn, Acc. Chem. Res., 2019, 52, 2571–2581 CrossRef CAS PubMed.
- H. H. Han, H. Tian, Y. Zang, A. C. Sedgwick, J. Li, J. L. Sessler, X. P. He and T. D. James, Chem. Soc. Rev., 2021, 50, 9391–9429 RSC.
|
This journal is © The Royal Society of Chemistry 2023 |
Click here to see how this site uses Cookies. View our privacy policy here.