DOI:
10.1039/D2FO02979K
(Paper)
Food Funct., 2023,
14, 262-276
The chemical profiling and assessment of antioxidative, antidiabetic and antineurodegenerative potential of Kombucha fermented Camellia sinensis, Coffea arabica and Ganoderma lucidum extracts†
Received
5th October 2022
, Accepted 2nd December 2022
First published on 2nd December 2022
Abstract
The scientific interest in the medicinal properties of Kombucha beverages, a carbonated drink with live microorganisms, has increased recently. Hence, the aim of this study was to determine the chemical profile and to examine the antioxidant, antidiabetic and antineurodegenerative potential of unfermented and also Kombucha fermented Camellia sinensis (green tea), Coffea arabica (coffee), and Ganoderma lucidum (Reishi) extracts. The extracts were prepared as follows: the first (unfermented) set contained 1 L of water, 50 g of sucrose and 20 g of dried and ground green tea, coffee, or Reishi basidiocarp, while the second (fermented) set contained all of the aforementioned ingredients individually inoculated with Kombucha and fermented for 21 days. The chemical analysis was conducted using liquid chromatography–mass spectrometry (LC-MS). The antioxidant activity was assessed by DPPH, total reducing power (TRP), and β-carotene bleaching assays. The inhibition of α-amylase and α-glucosidase activity was used to estimate the antidiabetic potential, while the level of inhibition of acetylcholinesterase (AChE) and tyrosinase (TYR) was used to evaluate the antineurodegenerative activity. The results suggested that the fermented extracts of green tea, coffee, and Reishi exert significant antioxidant effects, although they were lower compared to the unfermented extracts. The unfermented green tea extract exhibited the highest DPPH-scavenging activity (87.46%) and the highest preservation of β-carotene (92.41%), while the fermented coffee extract showed the highest TRP (120.14 mg AAE per g) at 10 mg mL−1. Although the extracts did not inhibit the activity of α-amylase, they were quite effective at inhibiting α-glucosidase, especially the unfermented Reishi extract, inhibiting 95.16% (at a concentration of 10 mg mL−1) of α-glucosidase activity, which was slightly higher than the positive control at the same concentration. The most effective AChE inhibitor was unfermented green tea extract (68.51%), while the fermented coffee extract inhibited 34.66% of TYR activity at 10 mg mL−1. Altogether, these results are in accordance with the differences found in the extracts’ chemical composition. Finally, this is the first report that highlights the differences in the chemical profile between the unfermented and Kombucha fermented green tea, coffee and Reishi extracts, while it also reveals, for the first time, the antineurodegenerative potential of Kombucha fermented Reishi extract. The examined extracts represent potent functional foods, while their more detailed mechanisms of action are expected to be revealed in future research.
Introduction
The metabolism of aerobic organisms includes a series of reactions in mitochondria and peroxisomes that, in addition to providing the necessary energy in the form of ATP, also lead to the formation of reactive oxygen species (ROS), which include free radicals and other reactive species (O−, OH−, and H2O2).1 Besides the mentioned endogenous ROS, created as an intermediate product of biochemical reactions, the influence of exogenous triggers of ROS formation such as diet, poor lifestyle, ionizing radiation, toxins, and heavy metals is also significant. As a response to the constant presence of ROS, organisms have developed different antioxidant defense mechanisms that neutralize ROS, and as different tissues and organs are characterized by different metabolic activities and oxygen consumption, they are also characterized by different levels of antioxidants.2 When the production of ROS exceeds the capacity of a cell's antioxidant mechanisms, they accumulate causing oxidative stress. At this point the help of exogenous antioxidants is necessary.1 Due to their high reactivity, ROS can interact with any molecule in the body and induce damage on proteins, DNA, and cell membranes, leading to numerous disorders and diseases such as diabetes, neurodegenerative diseases, and even cancer.3
Diabetes is a metabolic disorder that affects an increasing world population and the key factor in its development is oxidative stress, which influences insulin production and cell sensitivity to it.4 Uncontrolled diabetes can lead to a reduced cell growth and division, reduced protein synthesis, as well as various microvascular complications, such as neuropathy, and also macrovascular complications, such as cardiovascular disease and stroke.5 Blood glucose levels can be controlled by physical activity, a balanced diet, or medications, which have been shown to have various side effects.6,7 Considering that α-amylase and α-glucosidase hydrolyse carbohydrates, which increase the level of sugar in the blood, their excessive activity leads to the development of a pathological condition. Bearing this in mind, natural inhibitors of these enzymes represent great potential for developing effective drugs against diabetes.8
The advancement of medicine and the improvement of living conditions in developed parts of the world have led to a rising proportion of elderly people in the general population, but unfortunately also to an increasing presence of various neurodegenerative diseases, primarily Alzheimer's and Parkinson's. Thus, every third elderly person dies with some form of dementia, and currently in the United States alone, 6.2 million people over the age of 65 years suffer from Alzheimer's disease as the main cause of dementia. Parkinson's disease is the second most important neurodegenerative disease that affects an increasing population of the elderly, from about 4 million in 2005 to about 10 million sufferers worldwide at the moment.9,10 Genetic and numerous exogenous factors are intertwined in the pathogenesis of neurodegenerative diseases. It has been proven that oxidative stress can induce a change in the production of acetylcholine through enhanced activity of acetylcholinesterase (AChE) as the trigger of Alzheimer's disease, and also increased activity of tyrosinase (TYR), which leads to the onset of Parkinson's disease.11 Since the effects of oxidative stress, such as inflammation, are also known to be the cause of diabetes, it is surprising that patients with diabetes have a greater incidence of neurodegeneration, mainly Alzheimer's disease.12
The most commonly used synthetic antioxidants and conventional drugs used in the therapy of diabetes and neurodegenerative disorders can exert a number of harmful effects and also usually do not stop the progression of the disease, but only slow it down or temporarily alleviate the symptoms. Bearing this in mind, scientists are aiming to find alternative natural sources of bioactive compounds such as mushrooms and plants.13–15 Many studies have shown that plants and mushrooms possess bioactive compounds with antitumor, antidiabetic, antineurodegenerative, anti-inflammatory, antimicrobial, antiviral and other medicinal properties.5,16,17
Kombucha, also known as the Manchurian mushroom, represents a symbiotic culture of bacteria and yeasts.18 Kombucha tea is a slightly sour and sweet, carbonated refreshing beverage obtained by the fermentation of sweetened green or black tea leaves with a symbiotic association of fungi and acetic acid bacteria (most often fungi from the following genera: Schizosaccharomyces, Zygosaccharomyces, Candida, Torulospora, Pichia, Mycotorula, and Mycoderma, and bacteria: Acetobacter and Gluconobacter). Sucrose is mostly used as a tea sweetener, and Kombucha's invertases hydrolyze it into glucose and fructose, which are further used as carbon sources for the symbiotic community, in contrast to milk fermentation when lactose is used as a carbon source, which significantly affects the taste and chemical composition of the fermented beverage. In contrast to lactic acid fermentation, acetic acid and ethanol are dominantly produced during the oxidative fermentation of green tea by the Kombucha consortium, so fermented tea gains flavour characteristics and an increased content of certain bioactive compounds enhancing the bioactive potential of the fermented beverage.19,20 The positive effect of the fermentation type on sensory quality and bioactivity was also reported for other plant-based fermented drinks.21,22 Due to its medicinal properties, Kombucha tea is attracting more and more attention from consumers and researchers.23,24 This drink was traditionally used in the Far East, especially in China and Japan, for treating a plethora of diseases, while modern research has confirmed its numerous bioactivities and given it a scientific basis.19,25 Thus, it has been demonstrated that it promotes digestion, has a laxative effect, relieves arthritis, shows antimicrobial, anti-stress and anti-cancer effects, and increases the overall vitality.26 It is important to bear in mind that most of these benefits are based on its antioxidant potential, primarily thanks to the high content of antioxidants in green tea, such as polyphenols, vitamins (C, B2, and B6), citric acid, etc. In addition to green tea, as a basis for the production of Kombucha drink, other herbs can be used, as well as numerous dairy products, fruits and vegetables, whose fermentation produces drinks with high biological and pharmaceutical potential.18,20–22,27–29
Although numerous studies have confirmed that many plants and mushrooms are rich sources of bioactive substances, only a small number of plants and mushrooms have been examined as a basis for the preparation of Kombucha drink. This fact inspired us to set the goals of this research, including the determination of the chemical composition as well as examination and comparison of the antioxidant, antidiabetic, and antineurodegenerative properties of unfermented and Kombucha-fermented extracts of three widely known and used species – Camellia sinensis (green tea), Coffea arabica (coffee), and Ganoderma lucidum (Reishi).
Results
Chemical compositions of the extracts
Two major groups of phenolic compounds, namely phenolic acids and flavonoids, were determined in green tea, coffee, and Reishi unfermented and fermented extracts using LC-MS analysis (Table 1). Altogether, the most prominent phenolic acid was chlorogenic acid, especially in fermented and unfermented coffee extracts (43.52 and 41.33 mg L−1, respectively), while rutin was found to be the most abundant flavonoid, particularly in fermented and unfermented green tea extracts (3.86 and 4.54 mg L−1, respectively). The results suggested that there were more or less noticeable differences between the chemical composition of the unfermented and fermented extracts of the same taxa. For instance, the fermented Reishi extract contained larger amounts of protocatechuic, syringic, chlorogenic (which was the predominant compound found in this extract, 2.31 mg L−1), caffeic and p-coumaric acids, and aesculetin, compared to the respective unfermented extract. On the other hand, its unfermented extract contained a higher amount of p-hydroxybenzoic acid compared to the fermented extract. Differences in the chemical composition were also noticed for green tea extracts: the unfermented one contained a larger amount of protocatechuic, syringic and chlorogenic acids, rutin, vitexin, isoquercetin, quercitrin, astragalin, quercetin, and kaempferol compared to its fermented extract. The same was observed for the two coffee extracts: the fermented extract consisted of higher amounts of protocatechuic, syringic, chlorogenic, caffeic, p-hydroxybenzoic and p-coumaric acids, and rutin, vitexin, isoquercetin, and aesculetin. Finally, it is worth mentioning that quercetin and kaempferol were detected only in green tea extracts, while they were not found in coffee and Reishi extracts.
Table 1 LC-MS analysis of the tested extracts
LC-MS analysis (mg L−1) |
Constituents |
Fermented green tea |
Unfermented green tea |
Fermented coffee |
Unfermented coffee |
Fermented Reishi |
Unfermented Reishi |
Values are presented as means ± standard deviations (n = 3). Values for each constituent among different extracts (in the same row) superscripted with different letters (a–d) differ significantly (p < 0.01) according to Tukey's test (ND – not detected). |
Protocatechuic acid |
0.30 ± 0.03b |
2.93 ± 0.32d |
1.72 ± 0.17c |
0.59 ± 0.06b |
0.10 ± 0.01a |
0.08 ± 0.01a |
Syringic acid |
0.37 ± 0.02b |
3.11 ± 0.14d |
2.09 ± 0.10d |
1.21 ± 0.06c |
0.14 ± 0.01a |
0.07 ± 0.00a |
Chlorogenic acid |
0.22 ± 0.01a |
0.47 ± 0.03b |
43.52 ± 2.33d |
41.33 ± 2.22d |
2.31 ± 0.12c |
0.38 ± 0.02b |
Caffeic acid |
0.34 ± 0.01c |
0.16 ± 0.00b |
6.88 ± 0.14d |
5.36 ± 0.11d |
0.26 ± 0.01c |
0.02 ± 0.00a |
p-Hydroxybenzoic acid |
1.69 ± 0.26c |
1.11 ± 0.17c |
1.69 ± 0.26c |
0.28 ± 0.04a |
0.33 ± 0.05a |
0.51 ± 0.08b |
p-Coumaric acid |
0.11 ± 0.00b |
0.11 ± 0.00b |
0.16 ± 0.00b |
0.02 ± 0.00a |
0.05 ± 0.00a |
ND |
Aesculetin |
0.15 ± 0.00a |
0.10 ± 0.00a |
3.40 ± 0.04b |
2.73 ± 0.03b |
0.14 ± 0.00a |
ND |
Rutin |
3.86 ± 0.15c |
4.54 ± 0.18c |
0.15 ± 0.01b |
0.01 ± 0.00a |
0.01 ± 0.00a |
ND |
Vitexin |
0.95 ± 0.02b |
1.14 ± 0.03b |
0.04 ± 0.00a |
0.01 ± 0.00a |
ND |
ND |
Isoquercetin |
1.68 ± 0.08c |
2.08 ± 0.10c |
0.08 ± 0.00b |
0.03 ± 0.00a |
0.02 ± 0.00a |
0.02 ± 0.00a |
Quercitrin |
0.11 ± 0.00b |
0.33 ± 0.01c |
0.03 ± 0.00a |
0.04 ± 0.00a |
0.02 ± 0.00a |
0.02 ± 0.00a |
Astragalin |
1.14 ± 0.01b |
1.46 ± 0.02b |
0.03 ± 0.00a |
0.02 ± 0.00a |
0.02 ± 0.00a |
0.03 ± 0.00a |
Quercetin |
0.38 ± 0.01a |
0.82 ± 0.01b |
ND |
ND |
ND |
ND |
Kaempferol |
0.09 ± 0.00a |
0.11 ± 0.01a |
ND |
ND |
ND |
ND |
The chromatograms are shown in ESI Fig. 1.†
Antioxidative activity
Neutralization of DPPH radicals.
The obtained results showed different antioxidative activities for both fermented and unfermented extracts, which also varied to some extents due to synthetic antioxidants. Generally, with an increase in the concentration of extracts from 0.25 to 10 mg mL−1, their antioxidant activity also enhanced (Fig. 1a). Thus, unfermented green tea extract at a concentration of 10 mg mL−1 proved to be the most effective neutralizer of DPPH radicals (87.46%), followed by the unfermented coffee extract, which at the same concentration, inhibited 80.57% of DPPH radicals. On the other hand, at the same concentration, fermented green tea and coffee extracts showed a significantly lower ability to reduce DPPH˙ (about 70% and 60%, respectively) compared to the respective unfermented extracts. Furthermore, both unfermented and fermented Reishi extracts exhibited significantly lower antioxidant potential at all tested concentrations compared to green tea and coffee extracts, neutralizing less than 10% of DPPH˙ at the highest concentration. As expected, the level of antioxidant activity of the positive controls, BHA, BHT, and ascorbic acid, was already high at 0.5 mg mL−1 (over 70%), while at a concentration of 2.0 mg mL−1, they reduced even 90% of DPPH radicals. These differences in activities compared to the standards at lower concentrations were reflected on the obtained IC50 values, which were many times lower for ascorbic acid (0.03 mg mL−1, R2 0.99), BHA and BHT (∼0.20 mg mL−1, R2 0.99) compared to the most active extract (4.85 mg mL−1, R2 0.97) (Fig. 1a).
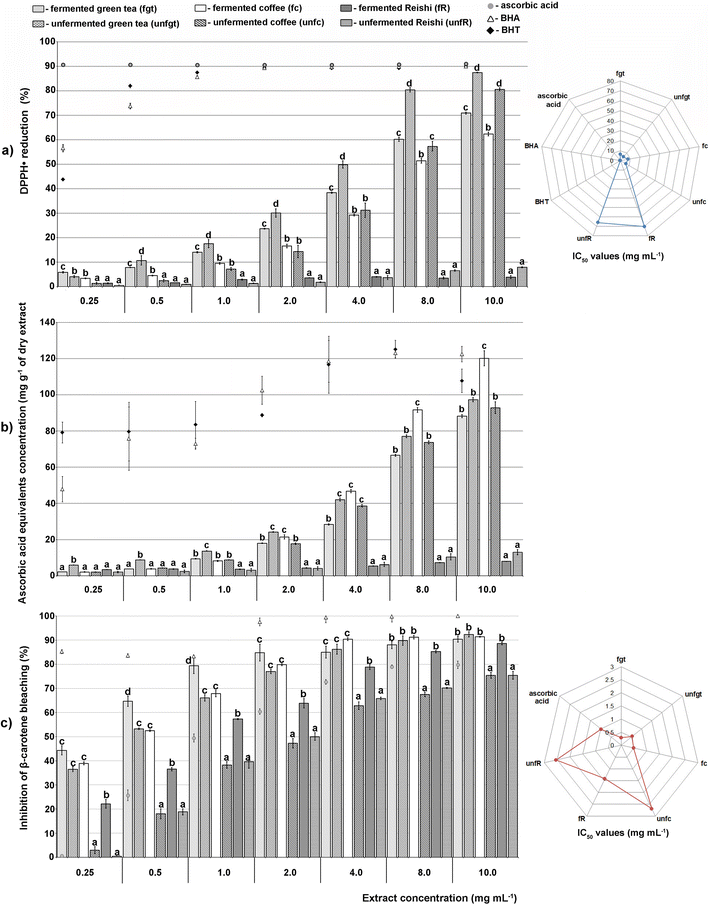 |
| Fig. 1 Antioxidative capacity of green tea, coffee and Reishi extracts. (The values labelled with different letters (a–c) in each concentration group differ significantly (p < 0.01) according to Tukey's test.) | |
Total reducing potential.
The TRP of green tea, coffee and Reishi extracts positively correlated with their applied concentration (Fig. 1b). In contrast to the results of the DPPH assay, the maximum reduction potential of Fe3+ to Fe2+ was shown by the fermented coffee extract (120.14 mg AAE per g). A slightly lower value was measured for unfermented green tea (97.33 mg AAE per g), while unfermented coffee and fermented green tea showed similar TRP (∼90 mg AAE per g). On the other hand, Reishi extracts showed a much lower activity, just 13.04 mg AAE per g for the unfermented, and 8 mg AAE per g for the fermented extract.
β-Carotene bleaching inhibition.
The antioxidant activity of the studied extracts, measured through the inhibition of β-carotene color loss, was high even at lower concentrations (Fig. 1c). At a concentration of 1 mg mL−1, the fermented green tea extract showed an inhibition of 79.44%. Furthermore, at a concentration of 10 mg mL−1, its activity increased to 90.41%, while a statistically insignificant higher activity of 92.41% was measured for the unfermented green tea extract. The fermented extracts of coffee and Reishi at the same concentration also significantly inhibited the loss of β-carotene color (91.41% and 88.62%, respectively), which was about 15% more than for the respective unfermented extracts. Interestingly, the antioxidant activity of the positive control, ascorbic acid, at all tested concentrations was lower compared to that of the green tea and coffee extracts, having a higher IC50 value (0.98 mg mL−1, R2 0.96) than the green tea and fermented coffee extracts. On the other hand, the activity of BHA reached its plateau at lower concentrations and did not change significantly with further concentration increase, being just slightly higher than the studied extracts at the highest concentration (Fig. 1c).
According to our IBR analysis, (ESI Fig. 2† and Fig. 2) which included the results of the employed antioxidant assays (DPPH, TRP and β-carotene bleaching assays), the most effective antioxidant agent among the tested extracts was the unfermented green tea, followed by the fermented coffee extract. The fermented Reishi extract was found to be the least effective antioxidant agent.
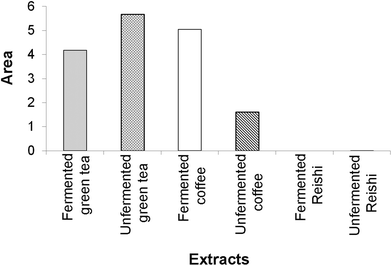 |
| Fig. 2 The area of the radar charts shown in ESI Fig. 2.† | |
In Fig. 2, a larger area indicates greater antioxidant potential of the tested extracts.
Antidiabetic activity
Inhibition of α-amylase.
In most cases, the studied extracts did not inhibit the activity of α-amylase or their activities were negligibly low, in contrast to the positive control, acarbose, whose activity ranged from 57.21 to 97.13% depending on the concentration used (IC50 0.11 mg mL−1, R2 0.81).
Inhibition of α-glucosidase.
Although a maximum of α-glucosidase inhibition (95.16%) was observed for the unfermented Reishi extract (at 10 mg mL−1), the unfermented green tea extract also showed a significantly higher capacity to inhibit this enzyme at all other tested concentrations (Fig. 3). In particular, at 2 mg mL−1, this extract inhibited 88.77% of α-glucosidase activity, reaching a plateau of about 90% with increasing concentration, which was reflected on its IC50 value (0.61 mg mL−1, R2 0.99) (Tables 2 and 3). The green tea fermented extract showed lower inhibition potential than the unfermented one at lower concentrations. On the other hand, the fermented Reishi extract, at all concentrations except for 10 mg mL−1, showed significantly higher potential for inhibiting the activity of this enzyme compared to the unfermented one, confirmed by an approximately two fold lower IC50 value (Tables 2 and 3). The fermented extracts of green tea and Reishi also showed exceptional antidiabetic potential at the highest concentration, inhibiting as much as 79.86% and 75.67%, respectively, of α-glucosidase activity. The activity of green tea and Reishi extracts differed from both unfermented and fermented coffee extracts, whose effects were significantly lower. However, although it showed poor α-glucosidase inhibition, it should be emphasized that the fermented coffee extract was two-fold more active than its unfermented extract, which at lower concentrations did not even show the ability to inhibit the activity of this enzyme. The inhibition of α-glucosidase by acarbose was only slightly higher than the activity of the unfermented green tea at higher concentrations due to the fact that it reached its plateau at 4 mg mL−1, which affected its IC50 value (obtained from a wider concentration range, 0.24 mg mL−1, R2 0.91) being lower than for unfermented green tea. However, it is worth mentioning that the maximal activity of acarbose at 10 mg mL−1 was still slightly lower (92.93%) than that measured for the Reishi extract.
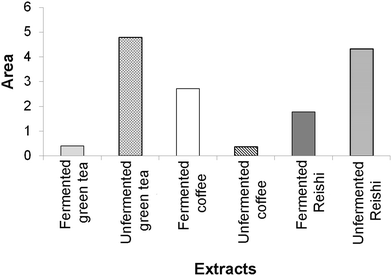 |
| Fig. 3 The area of the radar charts shown in ESI Fig. 3.† | |
Table 2 Antidiabetic and antineurodegenerative capacity of green tea, coffee and Reishi extracts
Tested extracts and standard |
Extracts and standard concentration (mg mL−1) |
α-Glucosidase inhibition (%) |
Acetylcholinesterase inhibition (%) |
Tyrosinase inhibition (%) |
*Values are presented as means ± standard errors (n = 3). The values in the same concentration group superscripted with different letters (a–f) differ significantly (p < 0.01) according to Tukey's test. |
Fermented green tea |
0.25 |
7.47 ± 0.78a |
0.00 ± 0.00 |
13.07 ± 0.27b |
0.5 |
15.18 ± 2.10c |
0.00 ± 0.00 |
16.80 ± 0.80c |
1 |
36.51 ± 0.94b |
0.00 ± 0.00 |
19.73 ± 0.71c |
2 |
48.79 ± 0.84b |
7.07 ± 0.80b |
20.53 ± 1.07b |
4 |
60.72 ± 0.76c |
11.48 ± 1.18b |
21.07 ± 0.53a |
8 |
78.64 ± 0.75b |
17.24 ± 0.21b |
23.20 ± 0.46a |
10 |
79.87 ± 0.71c |
21.65 ± 1.22b |
25.07 ± 1.16a |
|
Unfermented green tea |
0.25 |
24.18 ± 1.17c |
55.71 ± 1.33c |
0.00 ± 0.00 |
0.5 |
43.90 ± 2.80d |
56.83 ± 0.04c |
13.60 ± 1.22b |
1 |
75.30 ± 1.45c |
57.11 ± 0.45c |
19.20 ± 1.60c |
2 |
88.77 ± 0.04c |
58.35 ± 0.39e |
20.53 ± 0.96b |
4 |
88.46 ± 0.86d |
62.24 ± 2.39f |
21.60 ± 1.22a |
8 |
89.33 ± 0.20c |
65.86 ± 2.42e |
25.33 ± 1.92b |
10 |
90.56 ± 0.23d |
68.51 ± 3.42d |
28.27 ± 1.48b |
|
Fermented coffee |
0.25 |
2.61 ± 0.02a |
8.36 ± 0.44a |
0.00 ± 0.00 |
0.5 |
9.14 ± 0.72b |
19.76 ± 0.06a |
0.00 ± 0.00 |
1 |
7.21 ± 0.22a |
20.33 ± 0.17a |
17.07 ± 0.96b |
2 |
7.80 ± 0.47a |
21.74 ± 0.78c |
27.20 ± 0.46d |
4 |
11.47 ± 0.25a |
37.04 ± 0.90d |
29.33 ± 1.87c |
8 |
17.25 ± 0.30a |
39.15 ± 1.48c |
33.33 ± 0.71c |
10 |
15.33 ± 0.62b |
45.05 ± 3.46c |
34.67 ± 1.41c |
|
Unfermented coffee |
0.25 |
0.00 ± 0.00 |
0.00 ± 0.00 |
0.00 ± 0.00 |
0.5 |
0.00 ± 0.00 |
0.00 ± 0.00 |
2.40 ± 0.46a |
1 |
0.00 ± 0.00 |
0.00 ± 0.00 |
14.93 ± 0.53a |
2 |
0.00 ± 0.00 |
7.30 ± 0.92b |
21.33 ± 0.27b |
4 |
0.00 ± 0.00 |
19.97 ± 2.20c |
25.60 ± 0.46b |
8 |
0.00 ± 0.00 |
23.74 ± 1.78b |
26.93 ± 0.71b |
10 |
7.90 ± 3.93a |
26.31 ± 1.19b |
28.80 ± 0.46b |
|
Fermented Reishi |
0.25 |
11.81 ± 0.88b |
37.82 ± 1.29b |
10.40 ± 0.46a |
0.5 |
14.82 ± 2.99c |
43.05 ± 2.17b |
16.27 ± 0.71c |
1 |
35.10 ± 0.85b |
45.87 ± 0.26b |
17.87 ± 0.71b |
2 |
45.69 ± 1.48b |
47.31 ± 0.73d |
18.13 ± 0.53a |
4 |
60.42 ± 2.47c |
49.56 ± 1.93e |
20.00 ± 0.46a |
8 |
74.12 ± 1.49b |
50.11 ± 0.84d |
21.60 ± 0.46a |
10 |
75.67 ± 1.43c |
52.91 ± 0.87c |
23.20 ± 0.92a |
|
Unfermented Reishi |
0.25 |
0.00 ± 0.00 |
0.00 ± 0.00 |
14.40 ± 2.12b |
0.5 |
1.93 ± 0.76a |
0.00 ± 0.00 |
16.53 ± 0.27c |
1 |
8.64 ± 1.49a |
0.00 ± 0.00 |
21.33 ± 0.53c |
2 |
9.01 ± 0.38a |
2.02 ± 0.37a |
24.00 ± 0.46c |
4 |
34.76 ± 1.61b |
4.23 ± 0.00a |
25.60 ± 0.80b |
8 |
69.44 ± 6.49b |
7.49 ± 1.28a |
26.67 ± 0.71b |
10 |
95.16 ± 0.35d |
15.44 ± 2.67a |
27.47 ± 0.71b |
Table 3 Green tea, coffee and Reishi extracts and positive controls against α-glucosidase, acetylcholinesterase and tyrosinase activities expressed through half-maximal inhibitory concentrations
Studied extracts and standards |
α-Glucosidase activity assay |
Acetylcholinesterase activity assay |
Tyrosinase activity assay |
IC50 (mg mL−1) |
R
2
|
IC50 (mg mL−1) |
R
2
|
IC50 (mg mL−1) |
R
2
|
*Values are presented as means ± standard errors (n = 3). IC50 values in the same column superscripted with different letters (a–e) differ significantly (p < 0.01) according to Tukey's test; ND – the value was not detected in the tested concentration range. |
Fermented green tea |
2.76 ± 0.07c |
0.84 |
22.31 ± 0.08d |
0.96 |
33.20 ± 1.01d |
0.89 |
Unfermented green tea |
0.61 ± 0.02b |
0.99 |
ND |
|
24.51 ± 0.98c |
0.96 |
Fermented coffee |
26.73 ± 0.11e |
0.95 |
11.92 ± 0.06c |
0.99 |
13.27 ± 0.72b |
0.87 |
Unfermented coffee |
ND |
|
17.09 ± 0.09c |
0.89 |
16.36 ± 0.54b |
0.98 |
Fermented Reishi |
2.84c |
0.89 |
6.76 ± 0.02b |
0.70 |
34.17 ± 1.23d |
0.76 |
Unfermented Reishi |
5.63d |
0.99 |
36.13 ± 0.10e |
0.93 |
25.67 ± 0.87c |
0.79 |
Acarbose |
0.24 ± 0.02a |
0.91 |
|
|
|
|
Galantamine |
|
|
0.12 ± 0.10a |
0.99 |
|
|
Kojic acid |
|
|
|
|
0.11 ± 0.01a |
0.95 |
Antineurodegenerative activity
Acetylcholinesterase inhibition.
Compared with galantamine, serving as a positive control, the studied extracts proved to be moderate AchE inhibitors (Table 2). In particular, the most effective antineurodegenerative agent was the unfermented green tea extract, which even at the lowest concentration inhibited 55.71% of AChE activity while at the highest concentration, it inhibited 68.51% of AChE activity, which was 24% lower compared to galantamine, but 46% higher compared to the fermented extract. Thus, the half-maximal inhibitory concentration of unfermented green tea could not be derived from the tested concentrations and compared with the standard's IC50 value (0.12 mg mL−1, R2 0.99) (Table 3). On the other hand, the fermented extracts of Reishi and coffee showed significantly higher activity compared to their unfermented extracts. Thus, at 10 mg mL−1, the fermented Reishi extract inhibited AChE by 52.90%, which was more than three times higher activity compared to its unfermented extract. Moreover, the fermented coffee extract was almost twice as effective as its unfermented extract (Table 2).
Tyrosinase inhibition.
The tested extracts showed weak to moderate potential for TYR inhibition, which was positively correlated with their concentrations (Tables 2 and 3). The fermented coffee extract was shown to be the most promising TYR inhibitor among the tested extracts, exhibiting a maximum activity of 34.66% at 10 mg mL−1 (IC50 13.27 mg mL−1, R2 0.87). Slightly lower values were recorded for the unfermented extracts of coffee (28.8%), green tea (28.26%) and Reishi (27.46%), but their IC50 values were considerably lower than those of kojic acid (Tables 2 and 3).
The results of the enzyme-inhibitory activity (α-amylase, α-glucosidase, AChE, and TYR) assays obtained in our study for the tested unfermented and fermented extracts were also subjected to IBR analysis (ESI Fig. 3† and Fig. 3). As a result of this analysis, it was found that the most active sample was the unfermented green tea extract, followed by the unfermented Reishi extract. The lowest overall enzyme-inhibitory activity was detected for the unfermented coffee extract.
In Fig. 3, a larger area indicates greater enzyme-inhibitory potential of the tested extracts.
The final results of the IBR analysis that included all seven assays employed for the testing of the biological activity of the unfermented and fermented extracts revealed that the unfermented green tea extract, followed by the fermented coffee extract, had the highest biological potential. On the other hand, the lowest biological potential was shown by both Reishi extracts, especially the fermented one (ESI Fig. 4† and Fig. 4).
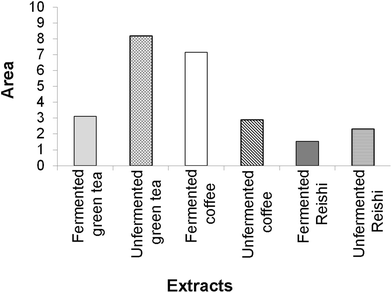 |
| Fig. 4 The area of the radar charts shown in ESI Fig. 3.† | |
A larger area in Fig. 4 indicates greater overall biological potential of the tested extracts.
Discussion
Man's search for therapeutic agents of natural origin dates back to the ancient times, as revealed by several pieces of evidence such as written documents, monuments, and even preserved original herbal drugs. Therefore, it can be argued that the evolution of raising awareness about the use of natural remedies is not only the result of century-old struggle for survival, but also the struggle against diseases. The development of new scientific knowledge about medicinal plants and mushrooms, as well as the development of awareness about the use of natural foods, has increased the interest of researchers to respond to numerous challenges with the aim of improving the quality of life of people in the modern world.30 The latter is additionally justified by the fact that plants and mushrooms produce numerous compounds with positive effects on a plethora of disorders and diseases in humans, while on the other hand, synthetic drugs achieve their pharmacological effects in a more directed manner, often exhibiting numerous side effects.31 Thanks to this, the traditional use of plants and mushrooms for medicinal purposes has over time become a part of modern pharmacotherapy.
Among the pharmacologically and biologically active metabolites produced by plants and mushrooms are phenolic compounds that are known to possess great potential to alleviate the damaging effects caused by oxidative stress by promoting the protective effects of antioxidant agents.32,33 Among the most common groups of phenolic compounds are phenolic acids, flavonoids, coumarins, and tannins.34 Although the content of phenolic compounds in both plants and mushrooms is highly dependent on a number of factors such as phenophase and geographical factors, their preparation also plays an important role in the final detection of their chemical profile. Bearing this in mind, beverages that people consume regularly, such as green tea and coffee, are being intensively investigated since they possess multiple benefits for our general health and reduce the risk of developing severe diseases.33
Fermentation represents a bioprocess often applied to obtain bioactive compounds, mainly in the food industry; however, nowadays it is also used to a greater extent in the cosmetic industry for boosting the quality of phytochemicals and expediting their absorption by the human organism. Interestingly enough, extracts obtained by fermentation are rich in metabolites with antioxidant potential, such as vitamins, minerals, proteins, fibers, probiotics, and secondary metabolites.18,21,22
It has been reported that unfermented green tea phenolic compounds are mainly comprised of flavanols (catechin derivatives), flavonols, and phenolic acids to a lower extent,34 which has also been proven in our research (Table 1). Moreover, Malbaša et al.35 reported similar findings in Kombucha fermented green tea, highlighting the presence of flavonoids, predominantly of catechin and epicatechin (30–42%), while phenolic acids were found at a rather lower concentration. On the other hand, while analyzing the unfermented and Kombucha fermented black tea, Ivanišová et al.36 have shown that gallic, chlorogenic, syringic, and protocatechuic acids, and rutin and vitexin were dominant in the Kombucha beverage, which is in line with our results for the fermented green tea (Table 1).
Although Zofia et al.18 reported that the unfermented green coffee extract has a higher overall phenolic and flavonoid content compared to the fermented extract, our LC-MS analysis actually showed opposite results (Table 1). Moreover, the authors reported that the predominant phenolic acids in these extracts were chlorogenic acid and its derivatives, which is in line with the results presented in Table 1 and with the work of Williamson et al.,37 which also reported that phenolic acids were the main phenolic compounds in coffee extracts.
Oludemi et al.38 investigated the phenolic profile of Reishi extracts and revealed that phenolic acids, primarily protocatechuic, p-hydroxybenzoic and syringic acids, were the most prominent phenolic compounds. These results are in agreement with ours since our LC-MS analysis revealed that Reishi extracts possess higher concentrations of phenolic acids (predominantly chlorogenic acid in the fermented extract, and p-hydroxybenzoic in the unfermented one) compared to flavonoids. To the best of our knowledge, there are no previous reports on the chemical composition of Kombucha fermented Reishi extracts.
Manian et al.39 reported that the green tea extracts showed an exceptional activity of as much as 80% at a concentration of 37.5 μg. Ivanišová et al.36 have shown by the ABTS scavenging assay, whose results strongly correlate with the DPPH assay, that the Kombucha fermented black tea beverage has higher antioxidant potential compared to the unfermented extract (1.16 via 0.67 mg TEAC per mL), which the authors also confirmed by testing the reduction potential of both extracts (2.04 via 0.81 mg TEAC per mL). These results are not in agreement with the ones we obtained since our unfermented green tea extract exerted a higher antioxidant capacity compared to the fermented green tea extract. However, it is commonly known that green tea possesses great antioxidant potential, while its phenolic compounds, especially phenolic acids and flavonols, are proven to enhance its antioxidant status and reduce the undesirable changes of oxidative reactions,33 which is also in agreement with the results presented in Fig. 1a–c.
Coffee extract with a recorded activity of 80–90% also showed an effective reduction of DPPH radicals.40 Although the literature data suggest that the Kombucha fermentation process has a significant influence on the percentage of free radicals scavenged compared with the unfermented extracts,18 our research showed opposite results. Nevertheless, Zofia et al.18 obtained similar results to ours when they varied the extracts’ preparation protocol and subjected the coffee extract to a shorter fermentation period of 7 days, indicating that there was also a variation between the content of active compounds, such as phenolic acids and flavonoids.
In previous studies, various extracts of Reishi showed a significantly higher degree of DPPH radical reduction compared to our results. For instance, Xiao Ping et al.41 proved Reishi to be an excellent neutralizer of DPPH radicals (90%) at a concentration of 2.5 mg mL−1, while Sknepnek et al.42 also confirmed that Kombucha fermentation on a Reishi mushroom substrate significantly enhanced its antioxidant activity. However, this was not confirmed in our study.
The discrepancy observed between previous studies and our results might be explained through the application of different extracts’ preparation protocols. In particular, it has been shown that enzymes discharged by bacteria and yeasts during prolonged Kombucha fermentation may end up in an increased effectiveness for scavenging nitrogen and superoxide radicals, however, also in a decreased ability for scavenging hydroxyl radicals.18 However, the performed antioxidant assays were validated through the similarity of half-maximal inhibitory concentrations obtained in some previous studies.43
Oxidative stress, which increases the production of ROS, plays a key role in the pathogenesis of diabetes, while the main molecular mechanisms involved in the development of this disease are related to glucose and lipid metabolisms. Under hyperglycemia, excessive production of ROS is observed during glycolysis, which further leads to DNA damage and subsequent activation of DNA repair enzymes. Also, elevated levels of ROS lead to oxidative damage of pancreatic β-cells, which affects the quantity and quality of insulin secretion. Therefore, it is necessary to identify and take into account the main biochemical markers of free radical reactions, and the relationship between oxidative stress reactions and inflammation, which represents an additional criterion for monitoring disease progression.44
Given that all of the tested extracts have displayed antioxidant potential, it is not surprising that a number of previous studies have also confirmed the antidiabetic activity of unfermented extracts of green tea, coffee and Reishi. Actually, many phenolic compounds are known inhibitors of enzymes key to the metabolism of carbohydrates in the body, with the ability to modulate their digestion, and thanks to them, the glycemic index of certain food products can be controlled.45 In particular, Duangjai et al.46 showed that phenolic acids isolated from coffee extract inhibited 49% of α-amylase activity, while a similar value (52.26%) was observed by Nickavar and Yousefian47 for green tea extract at a concentration of 2.304 mg mL−1. Although the coffee extract showed a low degree of α-amylase inhibition in both our study and the study of Zengin et al.,48 the results of Duangjai et al.46 indicated moderate inhibition with an activity of up to 46.57%. Furthermore, the α-amylase and α-glucosidase activities were also confirmed earlier for the fermented green tea and coffee extracts.49,50 Overall, the starch hydrolase inhibitory properties of the fermented beverages might be an indication of their potential to act as functional foods due to their beneficial impact on the maintenance of human health and wellness.
The fermentation broth of Reishi showed exceptional antidiabetic potential, considering that it inhibited the activity of α-amylase by as much as 78%.51 Moreover, Reishi extract has also been shown to be very effective at inhibiting α-glucosidase in the study of Joo et al.,52 who reported an inhibition extent of 89.68%.
In our study, the extracts showed significantly higher activity towards α-glucosidase than towards α-amylase, exerting an effect dependent on the tested concentration. It is worth mentioning that only the Kombucha fermented coffee extract had a higher activity than its unfermented extract, while for the green tea and Reishi extracts, the fermentation process did not enhance their antidiabetic properties. If we compare the obtained results through standard IC50 values, the values obtained in our study for acarbose were insignificantly lower than the ones reported previously.53 These results indicated that our extracts do not inhibit the activity of α-amylase, but the activity of α-glucosidase was substantial for further experimental design of our research. Recently, α-amylase has been found to play a significant role in the neuroinflammation associated with Alzheimer's disease. In particular, in order to function properly, brain cells require a constant supply of glucose. Moreover, aiming to prevent the risk of glucose deficiency in the event of an increased need for energy or hypoglycemia, the brain uses glycogen as a depot of energy. In other words, the activity of α-amylase in the brain represents a backup option that primarily functions under pathological conditions when astrocytes need energy for a rapid reaction.54 Therefore, it is considered that in the case of Alzheimer's disease, a low level of activity of this enzyme can lead to progressive memory loss,55 and it is important that the applied therapeutics do not inhibit it. On the other hand, patients with diabetes have an increased incidence of Alzheimer's disease,12 while the drugs they use are often based on the inhibition of α-amylase activity.56 Therefore, further research should be directed towards a more detailed definition of the role of this enzyme under physiological conditions in the brain, especially in the brain of people suffering from neurodegenerative diseases. Since the extracts examined in this study did not inhibit α-amylase, in the next steps their antineurodegenerative effect was examined.
Green tea extract studied by Kim et al.57 showed a similar level of antineurodegenerative activity to the one discovered in our study, as it inhibited the activity of AChE by 71%. Moreover, this extract studied by No et al.58 showed anti-TYR activity of 70%. To the best of our knowledge, there is no literature data on the effects of Kombucha fermented green tea extracts; however, there are studies that indicate that rutin, a flavonoid compound that we found in a large amount in both unfermented and fermented green tea extracts (Table 1), exhibits significant anti-AChE and anti-TYR activities.59
Previous studies showed that coffee extracts inhibit efficiently both AChE and TYR activities, which is believed to be due to a high amount of phenolic compounds, especially chlorogenic and caffeic acids, which are known to exhibit promising enzyme inhibitory effects both in in vitro and in vivo studies.48,60 Our literature survey showed that there are no previous studies on the anti-AChE and anti-TYR activities of the fermented coffee extracts, hence, the present study represents the first report on this topic.
According to our previous study, the extract of Reishi basidiocarps grown on an alternative substrate inhibited 61.33% of AchE at a concentration of 0.8 mg mL−1, while at 1.6 mg mL−1, it inhibited 86.42% of TYR activity.61 There is no previous data on the Kombucha fermented extracts of this medicinal mushroom inhibiting the AChE and TYR activities.
In addition to the inhibition of enzymes responsible for the onset and progression of neurodegenerative diseases, a great threat is also posed by neuroinflammatory processes, which cause and accelerate the process of the aforementioned diseases. Actually, it has been proven that the common denominator of neurodegenerative diseases is neuroinflammation, and various mitochondrial dysfunctions, which can also be associated with an increased level of oxidative and nitroxidative stress.62,63 In a state of increased oxidative stress, oxidative damage to essential molecules such as lipids, proteins and DNA in nerve and glial cells is induced, which further leads to the activation of glial cells and the release of pro-inflammatory cytokines, causing serious consequences that are associated with the development of neurodegeneration.63,64 Hence, since both unfermented and fermented extracts of green tea, coffee and Reishi showed promising antineurodegenerative potential, further studies should be focused on investigating their effects on glial and neural cells to discover whether they also possess antineuroinflammatory potential. On the other hand, it should be kept in mind that the assays applied in our research were chemical and biochemical ones, meaning that the experiments were carried out under in vitro conditions. Although this might be a drawback of our study, such tests had to be performed since to date, there are no data on the bioactivities of most of the studied extracts, but our results completely justify future cell cultures and in vivo studies.
Experimental
Chemicals and reagents
All used reagents and standards were of analytical grade. α-Amylase, α-glucosidase from Saccharomyces cerevisiae, type I, acetonitrile, acetylcholinesterase from Electrophorus electricus, acetylcholine iodide, acarbose, aesculetin, ascorbic acid, astragalin, BHA (2-tert-butyl-4-hydroxyanisole), BHT (3,5-di-tert-butyl-4-hydroxytoluene), β-carotene, caffeic acid, chlorogenic acid, disodium hydrogen phosphate dodecahydrate, DMSO (dimethyl sulfoxide), DPPH (2,2-diphenyl-1-picrylhydrazyl), DTNB (5,5′-dithiobis(2-nitrobenzoic acid)), galantamine, formic acid, iron(III) chloride, isoquercetin, kaempferol, kojic acid, L-DOPA (3,4-dihydroxy-L-phenylalanine), Lugol's solution, p-coumaric acid, p-hydroxybenzoic acid, pNPG (4-nitrophenyl β-D-glucopyranoside), protocatechuic acid, quercetin, quercitrin, rutin, sodium bicarbonate, sodium dihydrogen phosphate, sodium phosphate dihydrate monobasic, sodium hydrogen phosphate, sodium chloride, sodium carbonate anhydrous, syringic acid, tyrosinase from Agaricus bisporus, Tris base, and vitexin were obtained from Sigma-Aldrich, St Louis, USA. Dipotassium phosphate, disodium hydrogen phosphate dodecahydrate, methanol, and sodium phosphate dihydrate dibase were obtained from VWR, USA. Linoleic acid and Tween 40 were obtained from Acros Organics, Belgium. Potassium hexacyanoferrate(III) and trichloroacetic acid were obtained from Superlab, Serbia, while chloroform and hydrochloric acid were obtained from Zorka Pharma, Šabac, Serbia. Finally, 1% starch solution was obtained from Carl Roth, Germany.
Preparation of extracts
The medium on which Kombucha was grown was composed of: 1 L of water, 50 g of sucrose, and 20 g of dried and ground Camellia sinensis (green tea), Coffea arabica (coffee), or Ganoderma lucidum (Reishi) basidiocarp. The extraction material was poured into boiling water and the extraction was performed at room temperature on a magnetic stirrer for 30 minutes. The material was afterwards filtered through gauze and poured into 2 L glass jars covered with multiple layers of gauze and then autoclaved at 114 °C for 15 min. After sterilization, the extracts were cooled to room temperature when Kombucha inoculation was performed under sterile conditions. Cultivation was performed under static conditions at room temperature with a day/night light regime for 20 days. At the end of the fermentation period, the obtained fermentate was separated from the surface “cake”, lyophilized, and stored in a freezer for further experiments. The lyophilisates were used to prepare the tested concentrations of fermented extracts (0.25–10.00 mg mL−1).
The unfermented extracts were prepared as described in the previous paragraph; however, they were not inoculated with Kombucha.
Chemical compositions of the extracts
Since phenolic compounds are known to be sensitive to high temperatures, the best technique to identify them is, among others, liquid chromatography. The liquid chromatography separation followed by mass spectrometry can provide helpful information for the determination of the phenolic profile of a sample.31,35
The separation of phenolic compounds was performed using a Dionex Ultimate 3000 UHPLC system equipped with a diode array detector (DAD) connected to a TSQ Quantum Access Max triple-quadrupole mass spectrometer supplied with a heated electrospray ionization probe (HESI-II, Thermo Fisher Scientific, Germany) in negative ionization mode. For compound separation, a Syncronis C18 column (100 × 2.1 mm, 1.7 μm particle size) at 40 °C was used as follows: the flow rate was set to 0.3 mL min−1 and the mobile phase consisted of (A) water + 0.1% formic acid and (B) acetonitrile. Moreover, a linear gradient program was used as follows: 0.0–1.0 min 5% B, 1.0–14.0 min from 5% to 95% (B), 14.0–14.1 min from 95% to 5% (B), and 5% (B) for 6 min. The parameters of the ion source and the other MS data needed for quantification were as previously specified by Mišić et al.65 Finally, for instrument control, data acquisition, and data analysis, the Thermo Fisher Scientific Xcalibur software, version 2.1, was used.
Determination of antioxidant activity
The antioxidant activity of unfermented and Kombucha fermented green tea, coffee, and Reishi was determined by DPPH, total reducing power (TRP), and β-carotene bleaching assays.
DPPH assay
According to a procedure developed by Blois,66 with certain modifications, the DPPH test measures the antioxidant capacity of the extract through its ability to “scavenge” DPPH radicals. Thus, in the presence of antioxidants, the purple color of the 4% methanolic solution of DPP-hydrazyl changes to yellow-colored DPP-hydrazine. To a 96-well microtiter plate was added 20 μL of extracts and 180 μL of 4% DPPH solution (40 μg mL−1). Methanol was used as a negative control (blank), while BHA, BHT and ascorbic acid were used as positive controls. The absorbance was measured after 30 min of incubation in the dark, at room temperature, at 517 nm using a microtiter plate reader (Multiskan Sky Thermo Scientific, Finland). The inhibition of DPPH radicals in the presence of the tested extracts was calculated using the following formula and expressed as percentages (%) ± standard errors:
Inhibition of DPPH radicals (%) = [(A0 − As)/A0] × 100, |
A
0 is the absorbance of the blank, while As is the absorbance of the reaction mixture. The results are also presented as the half-maximal inhibitory concentration (IC50 – mg mL−1) derived from the percentage inhibition of DPPH radicals.
Determination of total reducing potential
The TRP of the extracts was determined by a method developed by Oyaizu,67 following a procedure introduced by Tusevski et al.68 with some modifications. This method is based on suppressing the ability of the extract to reduce Fe3+ to Fe2+, during which the solution develops a blue-green color, whose intensity is measured spectrophotometrically. The reaction mixture contained 20 μL of an extract, 40 μL of phosphate buffer (0.2 M, pH 6.6) and 40 μL of 1% potassium hexacyanoferrate(III). After incubation at 45 °C for 20 min, 40 μL of 10% trichloroacetic acid, 40 μL of distilled water, and 8 μL of 0.1% iron(III) chloride were added. After incubation at room temperature for 10 minutes, the absorbance was measured at 700 nm using a microtiter plate reader (Multiskan Sky Thermo Scientific, Finland). The reducing potential of the extracts was expressed as μmol equivalents of ascorbic acid (AAE) per gram of dry extract (μmol AAE per g of dry extract) ± standard errors.
β-Carotene bleaching assay
The β-carotene bleaching assay was performed according to a modified procedure developed by Dapkevicius et al.69 The principle of this assay lies in the fact that linoleic acid in the presence of O2 and ROS creates peroxyl radicals (ROO˙) that react with β-carotene to form a stable β-carotene radical and thus lead to the color loss of β-carotene. An emulsion was first made by dissolving linoleic acid (6.25 μL) and Tween 40 (50 mg) in a solution of β-carotene in chloroform (125 μL, 4 mg mL−1) and then adding 125 μL of chloroform. After dissolution, chloroform was evaporated using a rotary evaporator (Buchi rotavapor R-114, Switzerland) at 40 °C while the dry residue was redissolved with distilled water. BHA, BHT and ascorbic acid were used as positive controls, while 100% methanol was used as a blank. To each well of a microtiter plate, 200 μL of emulsion and 28 μL of the test substance (extracts/positive controls/100% methanol) were added. The addition of extracts and reagents (t = 0) was followed by an incubation that lasted for 120 min.
The absorbance was measured at 490 nm using a microtiter plate reader (Multiskan Sky Thermo Scientific, Finland). The antioxidant activity of the extracts was determined by monitoring the inhibition of β-carotene color loss, using the following equation, while the results were presented as percentages (%) ± standard errors:
% inhibition = [(A120 − C120)/(C0 − C120)] × 100, |
A
120 and C120 are the absorbances measured at t = 120 minutes for the extracts and the control (blank), while C0 is the absorbance of the control at t = 0 minutes. The results are also presented as the half-maximal inhibitory concentration (IC50 – mg mL−1) derived from the percentage inhibition of β-carotene bleaching.
Determination of antidiabetic activity
The antidiabetic activity of the extracts was assessed by determining the inhibition of α-amylase and α-glucosidase.
Determination of α-amylase inhibition
To determine the inhibition of α-amylase, the Caraway–Somogy iodine/potassium iodide method was used, performed according to Zengin et al.70 with some modifications. To each well of the microtiter plate, 25 μL of extract (S) and 50 μL of enzyme solution (0.5 mg mL−1) in sodium phosphate buffer (0.1 M, pH 6.8, with 6 mM sodium chloride) were added. After pre-incubation for 10 minutes at 37 °C, 50 μL of 0.2% starch was added and the plate was incubated for 10 minutes at 37 °C. The reaction was stopped by the addition of 25 μL of 1 M hydrochloric acid, followed by the addition of 100 μL of Lugol's solution. The enzyme control (C1) contained buffer instead of the extracts, the substrate control (C2) contained buffer instead of the enzyme, while the extracts’ color control (B) contained buffer instead of the enzyme, starch and Lugol's solution. Acarbose was used as a positive control. The absorbances were measured at 630 nm using a microtiter plate reader (Multiskan Sky Thermo Scientific, Finland). The percentages of α-amylase inhibition (% I) were calculated based on the following equation:
% Inhibition = [(S − B) − C1]/C2 × 100. |
Determination of α-glucosidase inhibition
To determine α-glucosidase inhibition, the pNPG (p-nitrophenyl-α-D-glucopyranoside) method was performed according to Wan et al.71. To each test well of a microtiter plate (S), 120 μL of extract of an appropriate concentration and 20 μL of enzyme solution (0.5 U mL−1) in potassium phosphate buffer (0.1 M, pH 6.8) were added. After incubation for 5 minutes at 37 °C, 20 μL of 5 mM pNPG was added and incubated for an additional 20 minutes at 37 °C. The reaction was stopped by adding 80 μL 0.2 M sodium carbonate in buffer. The control (C) contained buffer instead of the extracts, while the color control (B) contained all components except the enzyme, instead of which buffer was added. Acarbose was used as a positive control.
The absorbances were read at 405 nm using a microtiter plate reader (Multiskan Sky Thermo Scientific, Finland). The percentages of α-glucosidase inhibition (% I) were calculated based on the following equation:
% Inhibition = [C − (S − B)]/C × 100. |
The results are also presented as the half-maximal inhibitory concentration (IC50 – mg mL−1) derived from the percentage inhibition of α-glucosidase.
Determination of antineurodegenerative activity
The antineurodegenerative activity of the extracts was determined based on their capacity to inhibit acetylcholinesterase (AChE) and tyrosinase (TYR) activities.
Acetylcholinesterase inhibition test
The level of AChE inhibition was determined spectrophotometrically based on the procedure of Ellman et al.72 with certain modifications. The reaction mixture (S) contained 140 μL of sodium phosphate buffer (0.1 M, pH 7), 20 μL of DTNB solution, 20 μL of extracts’ solution in buffer containing 5% DMSO and 20 μL of AChE solution (5 U mL−1) in Tris-hydrochloric acid buffer (20 mM, pH 7.5). Galantamine was used as a positive control, while the mixture without extracts was used as a negative control (C). After incubation for 15 min at 25 °C, the reaction was initiated by the addition of 10 μL of acetylcholine iodide.
The absorbances were read at 412 nm using a microtiter plate reader (Multiskan Sky Thermo Scientific, Finland).
The percentages of enzyme inhibition were calculated based on the following equation:
Inhibition of AChE (%) = [(C − S)/C] × 100. |
The results are also presented as the half-maximal inhibitory concentration (IC50 – mg mL−1) derived from the percentage inhibition of AChE.
Tyrosinase inhibition test
The level of TYR inhibition was measured spectrophotometrically based on the procedure of Masuda et al.,73 with some modifications. The reaction mixture (S) was prepared by adding 80 μL of sodium phosphate buffer (0.1 M, pH 7), 40 μL of tyrosinase solution (46 U L−1) and 40 μL of the extracts. The mixture without extracts was used as a negative control (C), and kojic acid as a positive control. After adding 40 μL of L-DOPA solution in buffer and incubation (30 min, 25 °C), the absorbances were measured using a microtiter plate reader (Multiskan Sky Thermo Scientific, Finland) at 475 nm. The percentages of inhibition were determined based on the following equation:
Tyrosinase inhibition (%) = [(C − S)/C] × 100. |
The results are also presented as the half-maximal inhibitory concentration (IC50 – mg mL−1) derived from the percentage inhibition of TYR.
Statistical analysis
The assays were carried out in three replicates and the results are expressed as the mean ± standard error (standard deviation for LC-MS analysis). One-way analysis of variance (ANOVA) and Tukey's HSD post hoc test were performed to analyse any significant differences among means (p < 0.01). Moreover, in order to calculate the integrated biomarker response (IBR) values, the results for the applied assays used for the determination of biological activity of the unfermented and fermented extracts were individually standardized according to Beliaeff and Burgeot.74
Conclusions
The results of this study showed that there are noticeable differences between the chemical profile of the unfermented and Kombucha fermented extracts of green tea, coffee and Reishi, which we proved to have a great impact on their biological potential. These extracts exhibited great antioxidant potential, which revealed several mechanisms of action: their ability to transfer hydrogen atoms and individual electrons, protect other antioxidant substances by inhibiting their degradation, and manage redox reactions. Among the tested extracts, our IBR analysis revealed that the unfermented green tea extract, followed by the fermented coffee and green tea extracts, has the greatest antioxidant potential, and it also showed the highest enzyme-inhibitory potential. A complete IBR analysis confirmed that the unfermented green tea extract has the overall highest bioactivity, while the Reishi extracts showed the lowest biological potential in this study. Bearing in mind that oxidative stress is the basis of many human diseases, and the fact that nowadays there is a tendency to replace harmful synthetic products with natural preparations, the studied extracts were proven as potential functional foods since they exhibited promising inhibitory activity towards several enzymes responsible for the onset and progression of diabetes, Alzheimer's and Parkinson's diseases. Although there are studies that confirm the antioxidant, antidiabetic and antineurodegenerative activities of unfermented green tea, coffee and Reishi extracts, this research represents the first comprehensive report on their differences from the chemical profiles and activities of Kombucha fermented extracts. Moreover, the antineurodegenerative activities of Kombucha fermented green tea, coffee and Reishi extracts have never been reported elsewhere; hence, this also represents a significant novelty of this study. Finally, modern research has once more confirmed the traditional use of popular beverages and proved their biological activities, hence, giving a scientific basis for their use as functional foods.
Author contributions
Conceptualization – Sonja Duletić-Laušević and Mirjana Stajić; data curation – Mariana Oalđe Pavlović, Jasmina Ćilerdžić and Uroš Gašić; formal analysis – Mariana Oalđe Pavlović, Jasmina Ćilerdžić and Uroš Gašić; funding acquisition – Sonja Duletić-Laušević and Mirjana Stajić; investigation – Mariana Oalđe Pavlović, Jasmina Ćilerdžić and Uroš Gašić; methodology – Mariana Oalđe Pavlović and Jasmina Ćilerdžić; project administration – Sonja Duletić-Laušević and Mirjana Stajić; resources – Sonja Duletić-Laušević and Mirjana Stajić; supervision – Sonja Duletić-Laušević and Mirjana Stajić; validation – Sonja Duletić-Laušević and Mirjana Stajić; visualization – Mariana Oalđe Pavlović and Jasmina Ćilerdžić; writing – original draft – Mariana Oalđe Pavlović and Jasmina Ćilerdžić; writing – review & editing – Mirjana Stajić.
Conflicts of interest
There are no conflicts to declare.
Acknowledgements
This work was supported by the Ministry of Education and Science of the Republic of Serbia (Contract number: 451-03-68/2022-14/200178 and 451-03-68/2022-14/200007).
References
- J. Limón-Pacheco and M. E. Gonsebatt, The role of antioxidants and antioxidant-related enzymes in protective responses to environmentally induced oxidative stress, Mutat. Res., 2009, 674, 137–147 Search PubMed.
- N. Kushairi, C. W. Phan, V. Sabaratnam, P. David and M. Naidu, Lion's Mane Mushroom, Hericium erinaceus (Bull.: Fr.) Pers. suppresses H2O2-Induced oxidative damage and LPS-induced inflammation in HT22 hippocampal neurons and BV2 microglia, Antioxidants, 2019, 8, 261 CrossRef CAS PubMed.
- P. D. Ray, B. W. Huang and Y. Tsuji, Reactive oxygen species (ROS) homeostasis and redox regulation in cellular signalling, Cell Signaling, 2012, 24, 981–990 CrossRef CAS PubMed.
- A. Bloch-Damti and N. Bashan, Proposed mechanisms for the induction of insulin resistance by oxidative stress, Antioxid. Redox Signal., 2005, 7, 1553–1567 CrossRef CAS PubMed.
- H. C. Lo and S. P. Wasser, Medicinal mushrooms for glycemic control in diabetes mellitus: history, current status, future perspectives, and unsolved problems, Int. J. Med. Mushrooms, 2011, 13, 401–426 CrossRef CAS PubMed.
- H. Bischoff, W. Puls, H. P. Karause, H. Schutt and G. Thomas, Pharmacological properties of the novel glucosidase inhibitors BAY m 1099 (miglitol) and BAY o 1248, Diabetes Res. Clin. Pract., 1985, 1, 53–62 Search PubMed.
- P. Zimmet, K. G. Alberti and J. Shaw, Global and societal implications of the diabetes epidemic, Nature, 2001, 414, 782–787 Search PubMed.
- S. Dhital, A. H. M. Lin, B. R. Hamaker, M. J. Gidley and A. Muniandy, Mammalian mucosal α-glucosidases coordinate with α-amylase in the initial starch hydrolysis stage to have a role in starch digestion beyond glucogenesis, PLoS One, 2013, 8, 1–13 CrossRef PubMed.
- M. Bisaglia, M. E. Soriano, I. Arduini, S. Mammi and L. Bubacco, Molecular characterization of dopamine-derived quinones reactivity toward NADH and glutathione: Implications for mitochondrial dysfunction in Parkinson disease, Biochim. Biophys. Acta, 2010, 1802, 699–706 CrossRef CAS PubMed.
- Z. Ou, J. Pan, S. Tang, D. Duan, D. Yu, H. Nong and Z. Wang, Global trends in the incidence, prevalence, and years lived with disability of Parkinson's disease in 204 countries/territories from 1990 to 2019, Front. Public Health, 2021, 9, 776847 CrossRef PubMed.
- S. Angeloni, E. Spinozzi, F. Maggi, G. Sagratini, G. Caprioli, G. Borsetta, G. Ak, K. I. Sinan, G. Zengin, S. Arpini and G. Mombelli, Phytochemical profile and biological activities of crude and purified Leonurus cardiaca extracts, Plants, 2021, 10, 1–15 CrossRef PubMed.
- K. Mittal, R. J. Mani and D. P. Katare, Type 3 diabetes: Cross talk between differentially regulated proteins of type 2 diabetes mellitus and Alzheimer's disease, Sci. Rep., 2016, 6, 1–8 CrossRef PubMed.
- M. C. Achilonu and D. O. Umesiobi, Bioactive phytochemicals: bioactivity, sources, preparations, and/or modifications via silver tetrafluoroborate mediation, J. Chem., 2015, 2015, 423734 Search PubMed.
- J. Ćilerdžić, A. Alimpić Aradski, M. Stajić, J. Vukojević and S. Duletić-Laušević, Do Ganoderma lucidum and Salvia officinalis extracts exhibit synergistic antioxidant and antineurodegenerative effects?, J. Food Meas. Charact., 2019, 13, 3357–3365 CrossRef.
- B. Jacob and R. T. Narendhirakannan, Role of medicinal plants in the management of diabetes mellitus: A review, 3 Biotech, 2019, 9, 1–17 Search PubMed.
- S. Tasneem, B. Liu, B. Li, M. I. Choudhary and W. Wang, Molecular pharmacology of inflammation: Medicinal plants as anti-inflammatory agents, Pharmacol. Res., 2019, 139, 126–140 Search PubMed.
- M. Oalđe Pavlović, T. Lunić, S. Graovac, M. Mandić, J. Repac, U. Gašić, B. Božić Nedeljković and B. Božić, Extracts of selected Lamiaceae species as promising antidiabetics: Chemical profiling, in vitro and in silico approach combined
with dynamical modelling, Ind. Crops Prod., 2022, 186, 115200 CrossRef.
- N. Ł. Zofia, Z. Aleksandra, B. Tomasz, Z. D. Martyna, Z. Magdalena, H. B. Zofia and W. Tomasz, Effect of fermentation time on antioxidant and anti-ageing properties of green coffee Kombucha ferments, Molecules, 2020, 25, 5394 Search PubMed.
- L. Kallel, V. Desseaux, M. Hamdi, P. Stocker and E. H. Ajandouz, Insights into the fermentation biochemistry of Kombucha teas and potential impacts of Kombucha drinking on starch digestion, Food Res. Int., 2012, 49, 226–232 CrossRef CAS.
- K. G. Kanurić, S. D. Milanović, B. B. Ikonić, E. S. Lončar, M. D. Iličić, V. R. Vukić and D. V. Vukić, Kinetics of lactose fermentation in milk with kombucha starter, J. Food Drug Anal., 2018, 26, 1229–1234 CrossRef PubMed.
- Z. X. Ng, M. J. Y. Than and P. H. Yong,
Peperomia pellucida (L.) Kunth herbal tea: Effect of fermentation and drying methods on the consumer acceptance, antioxidant and anti-inflammatory activities, Food Chem., 2021, 344, 128738 CrossRef CAS PubMed.
- Z. X. Ng, E. Y. W. Soh and P. H. Yong, The influence of fermentation and drying methods on the functional activities and sensory quality of Artemisia argyi H.Lév. & Vaniot herbal tea, J. Appl. Res. Med. Aromat. Plants, 2022, 30, 100393 Search PubMed.
- R. Jayabalan, P. Subathradevi, S. Marimuthu, M. Sathishkumar and K. Swaminathan, Changes in free-radical scavenging ability of kombucha tea during fermentation, Food Chem., 2008, 109, 227–234 CrossRef CAS PubMed.
- S. A. Villarreal-Soto, S. Beaufort, J. Bouajila, J. P. Souchard and P. Taillandier, Understanding kombucha tea fermentation: A Review, J. Food Sci., 2018, 83, 580–588 Search PubMed.
- P. Bishop, E. R. Pitts, D. Budner and K. A. Thompson-Witrick, Kombucha: Biochemical and microbiological impacts on the chemical and flavour profile, Food Chem. Adv., 2022, 1, 100025 Search PubMed.
- R. V. Malbaša, E. S. Lončar, J. S. Vitas and J. M. Čanadanović-Brunet, Influence of starter cultures on the antioxidant activity of kombucha beverage, Food Chem., 2011, 127, 1727–1731 CrossRef.
- H. Battikh, A. Bakhrouf and E. Ammar, Antimicrobial effect of Kombucha analogues, LWT – Food Sci. Technol., 2012, 47, 71–77 CrossRef CAS.
- J. S. Vitas, A. D. Cvetanović, P. Z. Mašković, J. V. Švarc-Gajić and R. V. Malbaša, Chemical composition and biological activity of novel types of kombucha beverages with jarrow, J. Funct. Foods, 2018, 44, 95–102 Search PubMed.
- E. Zubaidah, F. J. Dewantari, F. R. Novitasari, I. Srianta and P. J. Blanc, Potential of snake fruit (Salacca zalacca (Gaerth.) Voss) for the development of a beverage through fermentation with the Kombucha consortium, Biocatal. Agric. Biotechnol., 2018, 13, 198–203 Search PubMed.
- B. B. Petrovska, Historical review of medicinal plants’ usage, Pharmacogn. Rev., 2012, 6, 1–5 Search PubMed.
- F. Carmona and A. M. S. Pereira, Herbal medicines: old and new concepts, truths and misunderstandings, Rev. Bras. Farmacogn., 2013, 23, 379–385 CrossRef.
-
Plant secondary metabolites: occurrence, structure and role in the human diet, ed. A. Crozier, M. N. Clifford and H. Ashihara, Blackwell Publishing, New Jersey, 2007 Search PubMed.
- J. M. Lorenzo and P. E. S. Munekata, Phenolic compounds of green tea: Health benefits and technological application in food, Asian Pac. J. Trop. Biomed., 2016, 6, 709–719 CrossRef CAS.
- F. Shahidi and J. Yeo, Bioactivities of phenolics by focusing on suppression of chronic diseases: a review, Int. J. Mol. Sci., 2018, 19, 1573 CrossRef PubMed.
- R. V. Malbaša, E. S. Lončar and L. A. Kolarov, TLC analysis of some phenolic compounds in Kombucha beverage, Acta Period. Technol., 2004, 35, 199–205 CrossRef.
- E. Ivanišová, K. Meňhartová, M. Terentjeva, L. Godočíková, J. Árvay and M. Kačániová, Kombucha tea beverage: microbiological characteristic, antioxidant activity, and phytochemical composition, Acta Aliment., 2019, 48, 324–331 Search PubMed.
- G. Williamson, F. Dionisi and M. Renouf, Flavanols from green tea and phenolic acids from coffee: critical quantitative evaluation of the pharmacokinetic data in humans after consumption of single doses of beverages, Mol. Nutr. Food Res., 2011, 55, 864–873 Search PubMed.
- T. Oludemi, L. Barros, M. A. Prieto, S. A. Heleno, M. F. Barreiro and I. C. Ferreira, Extraction of triterpenoids and phenolic compounds from Ganoderma lucidum: optimization study using the response surface methodology, Food Funct., 2018, 9, 209–226 RSC.
- R. Manian, N. Anusuya, P. Siddhuraju and S. Manian, The antioxidant activity and free radical scavenging potential of two different solvent extracts of Camellia sinensis (L.) O. Kuntz, Ficus bengalensis L. and Ficus racemosa L., Food Chem., 2008, 107, 1000–1007 CrossRef CAS.
- M. H. Santos, B. L. Batista, S. M. Silveira Duarte, C. M. P. Abreu and C. Gouvea, Influence of processing and roasting on the antioxidant activity of coffee (Coffea arabica), Quim. Nova, 2007, 30, 604–610 Search PubMed.
- C. Xiao Ping, C. Yan, L. Shui Bing, C. You Guo, L. Jian Yun and L. Lan Ping, Free radical scavenging of Ganoderma lucidum polysaccharides and its effect on antioxidant enzymes and immunity activities in cervical carcinoma rats, Carbohydr. Polym., 2009, 77, 389–393 CrossRef.
- A. Sknepnek, M. Pantić, D. Matijašević, D. Miletić, S. Lević, V. Nedović and M. Nikšić, Novel kombucha beverage from lingzhi or reishi medicinal mushroom, Ganoderma lucidum, with antibacterial and antioxidant effects, Int. J. Med. Mushrooms, 2018, 20, 243–258 CrossRef PubMed.
- Z. X. Ng and U. R. Kuppusamy, Effects of different heat treatments on the antioxidant activity and ascorbic acid content of bitter melon, Momordica charantia, Braz, J. Food Technol., 2019, 22, e2018283 Search PubMed.
- M. A. Darenskaya, L. I. Kolesnikova and S. I. Kolesnikov, Oxidative stress: Pathogenetic role in diabetes mellitus and its complications and therapeutic approaches to correction, Bull. Exp. Biol. Med., 2021, 171, 179–189 Search PubMed.
- D. Kajaria, J. T. Ranjana, Y. B. Tripathi and S. Tiwari,
In vitro α-amylase and glycosidase inhibitory effect of ethanolic extract of antiasthmatic drug – Shirishadi, J. Adv. Pharm. Technol. Res., 2013, 4, 206–209 CrossRef PubMed.
- A. Duangjai, P. Pontip, S. Sumhem, W. Kaweekul, M. Utsintong, A. Ontawong, K. Trisat and S. SaoKaew, Phenolic acids from Coffea arabica L. suppress intestinal uptake of glucose and cholesterol, Biomed. Res., 2020, 31, 59–66 CAS.
- B. Nickavar and N. Yousefian, Evaluation of a-amylase inhibitory activities of selected antidiabetic medicinal plants, J. Verbraucherschutz Lebensmittelsicherh., 2010, 6, 191–195 CrossRef.
- G. Zengin, K. I. Sinan, M. F. Mahomoodally, S. Angeloni, A. M. Mustafa, S. Vittori, F. Maggi and G. Caprioli, Chemical composition, antioxidant and enzyme inhibitory properties of different extracts obtained from spent coffee ground and coffee silverskin, Foods, 2020, 9, 713 Search PubMed.
- M. I. Watawana, N. Jayawardena and V. Y. Waisundara, Enhancement of the functional properties of coffee through fermentation by “Tea Fungus”(Kombucha), J. Food Process. Preserv., 2015, 39, 2596–2603 Search PubMed.
- M. I. Watawana, N. Jayawardena and V. Y. Waisundara, Value-added Tea (Camellia sinesis) as a functional food using the Kombucha ‘Tea Fungus’, Chiang Mai J. Sci., 2018, 45, 136–146 CAS.
- R. Sarnthima, S. Khammaung and P. Sa-ard, Culture broth of Ganoderma lucidum exhibited antioxidant, antibacterial and a-amylase inhibitory activities, J. Food Sci. Technol., 2017, 54, 3724–3730 CrossRef CAS PubMed.
- O. S. Joo, C. E. Hwang, S. Y. Hong, E. C. Sin, S. H. Nam and K. M. Cho, Antioxidative and digestion enzyme inhibitory activity of Ganoderma lucidum depends on the extraction solvent, Korean J. Food Preserv., 2018, 25, 124–135 CrossRef.
- K. L. Ho, C. G. Tan, P. H. Yong, C. W. Wang, S. H. Lim, U. R. Kuppusamy, C. T. Ngo, F. Massawe and Z. X. Ng, Extraction of phytochemicals with health benefit from Peperomia pellucida (L.) Kunth through liquid-liquid partitioning, J. Appl. Res. Med. Aromat. Plants, 2022, 30, 100392 Search PubMed.
- S. Agatonović-Kuštrin, E. Kuštrin, V. Gegechkori and D. W. Morton, Bioassay-guided identification of α-amylase inhibitors in herbal extracts, J. Chromatogr. A, 2020, 1620, 460970 Search PubMed.
- E. Byman, I. Martinsson, H. Haukedal, Netherlands Brain Bank, G. Gouras, K. K. Freude and M. Wennström, Neuronal α–amylase is important for neuronal activity and glycogenolysis and reduces in presence of amyloid beta pathology, Aging Cell, 2021, 20, e13433 CrossRef CAS PubMed.
- P. M. Sales, P. M. Souza, L. A. Simeoni, P. O. Magalhães and D. Silveira, α-Amylase inhibitors: a review of raw material and isolated compounds from plant source, J. Pharm. Pharm. Sci., 2012, 15, 141–183 Search PubMed.
- H. K. Kim, M. Kim, S. Kim, M. Kim and J. H. Chung, Effects of green tea polyphenol on cognitive and acetylcholinesterase activities, Biosci., Biotechnol., Biochem., 2004, 68, 1977–1979 CrossRef CAS PubMed.
- J. K. No, D. Y. Soung, Y. J. Kim, K. H. Shim, Y. S. Jun, S. H. Rhee, T. Yokozawa and H. Y. Chung, Inhibition of tyrosinase by green tea components, Life Sci., 1999, 65, 241–246 CrossRef PubMed.
- E. Neagu, G. Paun, C. Albu and G. L. Radu, Assessment of acetylcholinesterase and tyrosinase inhibitory and antioxidant activity of Alchemilla vulgaris and Filipendula ulmaria extracts, J. Taiwan Inst. Chem. Eng., 2015, 52, 1–6 CrossRef CAS.
- K. Iwai, N. Kishimoto, Y. Kakino, K. Mochida and T. Fujita, In vitro antioxidative effects and tyrosinase inhibitory activities of seven hydroxycinnamoyl derivatives in green coffee beans, J. Agric. Food Chem., 2004, 52, 4893–4898 Search PubMed.
- J. Lj. Ćilerdžić, I. V. Sofrenić, V. V. Tešević, I. D. Brčeski, S. N. Duletić-Laušević, J. B. Vukojević and M. M. Stajić, Neuroprotective potential and chemical profile of alternatively cultivated Ganoderma lucidum, basidiocarps, Chem. Biodivers., 2018, 15, e1800036 CrossRef PubMed.
- M. H. Pourhanifeh, R. Shafabakhsh, R. J. Reiter and Z. Asemi, The effect of resveratrol on neurodegenerative disorders: possible protective actions against autophagy, apoptosis, inflammation and oxidative stress, Curr. Pharm. Des., 2019, 25, 2178–2191 Search PubMed.
- D. S. Simpson and P. L. Oliver, ROS generation in microglia: Understanding oxidative stress and inflammation in neurodegenerative disease, Antioxidants, 2020, 9, 743 CrossRef CAS PubMed.
- J. van Horssen, P. van Schaik and M. Witte, Inflammation and mitochondrial dysfunction: A vicious circle in neurodegenerative disorders?, Neurosci. Lett., 2019, 710, 132931 CrossRef PubMed.
- D. Mišić, B. Šiler, U. Gašić, S. Avramov, S. Živković, J. Nestorović Živković, M. Milutinović and Ž. Tešić, Simultaneous UHPLC/DAD/(+/–) HESI–MS/MS analysis of phenolic acids and nepetalactones in methanol extracts of Nepeta species: A possible application in chemotaxonomic studies, Phytochem. Anal., 2015, 26, 72–85 CrossRef PubMed.
- M. S. Blois, Antioxidant determinations by the use of a stable free radical, Nature, 1958, 181, 1199–1200 CrossRef CAS.
- M. Oyaizu, Studies on products of browning reactions: antioxidative activities of products of browning reaction prepared from glucosamine, Jpn. J. Nutr. Diet., 1986, 44, 307–315 Search PubMed.
- O. Tusevski, A. Kostovska, A. Iloska, L. Trajkovska and S. Simic, Phenolic production and antioxidant properties of some Macedonian medicinal plants, Cent. Eur. J. Biol., 2014, 9, 888–900 CAS.
- A. Dapkevicius, R. Venskutonis, T. A. Van Beek and J. P. H. Linssen, Antioxidant activity of extracts obtained by different isolation procedures from some aromatic herbs grown in Lithuania, J. Sci. Food Agric., 1998, 77, 140–146 Search PubMed.
- G. Zengin, A. Uysal, E. Gunes and A. Aktumsek, Survey of phytochemical composition and biological effects of three extracts from a wild plant (Cotoneaster nummularia Fisch. et Mey.): a potential source for functional food ingredients and drug formulations, PLoS One, 2014, 9, e113527 CrossRef PubMed.
- L. S. Wan, Q. X. Min, Y. L. Wang, Y. D. Yue and J. C. Chen, Xanthone glycoside constituents of Swertia kouitchensis with α-glucosidase inhibitory activity, J. Nat. Prod., 2013, 76, 1248–1253 CrossRef CAS PubMed.
- G. L. Ellman, K. Courtney, V. Andres and R. M. Featherstone, A new and rapid colorimetric determination of acetylcholinesterase activity, Biochem. Pharmacol., 1961, 7, 88–95 Search PubMed.
- T. Masuda, D. Yamashita, Y. Takeda and S. Yonemori, Screening for tyrosinase inhibitors among extracts of seashore plants and identification of potent inhibitors from Garcinia subelliptica, Biosci., Biotechnol., Biochem., 2005, 69, 197–201 CrossRef CAS PubMed.
- B. Beliaeff and T. Burgeot, Integrated biomarker response: a useful tool for ecological risk assessment, Environ. Toxicol. Chem., 2002, 21, 1316–1322 CrossRef CAS PubMed.
|
This journal is © The Royal Society of Chemistry 2023 |
Click here to see how this site uses Cookies. View our privacy policy here.