DOI:
10.1039/D2FO03912E
(Paper)
Food Funct., 2023,
14, 7361-7374
Protein blends and extrusion processing to improve the nutritional quality of plant proteins†
Received
16th December 2022
, Accepted 17th July 2023
First published on 25th July 2023
Abstract
Plant proteins have low protein nutritional quality due to their unbalanced indispensable amino acid (IAA) profile and the presence of antinutritional factors (ANFs) that limit protein digestibility. The blending of pulses with cereals/pseudocereals can ensure a complete protein source of IAA. In addition, extrusion may be an effective way to reduce ANFs and improve protein digestibility. Thereby, we aimed to improve the protein nutritional quality of plant protein ingredients by blending different protein sources and applying extrusion processing. Protein blends were prepared with pea, faba bean, quinoa, hemp, and/or oat concentrates or flours, and extrudates were prepared either rich in pulses (texturized vegetable proteins, TVPs) or rich in cereals (referred to here as Snacks). After extrusion, all samples showed a reduction in trypsin inhibitor activity (TIA) greater than 71%. Extrusion caused an increase in the total in vitro protein digestibility (IVPD) of TVPs, whereas no significant effect was shown for the snacks. According to the molecular weight distribution, TVPs presented protein aggregation. The results suggest that the positive effect of decreased TIA on IVPD is partially counteracted by the formation of aggregates during extrusion which restricts enzyme accessibility. After extrusion, all snacks retained a balanced amino acid score whereas a small loss of methionine + cysteine was observed in the TVPs, resulting in a small reduction in IAA content. Thus, extrusion has the potential to improve the nutritional quality of TVPs by reducing TIA and increasing protein digestibility.
1. Introduction
The transition from an animal protein-based diet to a plant protein-based diet has become increasingly important for consumers due to environmental and health awareness.1 However, this transition can be limited by the fact that plant proteins have a lower nutritional quality than animal proteins. Protein nutritional quality is defined as how proteins are digested and how much they can provide of the indispensable amino acids (IAAs).2 Plant proteins have low nutritional quality due to their inability to provide sufficient levels of all IAAs required for human metabolism. In addition, plant proteins contain antinutritional factors (ANFs), such as protease inhibitors that decrease protein digestibility.3 Strategies to improve the nutritional quality of plant proteins include the use of protein blends and food processing to inactivate ANFs. Regarding protein blends, the combination of different protein sources can ensure a balanced IAAs profile. For instance, pulses are rich in IAAs such as lysine but low in the sulfur-containing amino acids methionine and cysteine.4 To ensure a balanced amino acid profile, pulses can be blended with cereals, and/or pseudocereals which contain a sufficient level of the sulfur-containing amino acids.5,6 This combination provides a plant protein source with enhanced protein quality. Furthermore, food processing, such as thermal processing, has been reported to be an effective way to reduce or inactivate heat-sensitive ANFs, resulting in increased protein digestibility.7 Extrusion is a thermomechanical process that involves applying high temperatures and mechanical forces to produce texturized proteins.8 Besides changing the structure of protein matrices, extrusion can reduce ANFs7 and therefore, potentially improve protein digestibility. Overall, a combination of protein blends with the extrusion process may be an effective way to boost the protein quality of plant protein-based products. Therefore, we aimed to improve the protein nutritional quality of plant protein ingredients by using protein blends to improve the amino acid profile and using extrusion processing to reduce ANFs thereby increasing protein digestibility.
2. Materials and methods
2.1. Chemicals
The enzymes used in this study were obtained from Sigma-Aldrich Denmark A/S (Søborg, DK) with the following specifications: bovine trypsin with a specific activity of 10
500 units per mg solids for the determination of trypsin inhibitors, pepsin from porcine gastric mucosa (≥250 units per mg solids) and pancreatin from porcine pancreas (4× USP specifications) for in vitro protein digestibility. All the other chemicals were also obtained from Sigma-Aldrich unless stated differently. Ultra-pure water (Milli-Q water system, Millipore Corporation, Merck) was used to prepare all the solutions unless stated differently.
2.2. Raw materials and extrudates
The protein ingredients and extrudates (textured vegetable proteins pulse-rich, TVPs, and extrudates cereal-rich, referred to as Snacks) were supplied by Organic Plant Protein A/S (Hedensted, DK) who sourced the pea (Pisum sativum L.) and faba (Vicia faba L.) protein concentrates from Vestkorn A/S (Holstebro, DK), the quinoa seeds (Chenopodium quinoa Willd) from Quinoa Quality A/S (Regstrup, DK), the oat (Avena sativa L.) flour from Dalby Mølle A/S (Kolding, DK), and the hemp (Cannabis sativa) protein concentrate from Dava Foods A/S (Hadsund, DK). Briefly, the pea protein concentrate (48.26% protein) and faba protein concentrate (56.89% protein) were produced by dry fractionation including heat treatment between 60 and 70 °C. The quinoa (Vikinga cultivar, 11.25% protein) and oat (10.92% protein) wholegrain flours were produced by milling. The hemp protein ingredient was obtained by first milling and grounding the residual cold-pressed oil cake from hemp seeds (Finola® cultivar). Then, the obtained flour was sieved mechanically, with a maximum temperature of 40 °C, into two fractions – a protein-rich fraction containing 50% of protein and a fiber-rich fraction. No further thermal or chemical process was involved. Information about the chemical composition of the protein ingredients supplied by the manufacturers is found in ESI 1.†
Three TVPs and three Snacks containing different blends of plant protein ingredients were prepared by low moisture extrusion (LME, 7–8% moisture) in a twin screw extruder (Clextral A/S, France) at Organic Plant Protein A/S (Hedensted, DK) (pictures of the TVPs and Snacks can be seen in ESI 2†). The TVPs and Snacks composition was based on an original recipe used by the company, which here is referred to as TVP 1 and Snack 1. Therefore, the composition of the other TVPs and Snacks was based on improving the amino acid profile of TVP 1 and Snack 1. The weight percentage of each plant protein ingredient was based to guarantee the same protein content among the three TVPs and the three Snacks (Table 1). In the extruder, the barrel temperature ranged from 40–165 °C for TVPs and from 30–145 °C for Snacks. The screw speed ranged from 783–794 rpm, the water pump was set at 25.0 L h−1, and the flow rate ranged from 190–220 kg h−1. Before analysis, TVP and Snack extrudates were ground to flour using a coffee grinder (Braun type 4047, Mexico) for 10 seconds. To compare samples before and after extrusion, a raw mixture (RM) of the protein ingredients was prepared by blending the equivalent protein ingredient composition for each extrudate. The RMs were hand shaken for 5 minutes to allow a homogeneous mixture. All samples were stored in closed packages and stored at room temperature until analyses.
Table 1 Ingredient composition of TVPs and Snacks extrudates prepared with a blend of plant proteins
Extrudates |
Composition |
The percentage of ingredients represents the weight percentage. The choice of the proportion of ingredients in each extrudate was based on the fact that TVPs and Snacks should have similar protein content, within the same group of the extrudate, and improved amino acid profile compared to TPV 1 and Snack 1. |
TVP 1 |
89% pea, 10% faba beans and 1% NaCl |
TVP 2 |
49% faba beans, 40% pea, 10% quinoa and 1% NaCl |
TVP 3 |
79% pea, 10% faba beans, 10% hemp and 1% NaCl |
Snack 1 |
60% oat, 39% pea and 1% NaCl |
Snack 2 |
50% quinoa, 39% pea, 10% oat and 1% NaCl |
Snack 3 |
59% oat, 20% pea, 20% hemp and 1% NaCl |
2.3. Chemical composition
The chemical composition and dietary fiber content of TVPs and Snacks were determined by Eurofins Steins Laboratorium A/S (Vejen, Denmark) in duplicates. According to Eurofins reports, carbohydrate, fat, and ash contents were analyzed by gravimetry, the dietary fibers were analyzed by enzymatic gravimetry, and the protein content was determined by the Kjeldahl method. The crude protein content was calculated from total nitrogen using a standard conversion factor of 6.25. The moisture content was measured in duplicates using a moisture analyzer at 130 °C (Mettler Toledo A/S, Denmark).
2.4. Determination of the molecular weight distribution
The protein molecular weight distribution of the protein ingredients, RMs, and extrudates was determined using Sodium-Dodecyl-Sulfate Polyacrylamide Gel Electrophoresis (SDS-PAGE) under reducing and non-reducing conditions. Protein extraction was carried out as follows: 10–20 mg sample was suspended in 600 μl Tris buffer (0.1 M, containing 5% SDS, pH 8.0), placed in a mixer mill for 5 minutes at frequency 30, and then, heated for 10 minutes at 80 °C and 350 rpm in a thermomixer (Eppendorf, DK). Afterward, the samples were centrifuged for 5 minutes at 20
000g and the supernatant was collected. The soluble protein concentration in the supernatant was measured at 280 nm using a nanodrop UV-Vis spectrophotometer (Thermo Fisher Scientific, Waltham, MA). Then, the following mixture was prepared: 25 μl 4× LDS sample buffer (lithium dodecyl sulfate, pH 8.4), 10 μl dithiothreitol (DTT, 1 M), and 65 μl of diluted sample to achieve a final protein concentration of 1 mg mL−1. For the non-reduced samples, DTT was replaced with ultra-pure water in the same volume. The sample mixtures were then heated for 10 minutes at 80 °C and 350 rpm in a thermomixer. Electrophoresis was carried out using Nupage 12% Bis-Tris gels (Thermo Fisher Scientific, Waltham, MA) and MOPS SDS running buffer (Thermo Fisher Scientific, Waltham, MA) in an XCell SureLock Mini-Cell Electrophoresis System (Thermo Fisher Scientific, Waltham, MA). The wells were loaded with 10 μl of sample and 5 μl of pre-stained protein standard (Thermo Fisher Scientific, Waltham, MA). After running the gels, they were stained with 2% Coomassie Brilliant Blue and equilibration buffer (0.4 M ammonium sulfate, 0.2 M 86% phosphoric acid, 3.9 M 96% ethanol) in a ratio of 1
:
100 overnight on a rocking table (Polymax 1040, Heidolph, DE). The following day, the gels were washed in ultra-pure water and scanned using a gel scanner (Epson Perfection V850 Pro) and Phoretix TL120 software.
2.5. Confocal laser scanning microscopy (CLSM)
Confocal microscopy was done using the TCS SP5-X point scanning confocal microscope (Leica Microsystems GmbH, Wetzlar, Germany) to observe the morphological characteristics of extrudates and raw mixtures. Extrudates were rehydrated using water and sliced carefully to avoid damaging the structure. Raw mixtures were resuspended in a few drops of water and placed on a 10-well slide. Samples were stained with fast green FCF (1 mg mL−1), Nile red (0.5 mg mL−1), and 60 μl of calcofluor (0.3 mg mL−1) directly on the microscope slides and incubated for 5 min before visualization. β-Glucans (fibers) were visualized using calcofluor white excited with a 355 nm UV laser and detected at 400–460 nm. Nile red and fast green FCF were excited using a supercontinuum white light laser (WLL) at 488 and 633 nm, respectively. Nile red was used to visualize lipid droplets between 515–550 nm and fast green FCF was used to stain protein and was detected between 660–720 nm. Images were taken using a 63× water-immersion lens and sequential scans to limit crosstalk.
2.6. Trypsin inhibitor activity (TIA) assay
The trypsin inhibitor activity was determined by the American Oil Chemists’ Society (AOCS) Method Ba 12a-2020 according to Liu et al. (2021).9 Briefly, 0.9–1.0 g of ground sample was extracted with 50 mL of 10 mM NaOH solution while stirring for 3 hours at 720 rpm. The sample extract was diluted with ultra-pure water so that 1.0 mL of the diluted sample resulted in 30–70% trypsin inhibition. Then, 1.0 ml of diluted extract sample (sample reading) and 1.0 ml of ultra-pure water were added to different tubes (reference reading) and placed in a water bath (LSB Aqua Pro, Grant Instruments, Cambridge, UK) at 37 °C. Afterward, the prewarmed (37 °C) substrate N-α-benzoyl-DL-arginine-p-nitroanilide (DL-BAPA) solution (0.4 mg mL−1 in 50 mM Tris buffer containing 20 mM calcium chloride (CaCl2), pH 8.2) was added to the tubes and vortexed at 1000 rpm for 10 seconds. Then, 1 ml of trypsin solution (20 μg mL−1 in 1 mM hydrochloric acid (HCL) with 5 mM CaCl2) was added and tubes were vortexed for 10 seconds. After 10 minutes, 0.5 ml acetic acid (30% v/v) was added to stop the reaction and the tubes were vortexed as previously described. For sample and reference blanks, acetic acid was added before the addition of the trypsin solution. The reaction mixtures were centrifuged at 1500g for 5 minutes. The absorbance of the supernatant was measured at 410 nm and 1.0 cm light path using a UV-Vis spectrophotometer (Shimadzu UV-1800; Cole-Parmer Instrument Company Ltd, UK). TIA was represented as trypsin inhibited units (TIU) per mg of sample and it was calculated as follows: |  | (1) |
where A410R = reference reading, A410RB = reference blank, A410S = sample reading, A410SB = sample blank, and mL of a diluted sample extract used for the assay = 1 mL. One trypsin unit (TU) is defined as an increase of 0.02 absorbance at 410 nm.10 The sample concentration was adjusted for the dilution factor.
The TIU per mg sample (as is) values were converted to dry matter basis by considering the moisture content of the samples. To allow comparison of samples with different protein contents, TIU per mg sample (dry basis) values were divided by the protein content (%). All samples were measured in three-independent replicates and each replicate was measured twice per assay.
2.7. Determination of in vitro protein digestibility (IVPD)
The gastrointestinal protein digestion was evaluated using a static, multistep in vitro protein digestibility (IVPD) assay according to Joehnke et al. (2018).11 In summary, the samples were weighed to contain 50 mg protein and solubilized in 10 mL of 0.05 M HCL overnight at 5 °C. Equivalent amounts of bovine serum alanine (BSA, as a reference), free alanine as internal standard, and blank samples containing only 0.05 M HCL were run simultaneously. On the following day, gastric digestion was simulated by a freshly prepared pepsin solution (1 mg mL−1 pepsin in 0.05 M acetate buffer, pH 4.5, 920 U mg−1 protein, from porcine gastric mucosa) for 1 h at 37 °C and 80 rpm in a shaking water bath (LSB Aqua Pro, Grant Instruments, Cambridge, UK). The pH of sample solutions containing pepsin to initiate digestion was below 2.0. Then, the small intestinal digestion was simulated by first adding to the residual sample solution a mix of salts: sodium bicarbonate buffer (2.35 mL, 0.6 M), NaOH (0.1 mL, 1.15 M), and freshly prepared sodium cholate solution (0.05 mL, 100 mg mL−1 sodium cholate hydrate prepared in 0.6 M sodium bicarbonate buffer). Secondly, a freshly prepared pancreatin solution (2.38 mL, 2 mg mL−1 pancreatin in 1 mM HCl; pancreatin from the porcine pancreas) and samples were incubated for 1 h more at 37 °C and 80 rpm. The enzyme-to-substrate ratios were kept constant at 1
:
50 (w/w) for pepsin digestion and 1
:
10 (w/w) for pancreatin digestion. Before digestion and after each stage (pepsin and pancreatin digestion), aliquots were withdrawn and diluted 1
:
10 v/v in sodium borate buffer (0.05 M, pH 10.0) to stop enzymatic hydrolysis. Samples were stored at 5 °C until further analyses. The IVPD (%) was determined by the quantification of free α-amino groups released during protein hydrolysis by the trinitrobenzenesulfonic acid (TNBS) colorimetric method using a microplate reader (Epoch 2, Biotek Instruments, Inc., Winooski, USA), described according to Joehnke et al. (2018).11 Shortly, alanine solution (0.2 mg mL−1DL-alanine in 0.05 M borate buffer, pH 10.0) was used for the calibration curve representing free α-amino groups and blanks only containing borate buffer (0.05 M, pH 10.0). Samples were diluted in a 2-fold series dilution with borate buffer (0.05 M, pH 10.0). In the final step, TNBS solution (0.1% 2,4,6-TNBS in H2O; picrylsulfonic acid solution) was added to all samples, calibration curve, and blank. The reaction with primary α-amino groups was detected continuously at 37 °C for 10 min at 450 nm. The absorbance curves were assessed using Gen5 v.3.11 Data Analysis Software (BioTek Instruments, VT) and fitted using a four-parameter logistic model. The concentration of α-amino groups was estimated from the alanine calibration curve. The IVPD (%) of all samples was determined as the ratio between the concentration of free α-amino groups and alanine internal standard which represented 100% protein digestibility, after correction with blanks (only containing enzymes) and undigested samples. To allow comparison of samples tested on different days, the total IVPD (%) was calculated relative to BSA, with BSA representing 100% digestibility. All samples were digested in independent triplicates.
2.8. Determination of the amino acid composition and score
The total amino acid content of individual protein ingredients and extrudates was analyzed by SGS (Germany GmbH, Hamburg) using an acid hydrolysis method and LC-MS/MS detection. Except for tryptophan content which was determined using alkaline hydrolysis, as it is destroyed during acid hydrolysis. A theoretical amino acid content was calculated for the RMs using the results obtained for each protein ingredient. The amino acid score was calculated based on the World Health Organisation (WHO) adult recommendation of 0.66 g protein per kg body weight using the following equations.12 The amino acid score was truncated. | 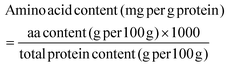 | (2) |
| 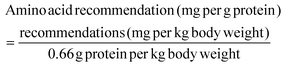 | (3) |
|  | (4) |
| 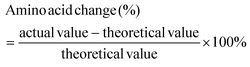 | (5) |
2.9. Statistical analysis
All results are expressed as the mean and standard deviation of independent samples. Statistical analysis was done using the Statistical Package for the Social Sciences (SPSS software v. 28.01, IBM Inc.). One-way analysis of variance (ANOVA) followed by Tukey's post hoc test was used to compare the results of TIA and IVPD among different samples. The significance level was set at p < 0.05.
3. Results & discussion
3.1. Proximate composition of extrudates
The proximate composition of the TVP and Snack extrudates is outlined in Table 2. All TVPs had a similar protein content ranging from 51.2 to 52.3 g per 100 g of the extrudate, which was expected as the combination of the protein ingredients was designed to reach similar protein content among all TVPs. Snacks had a lower protein content than the TVPs ranging from 27.7 to 28.9 g per 100 g of the extrudate and higher carbohydrate content. Snacks were prepared with a higher content of cereals and pseudocereals which goes in line with the carbohydrate content found. All extrudates are considered a high source of dietary fiber showing an amount higher than 12 g per 100 g of the extrudate.
Table 2 Proximate composition of extrudates produced with different protein blends
g per 100 g |
TVP 1 |
TVP 2 |
TVP 3 |
Snack 1 |
Snack 2 |
Snack 3 |
The results are shown as mean ± standard deviation of duplicates. |
Carbohydrate |
14.9 ± 0.78 |
14.6 ± 0.57 |
14.4 ± 0.21 |
45.5 ± 1.70 |
42.4 ± 0.71 |
41.0 ± 0.64 |
Dietary fiber |
15.3 ± 0.57 |
14.3 ± 0.28 |
15.4 ± 0.35 |
12.4 ± 1.34 |
12.5 ± 0.42 |
13.8 ± 0.57 |
Fat |
4.2 ± 0.07 |
5.0 ± 0.85 |
4.7 ± 0.21 |
5.8 ± 0.07 |
6.6 ± 0.42 |
6.7 ± 0.28 |
Protein |
51.2 ± 0.50 |
52.3 ± 0.28 |
51.6 ± 0.21 |
27.7 ± 0.35 |
28.9 ± 0.35 |
27.7 ± 0.14 |
Ash |
6.0 ± 0.01 |
6.0 ± 0.04 |
6.3 ± 0.07 |
4.1 ± 0.01 |
4.9 ± 0.03 |
4.7 ± 0.04 |
Moisture |
8.8 ± 0.06 |
7.7 ± 0.21 |
7.9 ± 0.05 |
4.6 ± 0.01 |
4.8 ± 0.42 |
6.2 ± 0.3 |
3.2. Molecular weight distribution of raw mixtures and extrudates
The molecular weight distribution of raw mixtures (RM) before extrusion and of extrudates (TVPs and Snacks) were determined by electrophoresis under non-reducing and reducing conditions, aiming to investigate if the extrusion process induced protein aggregation. Gels of the individual protein ingredients can be seen in ESI 3.†Fig. 1A and B shows the molecular weight distribution of RMs and TVPs. The main protein groups identified under non-reducing conditions were legumin, vicilin, and convicilin appearing between the molecular weight of 70 and 30 kDa (Fig. 1A). This was expected as the TVPs are mainly comprised of pea and faba bean protein ingredients. Under reducing conditions, the bands of legumin at 60 kDa dissociated revealing new bands at around 40 kDa (legumin acidic subunit) and 20 kDa (legumin basic subunit) (Fig. 1B). Vicilin and convicilin were found under both reducing and non-reducing conditions, in the RMs and TVPs. The appearance of both convicilin and vicilin bands under non-reducing and reducing conditions in the gels of TVPs indicates that these proteins seemingly are not involved in protein aggregation occurring during extrusion.13 The legumin bands were not visible for all the TVPs under non-reducing conditions, while under reducing conditions these bands appeared, indicating that disulfide bonds were broken under these conditions. This result suggests that part of the insolubility of legumins in extrudates can be explained by the formation of disulfide bonds which induces crosslinking during extrusion. The formation of intermolecular disulfide bonds has been described as the main force involved in the structure formation during the extrusion process since the process conditions induce the breakdown of intramolecular disulfide bonds and induce the formation of intermolecular bonds.14 Similar results were observed in the high moisture extrusion of pea protein isolates.13 Besides, other molecular interactions, such as hydrophobic interactions, can be involved in protein aggregation during the extrusion process contributing to protein insolubility.15
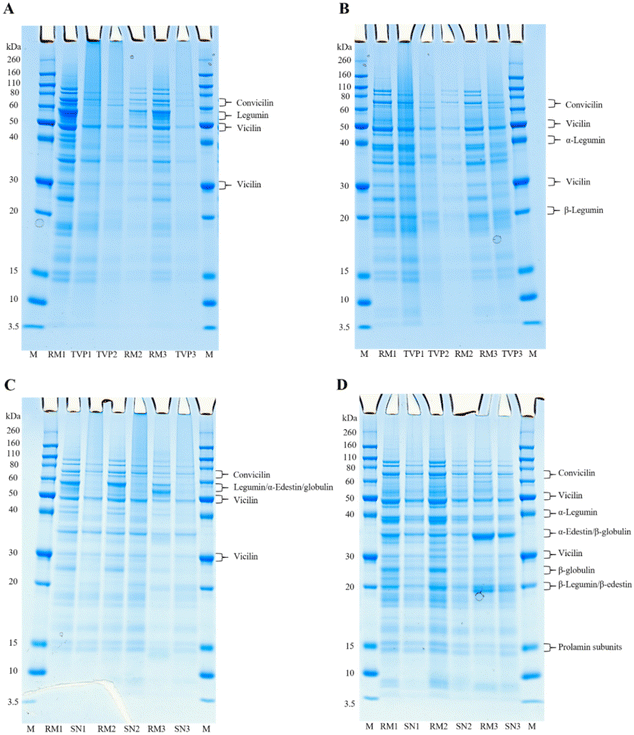 |
| Fig. 1 Molecular weight distribution of raw mixtures (RM) and extrudates, TVPs (A and B) and Snacks (C and D) obtained by Sodium Dodecylsulfate Polyacrylamide Gel Electrophoresis (SDS-PAGE) under non-reducing (A and C) and reducing conditions (B and D). M: molecular marker. The α-legumin: acidic subunit. The β-legumin: basic subunit. RM: raw mixtures. SN: snacks. Composition of RM and extrudates: TVP 1: 89% pea and 10% faba beans; TVP 2: 49% faba beans, 40% pea and 10% quinoa; TVP 3: 79% pea, 10% faba beans, 10% hemp; Snack 1: 60% oat and 39% pea; Snack 2: 50% quinoa, 39% pea and 10% oat; Snack 3: 59% oat, 20% pea, 20% hemp. | |
In Fig. 1C and D the molecular weight distribution of RMs and Snacks extrudates are displayed. Similar to the TVPs, the pea proteins convicilin and vicilin were identified in the Snacks at the same molecular weight. At the molecular level between 50 and 60 kDa for Snacks 1 and 2, the band could represent the oat globulin or the pea legumin, and in Snack 3 the band could represent the edestin from hemp protein. As all samples consist of a blend of proteins it is challenging to identify which protein is the most representative in the band. However, under reducing conditions bands between 40 and 30 kDa and between 30 and 20 kDa can be seen (Fig. 1D), which are in line with the molecular weight of the legumin subunits (β and α) from peas and the β-globulin (30–35 kDa) and α-globulin (20–25 kDa) subunits from oats, indicating that probably both proteins are present in the samples.16 There is an indication that the hemp protein edestin was present in Snack 3 before (RM) and after extrusion at the same molecular weight as legumin, as can be seen in the gels of the protein ingredients (ESI 3†). Under non-reducing conditions (Fig. 1C), the band that indicates either legumins or oat globulins is only visible in the RMs. The disappearance of this band after extrusion under non-reducing conditions suggests that these proteins were insoluble and could not penetrate the gel. Probably, these proteins were aggregated and stabilized by disulfide bonds which were disrupted under reducing conditions, as we can see in the appearance of the subunits in the reduced gel (Fig. 1D). Usually, legumins have a higher content of cysteine than vicilins which explains the formation of disulfide bonds within its subunits.17
Overall, the results from the SDS-PAGE indicated that extrusion processing could have resulted in the formation of large protein aggregates. The formation of protein aggregates can hamper the accessibility of digestive enzymes, resulting in lower protein digestibility18 which will be discussed further on the in vitro protein digestibility results.
3.3. Confocal microscopy
TVPs and Snacks were visualized using confocal microscopy before and after extrusion to study the change in microstructure (Fig. 2). Fat can be seen as lipid droplets (green) dispersed evenly through the majority of the samples. The size and distribution do not appear to change significantly after extrusion. Dietary fibers in plants are comprised of several types including cellulose, hemicellulose, lignin, and pectin.19 Calcofluor white stains β-glucans including cellulose, allowing the visualization of insoluble fibers. RMs of TVPs 1–3 and Snack 3 all contain what appear to be rigid fibers, likely crystalline cellulose, which become less apparent after extrusion. The visible fiber structures also appear larger after extrusion which could be due to the coagulation of smaller fibers during cooling post-extrusion. Heat treatment can cause the depolymerization and structural rearrangement of both cellulose and starch, glycosidic, and hydrogen bonds. Cellulose has also been shown to disrupt starch crystallization after extrusion, leading to more amorphous fibral structures.20
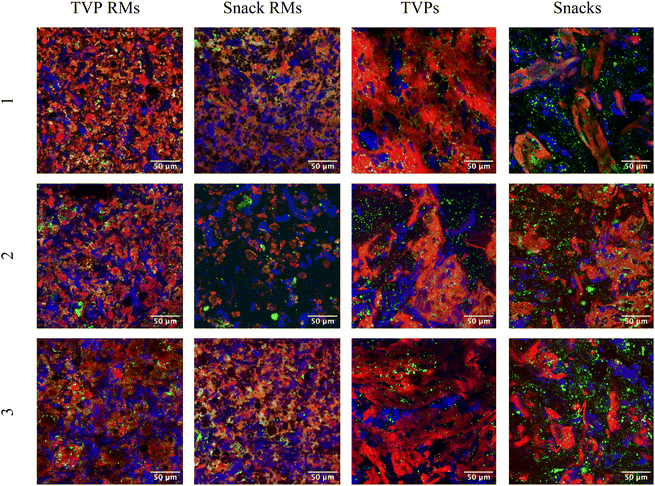 |
| Fig. 2 Confocal images of TVPs and Snacks before (left) and after (right) extrusion. Protein is stained with fast green FCF (red), lipid droplets with Nile red (green), and β-glucans with calcofluor white (blue). Composition of extrudates: TVP 1: 89% pea and 10% faba beans; TVP 2: 49% faba beans, 40% pea and 10% quinoa; TVP 3: 79% pea, 10% faba beans, 10% hemp; Snack 1: 60% oat and 39% pea; Snack 2: 50% quinoa, 39% pea and 10% oat; Snack 3: 59% oat, 20% pea, 20% hemp. | |
Proteins are stained using fast green FCF and appear more abundant in all TVP samples, as would be expected. The extrusion process changed all samples drastically and creates a matrix in both TVPs and Snacks. It has been shown that pea protein-based TVPs form a low-density, sponge-like protein matrix with a greater number of air cells (visible as black in the confocal images, Fig. 2) which is also visible in TVPs 1 + 2.21 Snacks 1 and 3, which contain a majority of oat, show less uniform protein distribution, with fewer and smaller air cells in the protein matrix. This correlates with a previous study that demonstrates the heterogeneity of protein aggregates in extruded oat.20 This could be due to swelling and gelatinization of high concentrations of carbohydrates present in Snack mixtures.
3.4. Trypsin inhibitor activity
Trypsin inhibitors are one of the ANFs present in many pulses and cereals that are responsible for the inactivation of trypsin by complexation, resulting in decreased protein digestibility in the intestinal phase.22 For this reason, methods to reduce or completely inactivate trypsin inhibitors are desirable to improve the plant protein's nutritional quality. These methods can be wet protein extraction and thermal processing of protein ingredients such as extrusion and cooking. In terms of protein extraction, wet extraction is usually more efficient to reduce trypsin inhibitor activity than dry fractionation which often leads to an accumulation of ANFs in the protein fraction due to the low molecular weight of the trypsin inhibitors.3,23 In this study, the protein ingredients used were obtained by dry fractionation and it can be expected that the extrusion process will reduce considerably the amount of these ANFs. The trypsin inhibitor activity (TIA) of the protein ingredients, TVPs, and Snacks before (RM) and after extrusion is shown in Table 3.
Table 3 The trypsin inhibitor activity (TIA) of protein ingredients, TVPs, and snacks
Protein ingredients/extrudates |
TIU per mg sample (dry basis) |
TIU per mg protein |
TIU reductiona (%) |
The results are shown as mean ± standard deviation of three independent replicates. Values within the same column with different letter superscripts are significantly different (p < 0.05). Reduction (%) indicates the decrease in TIU per mg sample (dry basis) after extrusion. <DL = below-known detection limits. NA: not applicable. RM: raw mixtures. |
Pea protein |
7.59 ± 0.16b |
15.72 ± 0.33b |
NA |
Faba bean protein |
10.94 ± 0.26a |
19.23 ± 0.46a |
NA |
Hemp protein |
5.32 ± 0.12c |
10.64 ± 0.23e |
NA |
Quinoa flour |
0.61 ± 0.11g |
5.47 ± 0.97f |
NA |
Oat flour |
<DL |
<DL |
NA |
|
TVP 1 RM |
7.35 ± 0.23b |
15.11 ± 0.47bc |
|
TVP 1 |
1.93 ± 0.13e |
3.78 ± 0.26g |
74 |
TVP 2 RM |
7.89 ± 0.04b |
16.33 ± 0.09b |
|
TVP 2 |
1.76 ± 0.25e |
3.37 ± 0.48g |
78 |
TVP 3 RM |
7.39 ± 0.15b |
15.15 ± 0.30bc |
|
TVP 3 |
1.56 ± 0.11e |
3.03 ± 0.21g |
79 |
|
Snack 1 RM |
3.57 ± 0.06d |
14.05 ± 0.23cd |
|
Snack 1 |
0.94 ± 0.09f |
3.40 ± 0.31g |
74 |
Snack 2 RM |
3.34 ± 0.06d |
13.11 ± 0.22d |
|
Snack 2 |
0.99 ± 0.03f |
3.42 ± 0.11g |
71 |
Snack 3 RM |
3.54 ± 0.06d |
13.57 ± 0.22d |
|
Snack 3 |
1.02 ± 0.04f |
3.68 ± 0.16g |
71 |
Regarding the protein ingredients, faba bean protein showed the significantly highest level of TIA (19.23 ± 0.46 TIU per mg protein), followed by pea protein (15.72 ± 0.33 TIU per mg protein), whereas quinoa and oat had the lowest TIA among all protein ingredients. The TIA level in oat proteins was below the detection range of the method.9 The discrepant difference in TIA levels observed in the protein ingredients was expected since pulses are known to have higher TIA than cereals.24
Comparing our results with the literature is a difficult task because there is great variability in the TIA levels reported for protein ingredients. This is explained by the varying methods and conditions used, such as enzyme activity, and the units used to express TIA in tested samples.25 For this reason, here we only compare our results based on TIU per mg of sample (dry basis) or mg of proteins. The results of faba bean and pea protein observed here were higher than those reported in the literature with values ranging from 2.31 to 7.20 TIU per mg protein for faba bean and 0.78 to 6.32 TIU per mg protein for field pea.22
Extrusion is a high-temperature short-time process that involves heating and shearing, resulting in a texturized final product. This process has been described to reduce or inactivate the protease inhibitor activities depending on the process conditions applied, such as moisture content of the feed, barrel temperature, and screw speed.24 The reduction of protease inhibitors during extrusion can be explained by the application of temperature and high shear forces that can physically deform the proteins, resulting in denaturation of the trypsin inhibitors by modification of the protein structure.26
For both TVPs and Snacks, the TIU per mg protein was significantly lower after extrusion compared to the RMs. A reduction in TIA of 71–79% was shown for all samples. Higher levels of TIA reduction in peas have been reported such as a 90% reduction after extrusion at 129 °C and total inactivation at 135 and 142 °C.27 However, the authors found much lower initial TIA in the raw pea (1.84 ± 0.15 TIU per mg dry basis). Many variables can contribute to different starting levels of TIA in the raw seeds, such as detection methods, cultivars, and agronomic conditions (e.g., climate and soil).28 In this study, the remaining trypsin inhibitors that could not be inactivated must be thermostable since it was not possible to completely inactivate trypsin inhibitors, even though the extrusion processing reached temperatures above 130 °C. Frias et al. (2011)27 showed that at 135 °C and 142 °C trypsin inhibitors were inactivated in pea extrudates. The difference can be explained by extrusion parameters, in which inactivation of trypsin inhibitors can be reached at high temperatures or at increased residence time at low temperatures.29 Hejdysz et al. (2022)30 also described a reduction in TIA after the extrusion of faba beans compared to the raw beans but did not find any additional reduction in trypsin inhibitor at temperatures above 110 °C, suggesting that the remaining trypsin inhibitor were more thermostable. The thermostability of trypsin inhibitors can be explained by the difference in the protein structure of the two types of trypsin inhibitors: Kunitz type which contains 2 disulfide bonds and the Bowman–Birk type which contains 7 disulfide bonds. The presence of these bonds is known to maintain the structural stability of the protein.
Comparing TVPs 1–3 and Snacks 1–3 no significant difference was observed in the TIA levels among the three TVPs and the three Snacks, despite the difference in their ingredients. However, Snacks had a lower TIA level than the TVPs, which goes in line with the fact that TVPs had more than 79% of pulses in their composition. As can be seen in Table 3, the legume ingredients had higher TIA than the cereals and pseudocereals.
Therefore, the extrusion process applied in this study as means to reduce trypsin inhibitors in the protein blends was accomplished. The inactivation of trypsin inhibitors is desirable as it is expected to increase the protein digestibility of the TVPs and Snacks, by allowing greater trypsin activity in the intestinal phase. A higher reduction in the TIA could be accomplished by changing extrusion parameters, combining pretreatment of the dry fractionated ingredients, such as heating or fermentation, or by cooking methods.7
3.5.
In vitro protein digestibility (IVPD)
The IVPD results give an indication of the extent of protein digestibility by pepsin in the gastric phase, and by pancreatin in the intestinal phase. The total digestibility represents the digestion in the gastric and intestinal phases combined. Table 4 outlines the IVPD results for RMs and TVPs.
Table 4
In vitro protein digestibility of the raw mixtures (RM) and TVPs after 1 h pepsin hydrolysis, 1 h pancreatin hydrolysis, and total protein digestibility (2 h)
Extrudates |
Pepsin digestibility (%) (1 h) |
Pancreatin digestibility (%) (1 h) |
Total digestibility (%) (2 h) |
The results are shown as mean ± standard deviation of three independent replicates. Values within the same column with different letter superscripts are significantly different (p < 0.05). BSA: bovine serum albumin. RM: raw mixtures. Composition: TVP 1 = 10% faba bean, 89% pea. TVP 2 = 49% faba bean, 40% pea, 10% quinoa. TVP 3 = 10% faba bean, 79% pea, 10% hemp. |
TVP 1 RM |
4.17 ± 0.15bc |
13.41 ± 0.19d |
17.58 ± 0.32c |
TVP 1 |
3.88 ± 0.06c |
17.07 ± 0.34ab |
20.94 ± 0.30b |
TVP 2 RM |
4.54 ± 0.16b |
16.14 ± 0.78bc |
20.68 ± 0.90b |
TVP 2 |
3.80 ± 0.18c |
17.64 ± 1.01ab |
21.44 ± 1.09b |
TVP 3 RM |
3.91 ± 0.18c |
14.34 ± 1.34cd |
18.25 ± 1.51c |
TVP 3 |
3.85 ± 0.22c |
18.67 ± 0.61a |
22.52 ± 0.58b |
BSA reference |
6.44 ± 0.09a |
18.77 ± 0.19a |
25.21 ± 0.22a |
Only the TVP 2 showed a significant reduction in pepsin digestibility after extrusion compared to the RM. This could be explained by the formation of protein aggregates induced by extrusion which reduces the accessibility for digestive enzymes, as observed in the SDS-PAGE under reducing conditions (Fig. 1B). Regarding the intestinal phase, TVP 1 RM (13.41% ± 0.19) and TVP 3 RM (14.34% ± 1.34) showed the lowest pancreatin digestibility. However, a significant increase in digestibility was detected for the respective extrudates, TVP 1 (17.07% ± 0.34) and TVP 3 (18.67% ± 0.61). This is likely caused by the reduction in trypsin inhibitor activity after extrusion, 74% and 79% respectively (Table 3), allowing greater protein digestion in the intestinal phase. Therefore, the significant increase in the total digestibility of TVP 1 (19% increase) and TVP 3 (23% increase) compared to the RMs can be attributed to the reduction in trypsin inhibitors. This trend agrees with the results of Qi et al. (2021)31 who found an increase of 12% in the protein digestibility of pea extrudates processed at 90 °C compared to the raw pea flour. The higher % increase observed in our study can be attributed to a difference in the level of trypsin inhibitor in the flour, and the extrusion conditions, since we used a higher extrusion temperature. However, no significant difference was observed for TVP 2 before and after extrusion, probably related to the presence of protein aggregates offsetting the positive effect of lower trypsin inhibitor activity.
The extrusion process can result in protein aggregation depending on the temperature applied which can limit the digestive enzyme accessibility, thereby reducing protein digestibility.32 Our results suggest a positive effect of the extrusion processing method on the nutritional quality of TVPs, regarding protein digestibility. However, compared to highly digestible animal protein, BSA reference, all TVPs still showed a lower total protein digestibility. This result was expected since extrusion can improve the digestibility of proteins by protein denaturation and a reduction in enzyme inhibitors, but the extent of the improvement depends on the food matrix and the type of proteins under consideration, as well as extrusion conditions.33
The IVPD results of the Snacks are outlined in Table 5. For all the Snacks, pepsin digestibility was significantly reduced after extrusion. As described earlier, this reduction in protein digestibility can be attributed to the formation of protein aggregates which are less accessible to protease enzymes34 (Fig. 1D). Moreover, the presence of fiber and starch in the Snacks, due to their higher content in cereals/pseudocereals, combined with the protein structural changes induced by extrusion may have played a role in the low protein digestibility observed. Opazo-Navarrete et al. (2019)35 showed that the incorporation of starch into quinoa protein isolates heated at 120 °C resulted in a larger decrease in the protein digestibility in the gastric phase after 180 min than in the protein isolate combined with starch and fiber, and in the heated dry fractionated quinoa concentrate. The authors explained that heating the mixture of protein isolate containing partially denatured protein, due to the wet fractionation process, and starch resulted in extensive protein aggregation and starch gelatinization which hinders the penetration of pepsin into the matrix and enzyme accessibility to proteins, resulting in very low protein digestibility. Moreover, it has been shown that the presence of amylose and/or amylopectin can affect protein digestibility positively or negatively. Chen et al. (2021)36 showed that adding amylopectin to pea protein high-moisture extrudates decreased the in vitro protein digestibility compared to pea protein extrudate. On the contrary, adding amylose to amylopectin at different ratios improved the protein digestibility compared to amylopectin alone. The authors suggested that amylopectin could result in protein aggregation, therefore, limiting protein digestibility.
Table 5
In vitro protein digestibility (%) of the raw mixtures (RM) and Snacks extrudates after 1 h pepsin hydrolysis, 1 h pancreatin hydrolysis, and total protein digestibility
Extrudates |
Pepsin digestibility (%) (1 h) |
Pancreatin digestibility (%) (1 h) |
Total digestibility (%) (2 h) |
The results are shown as mean ± standard deviation of three independent replicates. Values within the same column with different letter superscripts are significantly different (p < 0.05). BSA: bovine serum albumin. RM: raw mixtures. Composition: Snack 1 = 39% pea, 60% oat. Snack 2 = 39% pea, 50% quinoa, 10% oat. Snack 3 = 20% pea, 20% hemp, 59% oat. |
Snack 1 RM |
3.66 ± 0.09b |
13.58 ± 0.33bc |
17.24 ± 0.40b |
Snack 1 |
2.55 ± 0.08c |
15.63 ± 1.10b |
18.19 ± 1.05b |
Snack 2 RM |
4.02 ± 0.35b |
14.92 ± 0.44b |
18.94 ± 0.18b |
Snack 2 |
2.40 ± 0.04c |
15.14 ± 0.69b |
17.54 ± 0.70b |
Snack 3 RM |
2.77 ± 0.04c |
10.64 ± 0.50d |
13.41 ± 0.47c |
Snack 3 |
1.56 ± 0.36d |
12.36 ± 1.31cd |
13.93 ± 1.67c |
BSA reference |
6.79 ± 0.09a |
20.50 ± 0.19a |
27.28 ± 0.17a |
Regarding pancreatin digestibility, it seemed that protein digestibility increased after extrusion, however, the increase was not significant. Even though there was a significant reduction in the TIA after extrusion, the initial TIA in the RMs was already low. Thereby, the impact of TIA reduction in the protein digestibility of Snacks during the intestinal phase was not the same as seen for the TVPs. Likewise, Carbonaro et al. (2000)37 also showed that thermal treatment only had a slightly positive effect on the digestibility of common bean proteins. The thermal inactivation of the high amount of ANFs present in the beans was counterbalanced by the negative effect of heating on protein digestibility. However, we must consider that different raw materials, with different protein compositions and TIA levels, will result in different protein digestibility.
When comparing the IVPD of all TVPs and Snacks to the BSA reference, BSA had a higher protein digestibility compared to all samples (Tables 4 and 5). This result was expected as BSA is a highly digestible protein.38 To allow comparison of all TVPs and Snacks, the total IVPD was calculated relative to the protein digestibility of BSA as illustrated in Fig. 3. Overall, the snacks showed a lower IVPD compared to the TVPs. This can be attributed to the greater starch content in these samples which had higher carbohydrate content than the TVPs. In addition, the protein matrix in the Snacks (Fig. 2) was less uniformly distributed and less sponge-like as seen in the TVP extrudates, which may have contributed to hindering the penetration of the digestive enzymes and their accessibility to the proteins. The protein and starch matrix can reduce the accessibility of digestive enzymes to peptide bonds and thereby reducing protein digestibility.35,39
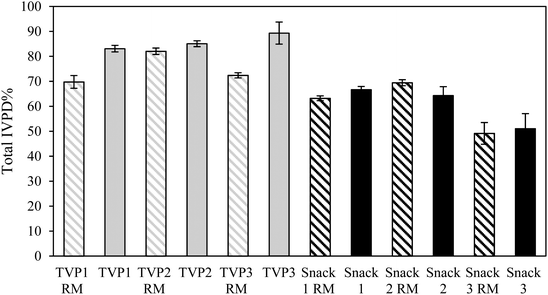 |
| Fig. 3 The total in vitro protein digestibility (IVPD%) of TVPs (grey bars) and Snacks (black bars) before (patterned bars) and after extrusion (solid bars) relative to the total IVPD% of BSA as a reference. Total IVPD represents the mean of 2 h protein digestion results (pepsin + pancreatin digestibility). RM: raw mixtures. BSA: bovine serum albumin. | |
Overall, the extrusion process did not seem to improve the total protein digestibility of Snacks, as the IVPD of the RM and extrudates did not differ significantly. Interestingly, Snack 3 before and after extrusion showed a significantly lower total protein digestibility compared to all other snacks. This might indicate an effect of the food matrix composition on protein digestibility since the hemp protein had the highest fiber content among all protein ingredients (ESI 1†). The presence of intact cell walls (i.e. dietary fiber) in whole beans/seeds, protein flours, and less processed protein ingredients can restrict the contact between proteins and digestive enzymes and enzyme mobility, therefore reducing the digestibility and absorption of proteins.40 Generally, the food matrix composition (i.e. the presence of starch, fiber, and ANFs) has been associated with a negative or no effect on in vitro protein digestibiliy.41 Studies have shown that the presence of soluble or insoluble dietary fiber decreased in vitro protein digestibility of plant protein sources.35,42,43
3.6. Amino acid profile and score
The amino acid composition and amino acid score for all extrudates were determined and a theoretical calculation was done to estimate the amino acid composition of the RMs, according to the amino acid composition of the individual protein ingredients (ESI 4†) to understand the effect of extrusion on amino acid loss. The amino acid score indicates the level of the limiting amino acid which is present at the lowest levels concerning human requirements. An amino acid score greater than 100 represents a complete protein source containing all IAAs at adequate levels required for human metabolism. A value less than 100 indicates that one or more of the IAAs is present at levels below the human requirements.12
The amino acid content and score of the TVPs before and after extrusion are shown in Table 6. The results of the theoretical calculation for the RM showed that among the TVPs, TVP 3 was the only extrudate with an amino acid score greater than 100 before extrusion. This proves that the higher content of pea protein in the RM of TVP 3 contributed to obtaining a balanced amino acid composition (for the amino acid content and score of pea protein see Table 2 in ESI 4†). Meanwhile, the higher content of faba bean protein in the RM of TVP 2 contributed to the lower amino acid score compared to the other RMs of TVPs. The sulfur-containing amino acids appeared to be the limiting amino acids for all TVPs. This result was expected since all TVPs were mainly comprised of pea and faba proteins which have a lower methionine and cysteine content compared to the cereals and pseudocereals. After the extrusion process, a reduction in the amino acid score was observed for all TVPs. However, TVP 3 was found to have the largest reduction in the amino acid score (from 106 to 95) despite the complete amino acid profile before extrusion.
Table 6 Amino acid composition and scores of TVPs before (raw mixture) and after extrusion. The lowest amino acid score is in bold
|
TVP 1 RM |
TVP 1 |
TVP 2 RM |
TVP 2 |
TVP 3 RM |
TVP 3 |
Indispensable amino acid |
Amino acid contenta |
Amino acid scorea |
Amino acid contentb |
Amino acid scoreb |
Amino acid contenta |
Amino acid scorea |
Amino acid contentb |
Amino acid scoreb |
Amino acid contenta |
Amino acid scorea |
Amino acid contentb |
Amino acid scoreb |
(mg per g protein) |
(mg per g protein) |
(mg per g protein) |
(mg per g protein) |
(mg per g protein) |
(mg per g protein) |
(mg per g protein) |
(mg per g protein) |
(mg per g protein) |
(mg per g protein) |
(mg per g protein) |
(mg per g protein) |
Theoretical amino acid content based on the composition of the individual raw mixtures (ESI 4†).
Actual amino acid content. RM: raw mixture. Composition: TVP 1: 89% pea and 10% faba beans; TVP 2: 49% faba beans, 40% pea and 10% quinoa; TVP 3: 79% pea, 10% faba beans, 10% hemp.
|
Histidine |
25.86 |
171 |
25.21 |
166 |
26.89 |
177 |
26.68 |
176 |
26.03 |
172 |
26.84 |
177 |
Isoleucine |
43.15 |
142 |
44.27 |
146 |
43.19 |
143 |
44.86 |
148 |
42.86 |
141 |
44.59 |
147 |
Leucine |
76.84 |
130 |
81.13 |
137 |
79.73 |
135 |
77.87 |
132 |
75.97 |
129 |
79.69 |
135 |
Lysine |
79.49 |
175 |
76.68 |
169 |
73.32 |
161 |
72.92 |
160 |
75.63 |
166 |
73.49 |
162 |
Methionine |
9.50 |
|
9.32 |
|
8.85 |
|
8.70 |
|
10.94 |
|
9.70 |
|
Cysteine |
12.69 |
|
11.86 |
|
12.36 |
|
11.86 |
|
13.11 |
|
11.97 |
|
Methionine + cysteine |
22.19 |
98
|
21.18 |
93
|
21.20 |
93
|
20.55 |
90
|
24.06 |
106
|
21.68 |
95
|
Phenylalanine |
51.64 |
|
48.62 |
|
48.15 |
|
48.62 |
|
51.12 |
|
49.34 |
|
Tyrosine |
37.00 |
|
35.57 |
|
36.84 |
|
35.57 |
|
36.89 |
|
35.92 |
|
Phenylalanine + tyrosine |
88.65 |
234 |
84.19 |
222 |
84.99 |
224 |
84.19 |
222 |
88.02 |
232 |
85.26 |
225 |
Threonine |
39.38 |
173 |
36.96 |
163 |
38.27 |
168 |
36.96 |
163 |
38.99 |
172 |
37.78 |
166 |
Tryptophan |
8.95 |
148 |
8.70 |
143 |
9.06 |
149 |
8.70 |
143 |
9.20 |
152 |
9.50 |
157 |
Valine |
46.39 |
118 |
48.02 |
122 |
46.48 |
118 |
48.02 |
122 |
46.56 |
118 |
47.07 |
119 |
The amino acid content and score of the Snacks are outlined in Table 7. Before extrusion, all Snacks had an amino acid score greater than 100, which shows that the complementation of pulses, cereals, and pseudocereals resulted in a complete protein source. Unlike the TVPs, the Snacks did not show a lower amino acid score compared to their RMs. Surprisingly, all Snacks retained an amino acid score greater than 100 after the extrusion process. These results showed that using a higher content of cereals/pseudocereals in the protein blends outcomes in a sufficient level of IAAs. This may be due to the inclusion of higher content of oats, quinoa, and hemp in the Snacks providing more sulfur-containing amino acids since those were not the limiting ones in these protein sources (ESI 4†). Interestingly, Snack 3 showed a higher content of sulfur-containing amino acids and lower lysine content compared to the other two Snacks. This is likely attributed to its composition containing a higher content of oat (59%) and hemp (20%) and a lower content of peas (20%), compared to Snack 1 (60% oat and 39% pea) and Snack 2 (50% quinoa, 39% pea, and 10% oat), providing more methionine and cysteine and less lysine due to the latter being the limiting amino acid in oat and hemp. The potential of blending plant proteins is well described in literature since cereal-based proteins that score low in lysine but high in methionine and cysteine to a certain extent can complement proteins obtained from pulses, which are high in lysine but low in sulfur-containing amino acids.44 Moreover, the degree of complementarity will depend on the ratio of combined protein sources.45
Table 7 Amino acid composition and scores of Snacks before (raw mixture) and after extrusion. The lowest amino acid score is in bold
|
Snack 1 RM |
Snack 1 |
Snack 2 RM |
Snack 2 |
Snack 3 RM |
Snack 3 |
Indispensable amino acid |
Amino acid contenta |
Amino acid scorea |
Amino acid contentb |
Amino acid scoreb |
Amino acid contenta |
Amino acid scorea |
Amino acid contentb |
Amino acid scoreb |
Amino acid contenta |
Amino acid scorea |
Amino acid contentb |
Amino acid scoreb |
(mg per g protein) |
(mg per g protein) |
(mg per g protein) |
(mg per g protein) |
(mg per g protein) |
(mg per g protein) |
(mg per g protein) |
(mg per g protein) |
(mg per g protein) |
(mg per g protein) |
(mg per g protein) |
(mg per g protein) |
Theoretical amino acid content based on the composition of the individual raw mixtures (ESI 4†).
Actual amino acid content. RM: raw mixture. Composition: Snack 1: 60% oat and 39% pea; Snack 2: 50% quinoa, 39% pea and 10% oat; Snack 3: 59% oat, 20% pea, 20% hemp.
|
Histidine |
24.86 |
164 |
24.63 |
163 |
26.73 |
176 |
26.91 |
178 |
25.51 |
168 |
25.83 |
170 |
Isoleucine |
42.21 |
139 |
44.12 |
146 |
42.51 |
140 |
44.00 |
145 |
41.10 |
136 |
42.68 |
141 |
Leucine |
76.33 |
129 |
75.74 |
128 |
75.21 |
127 |
76.00 |
129 |
72.91 |
123
|
75.63 |
128 |
Lysine |
71.30 |
157 |
71.32 |
157 |
75.84 |
167 |
73.82 |
162 |
56.28 |
124 |
59.53 |
131 |
Methionine |
11.79 |
|
11.76 |
|
13.59 |
|
13.45 |
|
17.42 |
|
16.47 |
|
Cysteine |
17.60 |
|
17.28 |
|
15.25 |
|
14.91 |
|
19.27 |
|
18.35 |
|
Methionine + cysteine |
29.38 |
129 |
29.04 |
128 |
28.85 |
127 |
28.36 |
125
|
36.69 |
161 |
34.82 |
153 |
Phenylalanine |
52.28 |
|
51.84 |
|
50.81 |
|
51.64 |
|
50.28 |
|
50.54 |
|
Tyrosine |
36.70 |
|
34.56 |
|
36.17 |
|
34.91 |
|
36.27 |
|
34.07 |
|
Phenylalanine + tyrosine |
88.98 |
235 |
86.40 |
228 |
86.98 |
230 |
86.55 |
228 |
86.54 |
228 |
84.61 |
223 |
Threonine |
38.44 |
169 |
37.50 |
165 |
39.60 |
174 |
37.45 |
165 |
36.91 |
162 |
36.32 |
160 |
Tryptophan |
10.47 |
173 |
10.29 |
170 |
10.40 |
172 |
10.18 |
168 |
11.47 |
189 |
11.61 |
192 |
Valine |
47.71 |
121
|
50.00 |
127
|
47.10 |
120
|
50.18 |
127 |
48.37 |
123
|
50.17 |
127
|
Fig. 4 illustrates the percentage of reduction in IAAs after the extrusion of the TVPs and Snacks. The results highlight that not all IAAs are lost during the extrusion process and that the loss of certain IAAs seems to depend on the extrudate composition and extrusion process. In TVP 1, TVP 3, and Snack 3 (Fig. 4A, C, and F), there was a greater loss in sulfur-containing amino acids compared to the other extrudates. In addition, in Snack 1 and Snack 3 (Fig. 4D, F) we noticed a slightly more pronounced decrease in tyrosine than in the other extrudates. However, the loss of these IAAs should not be a matter of concern, especially for the Snacks that maintained an amino acid score above 100 after extrusion. Frias et al. (2011) also found a decrease in the sulfur-containing amino acids after extrusion and that the amino acid score varied according to the extrusion temperature, from 80 in raw peas to 73 and 69 after extrusion at 135 °C and 142 °C, respectively.27 The loss of amino acids after extrusion can be explained by oxidation reactions such as the Maillard reaction or protein oxidation.45 Among all IAAs, lysine is the most sensitive to Maillard reaction under high temperatures and low feed moisture extrusion.45 Free sugars can be provided from the hydrolysis of starch during extrusion to react with lysine and other amino acids.45 Yet, since the reduction of methionine and cysteine is much greater than that of lysine, it rather suggests the oxidation of these amino acids during the extrusion process. In proteins, all amino acid residues are prone to be oxidized by different reactive oxygen species (ROS), especially methionine and cysteine residues due to their increased sensibility to ROS.46 Methionine is oxidized to methionine sulfoxide during food processing and to a less extent to methionine sulfone, only under rigorous oxidation conditions.47 Cysteine can also be oxidized into various products, such as disulfide, sulfenic acid, sulfonic acid, and sulfinic acid.48 The bioavailability of these oxidized forms of both methionine and cysteine depends on their degree of oxidation. Methionine sulfone and cysteic acid are not bioavailable, but methionine sulfoxide can be utilized to some degree.49 This highlights that oxidation occurring during extrusion can potentially affect the protein's nutritional quality due to the loss of IAAs.
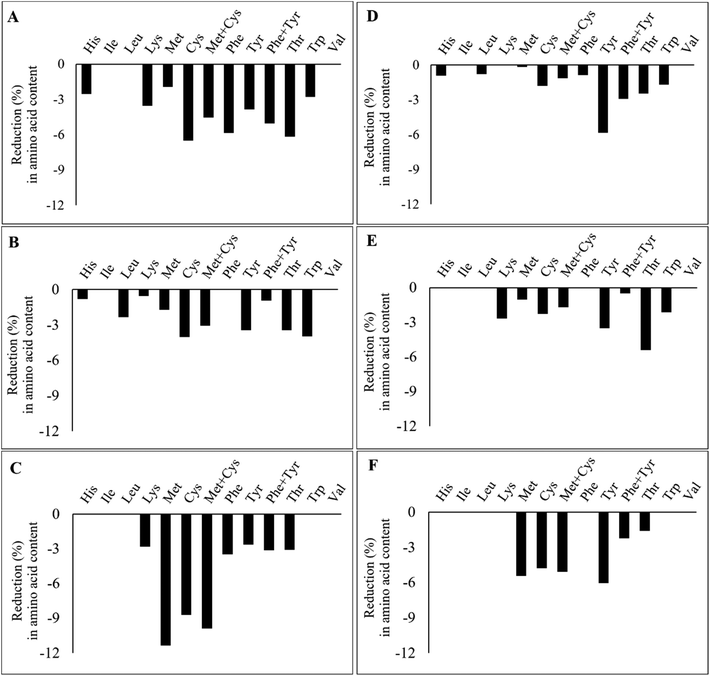 |
| Fig. 4 Percentage reduction (%) in indispensable amino acid content after extrusion of TVPs 1–3 (A–C) and Snacks 1–3 (D–F) compared to the raw material composition. His: histidine; Ile: isoleucine; Leu: leucine; Lys: lysine; Met: methionine; Cys: cysteine; Phe: phenylalanine; Try: tyrosine; Thr: threonine; Trp: tryptophan; Val: valine. | |
4. Conclusion
In this study, we aimed to investigate the effect of blending different protein sources to improve the IAAs content combined with the application of extrusion to improve protein digestibility by reduction of TIA. Based on our results, the extrusion process led to a reduced level of TIA by greater than 71% in all extrudates. However, we only observed an increase in total protein digestibility (gastric and intestinal stages) in TVPs, most likely associated with the reduction of TIA. Moreover, the results highlighted that extrusion processing caused the loss of certain IAAs, especially the sulfur-containing amino acids in TVPs. However, the effect of IAAs on the overall nutritional quality of the TVPs may be balanced out by the desirable reduction in TIA and increase in IVPD. A potential solution to ensure a complete protein source is to incorporate the TVPs in a meal containing cereals (e.g., rice, bread, pasta) to boost the methionine and cysteine content while obtaining a rich, digestible protein source from the extrudate. The reduction in TIA is also hugely beneficial for the nutritional quality of the meal, as it would be expected to have a positive effect on the overall protein digestibility. Regarding the Snacks, their IAAs composition was less negatively affected by extrusion as they retained a balanced amino acid composition, despite the loss of certain IAAs. However, the Snack extrudates showed a lower protein content and lower protein digestibility compared to the TVPs. Therefore, a larger portion of the Snacks would be required to achieve an equivalent protein intake. Overall, the extrusion process has the potential to enhance the nutritional quality of plant protein blends.
Author contributions
Patrícia Duque-Estrada: conceptualization, validation, visualization, supervision, writing – original draft; Kate Hardiman: formal analysis, visualization, investigation, writing – original draft; Astrid Bøgebjerg Dam: formal analysis, visualization,Nadia Dodge: formal analysis, visualization, and writing – original draft & review; Margit Dall Aaslyng: conceptualization, supervision, funding acquisition, project administration, resources, writing – review & editing; Iben Lykke Petersen: conceptualization, project administration, supervision, resources, writing – review & editing.
Conflicts of interest
There are no conflicts to declare.
Acknowledgements
This research was funded by the program Grønt Udviklings og Demonstrationsprogram (GUDP) from the Ministeriet for Fødevarer, Landbrug og Fiskeri of Denmark, under grant agreement no. 34009-20-1662. We would like to thank the Quinoa Quality A/S (Regstrup, DK) and Dava Foods A/S (Hadsund, DK) for kindly providing the protein ingredients and the Organic Plant Protein A/S (Hedensted, DK) for providing the extrudates. Furthermore, we thank the Center for Advanced Bioimaging (CAB) Denmark, University of Copenhagen, where the CLSM images were taken.
References
- P. Joseph, A. Searing and C. Watson,
et al., Alternative proteins: market research on consumer trends and emerging landscape, Meat Muscle Biol., 2020, 4, 1–11 CrossRef.
- S. Adhikari, M. Schop and I. J. M. de Boer,
et al., Protein quality in perspective: a review of protein quality metrics and their applications, Nutrients, 2022, 14, 947 CrossRef CAS PubMed.
- A. Amin, I. L. Petersen and C. Malmberg,
et al., Perspective on the effect of protein extraction method on the antinutritional factor (ANF) content in seeds, ACS Food Sci. Technol., 2022, 2, 604–612 CrossRef CAS.
- A. Iqbal, I. A. Khalil and N. Ateeq,
et al., Nutritional quality of important food legumes, Food Chem., 2006, 97, 331–335 CrossRef CAS.
- A. G. A. Sá, Y. M. F. Moreno and B. A. M. Carciofi, Plant proteins as high-quality nutritional source for human diet, Trends Food Sci. Technol., 2020, 97, 170–184 CrossRef.
- L. Alvarez-Jubete, E. K. Arendt and E. Gallagher, Nutritive value of pseudocereals and their increasing use as functional gluten-free ingredients, Trends Food Sci. Technol., 2010, 21, 106–113 CrossRef CAS.
- S. Avilés-Gaxiola, C. Chuck-Hernández and S. O. Serna Saldívar, Inactivation methods of trypsin inhibitor in legumes: A Review, J. Food Sci., 2018, 83, 17–29 CrossRef PubMed.
- M. Cotacallapa-Sucapuca, E. N. Vega and H. A. Maieves,
et al., Extrusion process as an alternative to improve pulses products consumption. A Review, Foods, 2021, 10, 1096 CrossRef CAS PubMed.
- K. Liu, S. Seegers and W. Cao,
et al., An international collaborative study on trypsin inhibitor assay for legumes, cereals, and related products, J. Am. Oil Chem. Soc., 2021, 98, 375–390 CrossRef CAS.
- K. Liu, Soybean trypsin inhibitor assay: further improvement of the standard method approved and reapproved by American Oil Chemists’ Society and American Association of Cereal Chemists International, J. Am. Oil Chem. Soc., 2019, 96, 635–645 CrossRef CAS.
- M. S. Joehnke, A. Rehder and S. Sørensen,
et al., In vitro digestibility of rapeseed and bovine whey protein mixtures, J. Agric. Food Chem., 2018, 66, 711–719 CrossRef CAS PubMed.
- WHO/FAO/UNU, Protein and Amino Acid Requirements in Human Nutrition, Report of a Joint WHO/FAO/UNI Expert Consultation, 2007, https://apps.who.int/iris/handle/10665/43411.
- R. Osen, S. Toelstede and P. Eisner,
et al., Effect of high moisture extrusion cooking on protein-protein interactions of pea (Pisum sativum L.) protein isolates, Int. J. Food Sci. Technol., 2015, 50, 1390–1396 CrossRef CAS.
- B. Arora, A. Yoon and M. Sriram,
et al., Reactive extrusion: A review of the physicochemical changes in food systems, Innovative Food Sci. Emerging Technol., 2020, 64, 102429 CrossRef CAS.
- O. K. Mosibo, G. Ferrentino and M. R. Alam,
et al., Extrusion cooking of protein-based products: potentials and challenges, Crit. Rev. Food Sci. Nutr., 2020, 62, 2526–2547 CrossRef PubMed.
- O. A. Sánchez-Velázquez, E. O. Cuevas-Rodríguez and M. Mondor,
et al., Impact of in vitro gastrointestinal digestion on peptide profile and bioactivity of cooked and non-cooked oat protein concentrates, Curr. Res. Food Sci., 2021, 4, 93–104 CrossRef PubMed.
- K. Shevkani, N. Singh and Y. Chen,
et al., Pulse proteins: secondary structure, functionality and applications, J. Food Sci. Technol., 2019, 56, 2787–2798 CAS.
- L. Kaur, B. Mao and A. S. Beniwal,
et al., Alternative proteins vs animal proteins: The influence of structure and processing on their gastro-small intestinal digestion, Trends Food Sci. Technol., 2022, 122, 275–286 CrossRef CAS.
- D. Dhingra, M. Michael and H. Rajput,
et al., Dietary fibre in foods: a review, J. Food Sci. Technol., 2012, 49, 255 CrossRef CAS PubMed.
- P. Ek, B. J. Gu and S. R. Saunders,
et al., Exploration of physicochemical properties and molecular interactions between cellulose and high-amylose cornstarch during extrusion processing, Curr. Res. Food Sci., 2021, 4, 588–597 CrossRef CAS PubMed.
- D. R. Cremer and G. Kaletunç, Fourier transform infrared microspectroscopic study of the chemical microstructure of corn and oat flour-based extrudates, Carbohydr. Polym., 2003, 52, 53–65 CrossRef CAS.
- Y. Kumar, S. Basu and D. Goswami,
et al., Anti-nutritional compounds in pulses: Implications and alleviation methods, Legume Sci., 2022, 4, e111 CAS.
- M. Vogelsang-O’Dwyer, I. L. Petersen and M. S. Joehnke,
et al., Comparison of faba bean protein ingredients produced using dry fractionation and isoelectric precipitation: techno-functional, nutritional and environmental performance, Foods, 2020, 9, 1–24 Search PubMed.
- N. Nikmaram, S. Y. Leong and M. Koubaa,
et al., Effect of extrusion on the anti-nutritional factors of food products: An overview, Food Control, 2017, 79, 62–73 CrossRef CAS.
- K. Liu, Trypsin inhibitor assay: expressing, calculating, and standardizing inhibitor activity in absolute amounts of trypsin inhibited or trypsin inhibitors, J. Am. Oil Chem. Soc., 2021, 98, 355–373 CrossRef CAS.
- S. Avilés-Gaxiola, C. Chuck-Hernández and S. O. Serna Saldívar, Inactivation methods of trypsin inhibitor in legumes: A Review, J. Food Sci., 2018, 83, 17–29 CrossRef PubMed.
- J. Frias, S. Giacomino and E. Peñas,
et al., Assessment of the nutritional quality of raw and extruded Pisum sativum L. var. laguna seeds, LWT – Food Sci. Technol., 2011, 44, 1303–1308 CrossRef CAS.
- D. Duijsens, S. Gwala and A. P. Pallares,
et al., How postharvest variables in the pulse value chain affect nutrient digestibility and bioaccessibility, Compr. Rev. Food Sci. Food Saf., 2021, 20, 5067–5096 CrossRef CAS PubMed.
- A. Pasqualone, M. Costantini and T. E. Coldea,
et al., Use of legumes in extrusion cooking: A Review, Foods, 2020, 9, 1–17 Search PubMed.
- M. Hejdysz, S. A. Kaczmarek and M. R. Bedford, The effect of different temperatures applied during extrusion on the nutritional value of faba bean and degradation of phytic P isomers, Anim. Feed Sci. Technol., 2022, 285, 115221 CrossRef CAS.
- M. Qi, G. Zhang and Z. Ren,
et al., Impact of extrusion temperature on in vitro digestibility and pasting properties of pea flour, Plant Foods Hum. Nutr., 2021, 76, 26–30 CrossRef CAS PubMed.
- V. Orlien, K. Aalaei and M. M. Poojary,
et al., Effect of processing on in vitro digestibility (IVPD) of food proteins, Crit. Rev. Food Sci. Nutr., 2021, 63, 2790–2839 CrossRef PubMed.
- M. Cotacallapa-Sucapuca, E. N. Vega and H. A. Maieves,
et al., Extrusion process as an alternative to improve pulses products consumption. A review, Foods, 2021, 10, 1096 CrossRef CAS PubMed.
- D. Drulyte and V. Orlien, The effect of processing on digestion of legume proteins, Foods, 2019, 8, 224 CrossRef CAS PubMed.
- M. Opazo-Navarrete, D. Tagle Freire and R. M. Boom,
et al., The influence of starch and fibre on in vitro protein digestibility of dry fractionated quinoa seed (Riobamba Variety), Food Biophys., 2019, 14, 49–59 CrossRef.
- Q. Chen, J. Zhang and Y. Zhang,
et al., Rheological properties of pea protein isolate-amylose/amylopectin mixtures and the application in the high-moisture extruded meat substitutes, Food Hydrocolloids, 2021, 117, 106732 CrossRef CAS.
- M. Carbonaro, G. Grant and M. Cappelloni,
et al., Perspectives into factors limiting in vivo digestion of legume proteins: Antinutritional compounds or storage proteins?, J. Agric. Food Chem., 2000, 48, 742–749 CrossRef CAS PubMed.
- M. S. Joehnke, R. Lametsch and J. C. Sørensen, Improved in vitro digestibility of rapeseed napin proteins in mixtures with bovine beta-lactoglobulin, Food Res. Int., 2019, 123, 346–354 CrossRef CAS PubMed.
- J. H. Wong, T. Lau and N. Cai,
et al., Digestibility of protein and starch from sorghum (Sorghum bicolor) is linked to biochemical and structural features of grain endosperm, J. Cereal Sci., 2009, 49, 73–82 CrossRef CAS.
- E. Capuano and N. Pellegrini, An integrated look at the effect of structure on nutrient bioavailability in plant foods, J. Sci. Food Agric., 2019, 99, 493–498 CrossRef CAS PubMed.
- J. Lappi, P. Silventoinen-Veijalainen and S. Vanhatalo,
et al., The nutritional quality of animal-alternative processed foods based on plant or microbial proteins and the role of the food matrix, Trends Food Sci. Technol., 2022, 129, 144–154 CrossRef CAS.
- M. S. Joehnke, S. Sørensen and C. Bjergegaard,
et al., Effect of dietary fibre fractions on in vitro digestibility of rapeseed napin proteins, Pol. J. Food Nutr. Sci., 2018, 68, 335–345 CrossRef CAS.
- R. T. Boachie, M. M. B. Commandeur and R. O. Abioye,
et al., β-Glucan interaction with lentil (Lens culinaris) and yellow pea (Pisum sativum) proteins suppresses their in vitro digestibility, J. Agric. Food Chem., 2021, 69, 10630–10637 CrossRef CAS PubMed.
- L. Herreman, P. Nommensen, B. Pennings and M. C. Laus, Comprehensive overview of the quality of plant- and animal-sourced proteins based on the digestible indispensable amino acid score, Food Sci. Nutr., 2020, 8, 5379–5391 CrossRef CAS PubMed.
- S. Singh, S. Gamlath and L. Wakeling, Nutritional aspects of food extrusion: A review, Int. J. Food Sci. Technol., 2007, 42, 916–929 CrossRef CAS.
- Y. L. Xiong and A. Guo, Animal and plant protein oxidation: chemical and functional property significance, Foods, 2020, 10(1), 40 CrossRef PubMed.
- M. Hellwig, The chemistry of protein oxidation in food, Angew. Chem., Int. Ed., 2019, 58, 16742–16763 CrossRef CAS PubMed.
- C. L. Hawkins and M. J. Davies, Detection, identification, and quantification of oxidative protein modifications, J. Biol. Chem., 2019, 294, 19683–19708 CrossRef CAS PubMed.
- S. M. Rutherfurd and P. J. Moughan, Determination of sulfur amino acids in foods as related to bioavailability, J. AOAC Int., 2008, 91, 907–913 CrossRef CAS PubMed.
|
This journal is © The Royal Society of Chemistry 2023 |
Click here to see how this site uses Cookies. View our privacy policy here.