DOI:
10.1039/D3FO00835E
(Paper)
Food Funct., 2023,
14, 7387-7399
Wild blueberry (V. angustifolium) improves TNFα-induced cell barrier permeability through claudin-1 and oxidative stress modulation in Caco-2 cells
Received
28th February 2023
, Accepted 14th July 2023
First published on 24th July 2023
Abstract
Increasing evidence links the impairment of intestinal permeability (IP), a feature of the intestinal barrier, to numerous dysmetabolic and dysfunctional conditions. Several host and environmental factors, including dietary factors, can negatively and/or positively affect IP. In this regard, polyphenol-rich foods including berries have been proposed as potential IP modulators. However, the exact mechanisms involved are not yet fully elucidated. The aim of the present study was to evaluate the effect of a wild blueberry (WB; V. angustifolium) powder, naturally rich in polyphenols, to affect Caco-2 cell monolayer permeability and to identify the potential mechanisms in modulating the IP process. Caco-2 cells were incubated with TNF-α (10 ng mL−1), as a pro-inflammatory stimulus, and supplemented for 24 hours with different concentrations (1 and 5 mg mL−1) of WB powder. The integrity of the intestinal cell monolayer was evaluated by measuring the transepithelial electrical resistance (TEER) and the paracellular transport of FITC-dextran. In addition, the production of the tight junction proteins, such as claudin-1 and occludin, as well as protein carbonyl and 8-hydroxy 2 deoxyguanosine, as oxidative stress markers, were quantified in the supernatant by ELISA kits. Overall, the treatment with WB powder (5 mg mL−1) mitigated the loss of Caco-2 cell barrier integrity, as documented by an increase in TEER and a reduction in FITC values. This modulation was accompanied by an upregulation of claudin-1 and a reduction of 8-OHdG. Conversely, no effect was documented for the lower concentration (1 mg mL−1) and the other IP markers, as well as oxidative stress markers analysed. In conclusion, our findings suggest a potential role of WB in the modulation of cell barrier integrity. This modulation process could be attributed to an increase in claudin-1 expression and a reduction in 8-OHdG. Further studies should be performed to corroborate the results obtained. In addition, since the effects were observed at doses of WB achievable with the diet, these findings should be substantiated also through in vivo approaches.
1 Introduction
The intestinal barrier (IB) constitutes the interface between the outer and the inner milieu of the body. The interaction between physical and immunological barriers is essential for maintaining proper function and permeability of the barrier that allows the absorption and transport of nutrients and fluids, but also the protection from potentially harmful components from crossing the intestinal epithelium and reaching the systemic circulation.1 Thus, a well-organized monolayer of epithelial cells is required to form a selective permeability system mainly controlled by the transcellular (through specific transporters or membrane channels) and paracellular (transfer of substances passing through the intercellular space) pathways.2 The epithelial cells represent the main physical barrier whose integrity and control of the paracellular route are ensured by the presence of junction proteins between cells. These proteins include tight junctions (TJ), gap junctions (GJ), adherent junctions (AJ), and desmosomes. TJ proteins (i.e., claudins, occludin, and tricellulin) are located at the apical ends of the lateral membranes of the intestinal epithelium; they are able to interact with the adjacent cells and seal the paracellular space between epithelial cells.3 The communication between cells is mediated via intercellular channels that cluster in specialized regions of the plasma membrane to form GJ. Gap junction channels link the cytoplasm of two cells and provide a means for the exchange of small ions, metabolites and second messengers, allowing electrical and biochemical coupling between cells.4 Adherent junctions are located immediately below the TJ; they represent transmembrane proteins that strictly interact with the intracellular scaffold proteins, such as zonula occludens are able to anchor these transmembrane proteins to the actin cytoskeleton, and interact with occludin and claudins, providing a direct link with the cytoskeleton.3 The interaction of TJ proteins with the actin cytoskeleton is crucial for the maintenance of TJ structure and permits the cytoskeletal regulation of TJ barrier integrity.3 Finally, desmosomes represent intercellular junctions that provide strong adhesion between epithelial cells.
Disturbances of TJs have been associated with a loss of intestinal homeostasis and functional impairments of IB integrity known as “leaky gut”, characterized by an increased intestinal permeability (IP). When the IB is disrupted, the TJ are disordered, allowing for paracellular transport of several components such as food compounds, toxins, and parts of bacteria (such as bacterial lipopolysaccharides; LPS), resulting in their translocation from the luminal content to the inner layers of the intestinal wall leading to endotoxemia.5 In response to these stimuli, dendritic cells and macrophages are activated to produce pro-inflammatory cytokines which not only contribute to the TJ dysfunction but also enhance further infiltration of immune cells, activating circulating macrophages and resulting in increased systemic inflammation. This increase is thought to be important in the initiation and development of several intestinal and systemic diseases.6,7
There is evidence that dietary (poly)phenols (PPs) can contribute to reducing the impairment of IB and IP.8,9 Recently, we have summarized the potential mechanisms through which PPs and their metabolites contribute to the control of IP.10,11 In this regard, PPs may act at different levels of IB; first, by exerting a prebiotic effect by inhibiting the pathogenic bacteria and stimulating the growth of beneficial microbes. This modification in the gut microbiota composition, may reduce the production of endotoxins and stimulate the growth of short-chain fatty acid-producing bacteria able to prevent the translocation of pathogens and antigens. Second, PPs may act at the intracellular level through an up- and/or down-regulation of TJ, AJ, GJ, and desmosome protein expression, thus contributing to the maintenance of enterocyte stability, communication, and functionality. In addition, PPs can regulate transcription factors like nuclear factor erythroid 2-related factor 2 (Nrf2), nuclear factor kappa B (NF-κB) and toll-like receptor 4 (TLR4), contributing to the control of oxidative stress and inflammatory response. Third, PPs may maintain a functional immune system and control inflammation at the systemic level.10,11
Blueberries represent one of the worldwide popular berries. Production, trade, and consumption of blueberries are expanding globally ranging from pureed to powdered forms, due to their flavor, versatility as ingredients and additives in foods and beverages, but also for their potential health benefits due to their nutrient and non-nutrient content.12–14 In this regard, wild blueberries (WB) contain large amounts of PPs, in particular anthocyanins and chlorogenic acids. Numerous in vitro and in vivo studies have reported the capacity of WB to reduce intestinal and vascular inflammation,15–17 lipid accumulation,18,19 oxidative stress,20,21 affect cell migration and angiogenesis,22,23 improve vasoreactivity,24–26 glucose metabolism,27 and gut microbiota composition.28,29 To the best of our knowledge, the capacity of WB to affect the IB and IP has never been tested. Thus, in the present study, we examined for the first time the effect of a WB powder on barrier function in Caco-2 intestinal epithelial cells aiming to identify the potential mechanisms involved in the modulation of IP.
2 Materials and methods
2.1 Chemicals, cells and reagents
Human Caucasian colon adenocarcinoma (Caco-2) cells (cat. no. 09042001-1VL), tested for intestinal permeability characteristics, were from the European Collection of Authenticated Cell Cultures (ECACC) and purchased by Sigma-Aldrich (St Louis, MO, USA). Minimum essential medium (MEM; cat. no 51411C-1000mL), penicillin–streptomycin solution (cat. no. P4333-100mL), MEM non-essential amino acid solution (100×) (cat. no. M7145-100mML), trypsin–EDTA (cat no. T4049-100ML) were provided by Sigma-Aldrich (St Louis, MO, USA). MEM phenol red free (cat no. 51200-038), sodium pyruvate (cat no. 11360-070), and fetal bovine serum (FBS; cat no. A47668-01) were provided by Thermo Fisher Scientific (Waltham, MA USA). Phosphate saline buffer (PBS; cat no. 806544-500ML), interferon-γ human (INF-γ; cat no. I17001-100UG), Trypan blue (cat no. T8154-100ML), potassium chloride, sodium chloride, fluorescein-5-isothiocyanate (FITC; cat no. 46944-100MG), and TRITON x100 were provided by Sigma-Aldrich (St Louis, MO, USA). TNF-α (cat no. rcyc-htnfa) was provided by InvivoGen (San Diego, CA, USA). Millicell® tissue culture plate well inserts (cat. no. PIHP01250), ethylenedinitrilotetraacetic acid disodium salt dihydrate (EDTA Na; cat no. E5134-500G) 3-(4,5-dimethylthiazol-2-yl)-2,5-diphenyltetrazolium bromide (MTT; cat no. M2128-500MG), dimethyl sulfoxide (DMSO), sodium dodecyl sulfate (SDS; cat no. 822050) and ethanol were purchased from Merck (Darmstadt, Germany). ProteinSafeTM protease inhibitor cocktail (100×) (cat no. DI111-02) was provided by Clinisciences (Guidonia Montecelio, RM, Italy). Tris-(hydroxymethyl)-aminomethan (tris; cat no. 33742) was provided by Honeywell International Inc. (Monza, MB, Italy). Standards of cyanidin (Cy)-, delphinidin (D)-, petunidin (Pt)-, peonidin (Pe)-, malvidin (Mv)- and their 3-O-glucoside (glc), Cy-, Pet-, Peo-, Mv-3-O-galactoside (gal) and Cy-arabinoside (Cy-ara) were purchased from Polyphenols Laboratory (Sandnes, Norway). Chlorogenic acid was from Sigma-Aldrich (Milan, Italy). Methanol, acetonitrile, and phosphoric acid were from Merck (Darmstadt, Germany). Water was from a Milli-Q apparatus (Millipore, Milford, MA).
2.2 Preparation of the WB stock solution
WB (V. angustifolium) freeze-dried powder was provided by FutureCeuticals (Momence, Ill., USA) and stored at −20 °C until use. The powder (100 mg) was dissolved in MEM (1 mL), vortexed for 30 seconds, and stored at 4 °C overnight to optimize solubilization. The solution was centrifuged at 865 rcf (3000 rpm) for 10 minutes and twice at 16.249 rcf (13
000 rpm) for 1 minute (rotor cat no. RA24-2 86 mm). Successively, the powder was filtered with sterilized filters (dimension 0.2 μm) to provide a stock solution at 100 mg mL−1. Finally, the stock solution was diluted until the final concentration of 5 and 1 mg mL−1.
Both tested concentrations represent an amount easily achievable through the diet. In fact, by considering an average colon volume of 561 mL,30 the concentration of 5 mg mL−1 of powder corresponds to about 28 g of fresh blueberries (the powder humidity is <5%). In terms of phenolic content (mainly represented by anthocyanins, PAC and chlorogenic acid), the quantity tested is approximately 125 μg mL−1 (∼2.5% of the powder), which is clearly reasonable since the model of Caco-2 provides for the direct contact of the compounds in the intestinal lumen with intestinal barrier cells. Also, considering the low bioavailability of blueberry (poly)phenols (0.1–1%),31 the absorbed quantity would be in the range of 125 ng mL−1 to 1.25 μg mL−1, considering physiological serum levels of these phenolic compounds following blueberry intake.32
2.3 Quantification of anthocyanins and chlorogenic acids
Approximately 50 mg of powder was dissolved in 5 mL of a solution methanol
:
1% H3PO4 in water (10
:
90, v/v) and the suspension was sonicated for 5 min, centrifuged at 1000g for 5 min and the supernatant was recovered. The residue was extracted with 4 mL of a solution of methanol
:
1% H3PO4 in water (10
:
90, v/v) and treated as described above. The supernatants were combined and then the final volume was adjusted to 10 mL with a solution of 1% H3PO4 in water. For the analysis, the WB stock solution was diluted by a solution of 1% H3PO4 in water.
Individual anthocyanins and chlorogenic acids were analysed by HPLC-DAD method. The HPLC system was an Alliance mod. 2695 (Waters, Milford, MA) equipped with a mod. 2998 photodiode array detector (Waters). The separation was carried out by a C18 Kinetex column (150 × 4.6 mm, 2.6 μm, Phenomenex, Torrence, CA) maintained at 45 °C. The flow rate was 1.7 mL min−1 and the eluents were (A) 1% H3PO4 and (B) acetonitrile/1% H3PO4 in water (35
:
65, v/v). The elution gradient was linear as indicated as follows: 0–15 min 14% B; 15–25 min from 14 to 20% B; 25–35 min from 20 to 32% B; 35–45 min from 32 to 50% B; 45–48 min from 50 to 90% B; 90% for 3 minutes. Chromatographic data were acquired from 200 to 700 nm and integrated at 520 nm. Anthocyanin and chlorogenic acid calibration curve was in the range 2–50 μg mL−1. Each analysis was carried out in duplicate.
2.4 Determination of the total amount of (poly)phenols and proanthocyanidins
To determine the total (poly)phenols in the WB powder and stock solution, the Folin–Ciocalteau method was used with gallic acid as a reference standard (Singleton, 1965). The results from three independent analyses are expressed as grams per 100 grams of gallic acid equivalents (GAE). For the determination of total proanthocyanidins, the method described by Gardana et al. was followed.33
2.5 Quantification of total sugars, proteins, lipids and fiber
The quantification of sugars (glucose and fructose) was carried out by UPLC (Acquity, Waters) coupled with a triple-quadrupole mass spectrometer model Quattro micro (Micromass), as previously reported.15 The conventional acid hydrolysis and Kjeldahl digestion methods were employed to determine the nitrogen (N) content in 2 g of WB, with the assistance of a copper catalyst. The resulting ammonia was collected by distillation and mixed with a boric acid solution, which was subsequently titrated with standard acid. The Kjeltec 1002 apparatus (Foss, Milan, IT) was utilized for the digestion and distillation processes. The protein content was then calculated as the product of the total nitrogen and 5.6. For the analysis of total lipid, the extraction method used for WB involved Soxhlet extraction, where 6 g of WB powder and 10 g of Na2SO4 were combined in a Soxtec HT 1043 system (Foss, Milan, Italy). The system contained 180 mL of a solution consisting of ethyl ether and petroleum ether in a 1
:
1 ratio (v/v). The mixture underwent extraction at a temperature of 140 °C for a duration of 12 hours. Subsequently, a solvent rinse of 30 minutes was performed, followed by evaporation of the solvent. The remaining residue was weighed and considered as the lipid content (% DW). The WB was further characterized for the content of fiber.34
2.6 Caco-2 cell culture
Minimum essential medium (MEM) supplemented with 10% (v/v) fetal bovine serum, antibiotics (50 U mL−1 penicillin, 50 μg mL−1 streptomycin), 1% (v/v) of 100× non-essential amino acids, and 1 mM sodium pyruvate was used to culture Caco-2 cells. An atmosphere of 37 °C and 5% (v/v) CO2 was utilized to maintain the cell culture. Cells were subcultured at 80% confluence with 0.05% trypsin/EDTA. The medium was replaced every 2–3 days during cell growth and differentiation. Cells were used between passages 3 and 15.
2.7 Cell viability assay
Trypan blue exclusion assay using a TC20TM automated cell counter and dual-chamber cell counting slides (BIORAD, Segrate, Milan, Italy) was employed to test the toxicity of the compounds on Caco-2 cell culture. Caco-2 cells were seeded in 12-well plates at a density of 400
000 cells. Cells with 80% confluence grade were treated with C (negative control; cell culture medium), TX-100 (positive control; 0.1% Triton), and WB at different concentrations (1–10 mg mL−1) for 24 hours. Subsequently, cells were trypsinized, resuspended, and used for Trypan blue exclusion assay. Three independent experiments were performed in which each condition was tested in triplicate.
Furthermore, the toxicity of the compounds was also tested by MTT assay.35 Caco-2 cells were seeded in 96-well plates at a density of 5000 cells per well and cultured for 24 hours before the addition of the extracts at different concentrations. Cell viability was tested 24 hours after adding the C, TX-100, and extracts at different concentrations (1–10 mg mL−1) using an MTT assay. The MTT solution was made in the cell culture complete medium (dissolving 1 mg mL−1 of MTT reagent) and filtered, sterilized, and added to each well. The plate was incubated at 37 °C for 4 hours in the dark. MTT solution was removed, 200 μl DMSO was added to solubilize formazan crystals, and the plate was incubated in the dark on a shaker for 20 minutes at room temperature. Absorbance in each well was measured at 450 nm using a plate reader (mod. F200 Infinite, TECAN, Milan, Italy). Three independent experiments were performed in which each condition was tested in six replicates.
2.8 Assessment of intestinal permeability by TEER and FITC assay
The assessment of the intestinal permeability was performed through the evaluation of the transepithelial electrical resistance (TEER) and the transport of fluorescein isothiocyanate-dextran (FITC-dextran).36,37 Caco-2 cells with 80% confluence grade were seeded on Transwell® 24-well permeable media (12 mm, 0.4 μm pore polyester membranes) at a density of approximately 20
000 cells per well. Blank wells without cells were also included in the plate. Cells were differentiated into polarized monolayers by growing on Transwell inserts for 18–21 days. The volume of medium added to the upper and lower compartments was 0.4 and 0.6 mL, respectively. After differentiation, Caco-2 cells were pre-incubated with interferon-γ (INF-γ; 10 ng mL−1) for 24 hours added to the lower chamber, to promote the exposure of tumor necrosis factor-α (TNF-α) receptors outside the cell membrane.37 Then, cells were treated with TNF-α (10 ng mL−1) and with different concentrations of WB powder (5 mg mL−1 and 1 mg mL−1) for 24 hours. TNF-α was added to the lower chamber, while the WB powder to the upper chamber. The negative control was represented by the untreated cells, while the positive control by the cells treated only with TNF-α. The experiments were performed in a serum- and phenol red-free medium. INF-γ, TNF-α, and the WB powder were previously diluted in the medium.
The analysis of TEER was used to measure the integrity of the TJ of the Caco-2 monolayer. TEER was measured using a Millicell-ERS resistance system (Millipore, Bedford, MA, USA) that includes a dual-electrode volt-ohm-meter. TEER was calculated as follows: TEER = (Rm − Ri) × A, where Rm is transmembrane resistance, Ri the intrinsic resistance of cell-free media, and A the surface area of the membrane in cm2. Monolayers were used when TEER values were between 350 and 450 Ω cm2. TEER was estimated before the treatment with IFN-γ/TNF-α and after the treatment with the inflammatory stimuli and the extracts. Before the TEER evaluation, the incubation medium was replaced with fresh medium. Three independent experiments were performed in which each condition was tested in triplicate.
The paracellular transport of FITC-D, a nonpolar fluorescent molecule spectrophotometrically detectable, was measured to evaluate Caco-2 cell monolayer permeability. After the TEER assessment, the medium was replaced with fresh medium. FITC-dextran prepared in medium (1 mg mL−1) was added to the upper chamber. The blank well without cells was used as a positive test control. The plate was incubated for 4 hours, and then, 50/100 μl of the medium in the lower chamber was collected in a 96-well plate. FITC-dextran was passed through the monolayer and was quantified by a fluorescence plate reader (mod. F200 Infinite, TECAN, Milan, Italy), with the excitation wavelength at 485–488 nm and the emission wavelength at 520–530 nm. Three independent experiments were performed in which each condition was tested in triplicate.
2.9 Assessment of intestinal permeability and oxidative stress markers by enzyme-linked immunosorbent assay (ELISA)
Caco-2 cells were seeded in a 6-well plate (300
000 cells per well) in duplicate to collect a sufficient number of cells for the analysis. Cells were differentiated into polarized monolayers for 8–10 days and followed the same treatment phase for the TEER evaluation described above (see paragraph 2.6). The assessment of protein carbonyl (PC), and 8-hydroxy-2-deoxyguanosine (8OHdG) was performed on the cell culture supernatant. While cell extract was used for the detection of claudin-1 (CLN-1), occludin (OCLN) and zonula occludens-1 (ZO-1). After the treatment, the cell supernatant was collected and centrifuged at 216 rcf (1500 rpm) for 10 min at 4 °C. Protein extraction was done, in accordance with the manufacturer's protocol. Briefly, cells were placed on ice, washed with cold PBS, and incubated for 1 minute on ice with a lysis buffer (100 mM tris, 150 mM NaCl, 1 mM EDTA Na, 1% SDS, 1% Triton, 1% proteases inhibitor). After detaching, the cells were incubated in ice for 30 min and vortexed every 10 min. The cell lysate was centrifuged at 16
249 rcf (13
000 rpm) for 10 min at 4 °C. Aliquots of supernatants and cell lysate were stored at −80 °C until analysis.
An ELISA kit was used to quantify the protein levels of claudin-1, occludin, ZO-1, PC, 8-OHdG (cat no. were MBS3800570, MBS451733, MBS2605490, MBS2602535, MBS267161 respectively, MyBioSource, Inc. San Diego, CA, USA), and IL-6, TNF-α (cat no. were EK0410, EK0525 respectively, booster biological technology, Pleasanton, CA, USA). Analyses were conducted in triplicate, and the results were derived from three independent experiments.
2.10 Statistical analysis
Statistical analysis was performed by means of GraphPad software (GraphPad Software Boston, MA, USA). The effects of the WB powder concentrations on Caco-2 cell monolayer permeability, TJ proteins and markers of oxidative stress were assessed by one-way analysis of variance (ANOVA). Post hoc analysis of differences between treatments was assessed by the least significant difference (LSD) test by setting the level of statistical significance for p ≤ 0.05. Data were derived from three independent experiments in which each condition was tested in triplicate. Results are reported as mean ± standard error of the mean.
3 Results
3.1 Chemical characterization of WB
Anthocyanins and chlorogenic acid in the WB powder and stock solution were identified by co-chromatography, on-line UV-Vis spectra, molecular weight and all-ions fragment. The chromatogram integrated at 520 nm documented the presence of 15 anthocyanins and the absence of the respective aglycones and acylated forms (Fig. 1A), while the integration at 325 nm (Fig. 1B) instead showed the presence of a main peak, identified as chlorogenic acid. The individual anthocyanin and chlorogenic acid percentage in WB powder was reported in Table 1. Monomeric ACNs and chlorogenic acid in the WB was 1.53 ± 0.04 and 0.57 ± 0.01%, respectively. The nutritional composition of the WB is reported in Table 2.
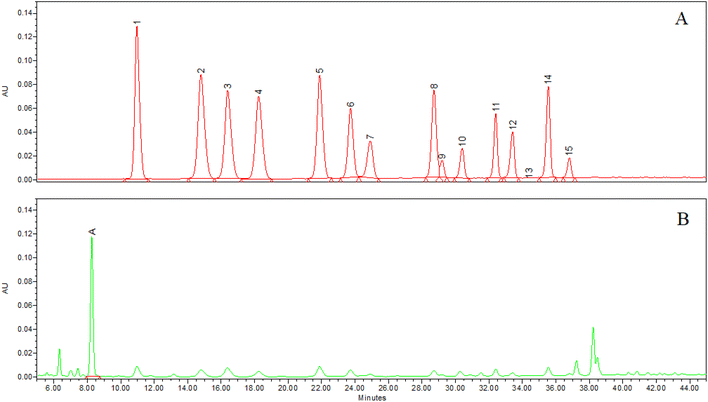 |
| Fig. 1 Chromatogram integrated at 520 nm (A) and 325 nm (B) of wild blueberry extract (see Table 1 for peak identification and amount). | |
Table 1 Compounds identified in the analysed samples. For each compound the retention time (RT min), molecular and fragment ions formula and the percentage in the stock solution is reported
Peak |
RT (min) |
Molecular formula |
Fragment ion formula |
Peak identification |
% |
Chlorogenic acid: CHL, D: delphinidin, Cy: cyanidin, Pet: petunidin, Peo: peonidin, Mv: malvidin, gal: galactose, glc: glucose, ara: arabinose. |
A |
8.3 |
C16H18O9 |
C9H8O4, C7H12O6 |
CHL |
0.57 |
1 |
10.0 |
C21H21O12 |
C15H12O6 |
D-Gal |
0.17 |
2 |
14.0 |
C21H21O12 |
C15H11O7 |
D-Glc |
0.20 |
3 |
15.0 |
C21H21O11 |
C15H11O6 |
Cy-gal |
0.08 |
4 |
17.0 |
C20H19O11 |
C15H11O7 |
D-ara |
0.11 |
5 |
22.0 |
C21H21O11 |
C15H11O6 |
Cy-glc |
0.09 |
6 |
24.0 |
C20H19O10 |
C15H11O6 |
Cy-ara |
0.05 |
7 |
25.0 |
C22H23O12 |
C16H13O7 |
Pet-gal |
0.09 |
8 |
28.5 |
C21H21O12 |
C16H13O7 |
Pet-glc |
0.12 |
9 |
29.0 |
C22H23O11 |
C13H13O6 |
Peo-gal |
0.02 |
10 |
30.2 |
C21H21O11 |
C16H13O7 |
Pet-ara |
0.06 |
11 |
32.0 |
C22H23O11 |
C13H13O6 |
Peo-glc |
0.06 |
12 |
32.5 |
C23H25O12 |
C17H15O7 |
Mv-gal |
0.18 |
13 |
33.2 |
C21H21O10 |
C13H13O6 |
Peo-ara |
0.02 |
14 |
34.5 |
C23H25O12 |
C17H15O7 |
Mv-glc |
0.21 |
15 |
35.4 |
C22H23O11 |
C17H15O7 |
Mv-ara |
0.11 |
Table 2 Composition of the wild blueberry powder and stock solution
Nutritional composition |
Powder (mg per 100 mg) |
Stock solution (mg per mL) |
Not detectable: ND. |
Total fat |
5.60 |
ND |
Protein |
2.80 |
1.43 |
Sugars |
68.4 |
60.3 |
Fiber |
16.8 |
1.91 |
Soluble |
2.40 |
1.91 |
Insoluble |
14.4 |
ND |
Total polyphenols |
2.46 |
2.46 |
Anthocyanins |
1.53 |
1.53 |
Proanthocyanins |
0.55 |
0.55 |
Chlorogenic acid |
0.57 |
0.57 |
3.2 Effect of WB on cell viability
The impact of the WB powder tested at different concentrations on cell toxicity were assessed by the Trypan blue exclusion assay and the MTT assay. The findings obtained were consistent with each other. Notably, the positive control (Triton X-100; TX-100) exhibited a reduction below 10% in cell viability values. Also, the highest concentration of WB (WB10; 10 mg mL−1) demonstrated a cytotoxic effect, reducing cell viability by around 50% compared to the negative control. Conversely, the treatment where only TNF-α (10 ng mL−1), WB5 (5 mg mL−1) and WB1 (1 mg mL−1) was used, maintained cell viability values above 90%.
3.3 Effect of WB on TNFα-induced permeabilization
In Fig. 2(A and B), results related to the effect of WB on IP are reported. IP was detected by the assessment of TEER (Fig. 2A) and FITC (Fig. 2B). The inflammatory stimulus induced by TNF-α (10 ng mL−1) documented a statistically significant reduction of TEER (−40%; p < 0.05) compared to the negative control. The administration of WB powder at the concentration of 5 mg mL−1, but not 1 mg mL−1, was capable of counteracting the loss of the TEER values following the inflammatory stimulus, maintaining the TEER values comparable to the negative control (Fig. 2A). The transport of FITC across the Caco-2 cell monolayer was significantly increased by TNF-α, two and a half times higher compared to the condition without the inflammatory stimulus (Fig. 2B). The treatment with 5 mg mL−1 of WB was able to inhibit the rise in paracellular transport (p < 0.05) in the presence of TNF-α, with analogue values to the negative control.
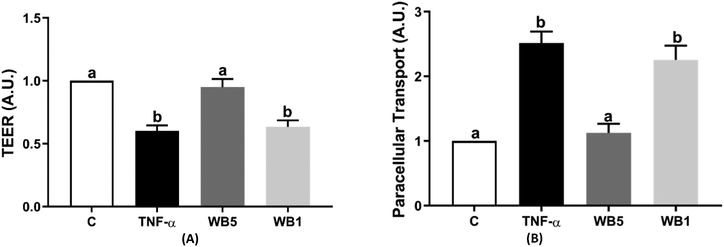 |
| Fig. 2 Effect of the wild blueberry (WB) powder on transepithelial electrical resistance (TEER) (A) and transport of fluorescein isothiocyanate-dextran (FITC-dextran) (B). Each data derives from three independent experiments in which each condition was tested in triplicate. C: negative control (no TNF-α); TNF-α: tumor necrosis factor-alpha (positive control, 10 ng mL−1); WB: wild blueberry (TNF-α + WB) tested at 1 (WB1) and 5 mg mL−1 (WB5). Data are reported as mean ± standard deviation. a,b Bars with different letters are significantly different (p < 0.05). | |
Again, the concentration of WB tested at 1 mg mL−1 was ineffective, showing a significant increase with values comparable to those of the positive control.
3.4 Effect of WB on tight junction proteins
Fig. 3(A–C) depicts the levels of claudin-1 (A), occludin (B) and ZO-1 (C) measured following the different treatments. As reported in Fig. 3A, the inflammatory stimulus documented a 19.6% decrease in claudin-1 protein expression compared to the negative control (p < 0.05). The incubation with 1 mg mL−1 of WB extract (WB1) did not counteract the impairment induced by TNF-α, showing a significant reduction (−17.1%; p < 0.05) in claudin-1 compared to the control. On the contrary, the highest concentration of WB (5 mg mL−1) was able to counteract the effects of TNF-α and maintain the levels of claudin-1 comparable to the control. Regarding occludin (Fig. 3B) and ZO-1 (Fig. 3C), both TNF-α and WB were ineffective to modulate the protein levels.
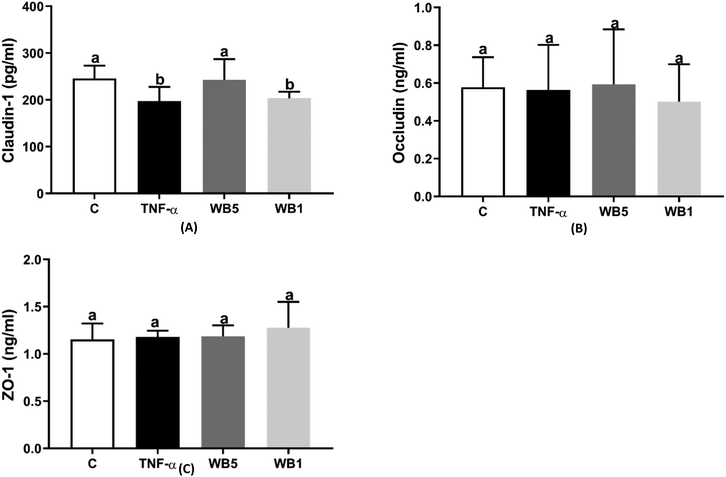 |
| Fig. 3 Effect of wild blueberry (WB) powder on claudin-1 (A), occludin (B) and zonula occludens (C). Each data derives from three independent experiments in which each condition was tested in triplicate. ZO-1: zonula occludens; C: negative control (no TNF-α); TNF-α: tumor necrosis factor-alpha (positive control, 10 ng mL−1); WB: wild blueberry (TNF-α + WB) tested at 1 (WB1) and 5 mg mL−1 (WB5). Data are reported as mean ± standard deviation. a,b Bars with different letters are significantly different (p < 0.05). | |
3.5 Effect of WB on oxidative stress markers
The findings related to the levels of 8-OHdG and protein carbonyl, as markers of oxidative stress, are reported in Fig. 4A and B, respectively. Although a pro-inflammatory stimulus was used to induce an oxidative stress condition, no significant change was observed in both biomarkers following the administration of TNF-α. However, the treatment with the WB powder at the concentration of 5 mg mL−1 revealed a significant reduction in the levels of 8-OHdG (−46.9%; p < 0.05, Fig. 4A) with respect to the control. No effect was documented when testing WB at the concentration of 1 mg mL−1. Regarding protein carbonyls (Fig. 4B), neither the stimulation with TNF-α nor the administration of the WB powder was able to affect their concentrations.
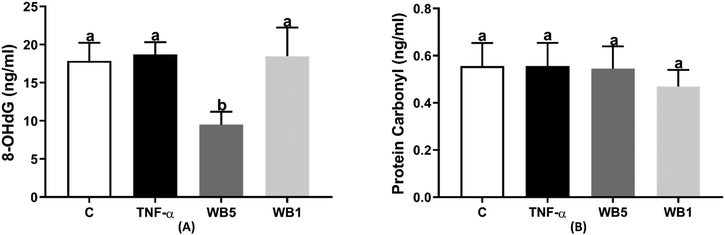 |
| Fig. 4 Effect of wild blueberry (WB) powder on 8-hydroxy-2-deoxyguanosine (A) and protein carbonyl (B). Each data derives from three independent experiments in which each condition was tested in triplicate. C: negative control (no TNF-α); TNF-α: tumor necrosis factor alpha (positive control, 10 ng mL−1); WB: wild blueberry (TNF-α + WB) tested at 1 (WB1) and 5 mg mL−1 (WB5). Data are reported as mean ± standard deviation. a,b Bars with different letters are significantly different (P < 0.05). | |
4 Discussion
In the current work, we observed the capacity of WB powder to counteract an impairment in the barrier dysfunction induced by an inflammatory stimulus as documented by the analysis on TEER and FITC. The underlying mechanisms of action involved seem to be an increase in claudin-1, as a TJ protein, and a reduction of the levels of 8-OHdG as a marker of oxidative stress.
Regarding TEER and FITC, we observed that the WB powder had a beneficial role on the integrity of the Caco-2 cell monolayer by increasing TEER and reducing FITC. This improvement was documented only when testing the highest WB concentration (5 mg mL−1), while no effect was demonstrated when using the lowest concentration (1 mg mL−1). However, it is important to underline that the highest WB concentration represents an amount easily achievable through the diet. In fact, this dose is equivalent to a small single portion of fresh blueberries (25 g), which could correspond to the minimum effective intake able to exert a positive role on IB integrity. Our findings are in accordance with those present in the literature documenting the capacity of blueberry (poly)phenols to maintain the integrity of the IB,37–42 measured by using the same assays, in the same Caco-2 cell model which is a widely recognized in vitro model of the intestinal epithelium. For instance, Polewski and colleagues38 found a recovery of TEER reduction by (poly)phenolic fractions from a freeze-dried blueberry powder after stimulation with E. coli. In particular, the most effective fraction was represented by the anthocyanins tested at 10, 25, 50, or 100 μg of GAE per mL for 24 hours. Also, Cremonini and coworkers37 tested the effect of anthocyanins from blueberries on the IB integrity showing that the use of an anthocyanin-rich extract and the individual compounds (in the range 0.25–1 μM) prevented the loss of TEER values following stimulation with TNF-α (5 ng mL−1 for 24 hours) in the same cell model. Other studies have investigated the effect of PPs and/or PP-rich foods on IB and permeability.39–42 The majority of the evidence seems to support the contribution of PPs in the modulation of TEER and FITC. In particular, the main positive evidence seems to derive from (−)-epicatechin,39 kaempferol,40 and naringenin.41,42
In the context of IB, TJ proteins play a key role in the modulation of IP. In our experimental conditions, the supplementation with the WB powder showed an increase in claudin-1, while no effect was observed in the levels of ZO-1 and occludin after stimulation with TNF-α, which in its turn was not able to affect the expression of these proteins. These results are partially in contrast to the ones obtained in other studies by using similar PPs. For example, Remenyik et al.43 investigated the effect of an anthocyanin-rich extract from sour cherry on TJ proteins in Caco-2 exposed to an inflammatory stimulus (50 ng mL−1 of TNF-α for 24 hours). The authors found that the extract (85 μM) counteracted the lowering of ZO-1 protein levels and up-regulated the levels of occludin. On the other side, the authors showed that occludin levels were not modulated by TNF-α, in line with our observations. Taladrid and coworkers44 found that a PP-rich extract deriving from intestinal digestion of grape pomace was ineffective in modulating mRNA levels of ZO-1 and occludin. Nevertheless, the same extract exerted a reduction of FITC paracellular transport through Caco-2 cell monolayer, indicating how the modulation of IP is determined by complex interactions and several proteins play a decisive role. These diverse results between studies may be attributed to the different concentrations used in terms of extract (5 mg mL−1vs. 12.5 mg mL−1) and TNF-α (10 vs. 50 ng mL−1). Another important difference could be related to the different food sources from which anthocyanins were obtained (blueberry, sour cherry, grape pomace). Studies investigating the effects of wild blueberry have reported mixed results that may be attributed to the differences in terms of anthocyanin composition among berries. For instance, Wu et al. observed that an 8-week treatment with blueberry (V. ashei) was able to reduce body weight in high-fat diet-induced obese male C57BL/6 mice.45 Differently, in the same animal model, an 8-week treatment by using a mix of blueberry varieties (V. ashei and V. corymbosum) failed to counteract an increase of body weight.46 Thus, the results may not be generalizable to all wild blueberry varieties but must be contextualized to the type of blueberry tested. In addition, a further explanation may be due to the distinct mechanisms of action of the bioactives characterizing the extracts. For instance, the wild blueberry powder tested in the current study is particularly rich in chlorogenic acids and some anthocyanins such as D-glc, D-gal, Mv-glc, and Mv-gal, which most likely have a synergistic role than acting as individual compounds. In this regard, Valdez and colleagues47 showed the efficacy of an Aronia berry extract (5 mg mL−1) to counteract the IB dysfunction in an in vitro model of Caco-2 cells, while no protective effect has been exerted by individual PPs or phenolic catabolites. In the same study,47 but in contrast with our findings, an increase in claudin-1 mRNA expression was observed following treatment with an inflammatory cocktail (50 ng mL−1 TNF-α, 50 ng mL−1 IFN-γ, 25 ng mL−1 IL-1β, 1 mg mL−1 LPS), which was attenuated by the administration of Aronia berry extract. However, due to the recognized role of claudin-1 in sealing the intercellular space at the level of enterocytes, it seems that the inflammatory stimulus resulted in an improvement in intestinal permeability. Nevertheless, it is important to highlight that the authors assessed claudin-1 at mRNA levels, which are not necessarily translated into proteins. In the study of Kim and colleagues,48 Aronia berry extract (62.5–250 μg mL−1) increased the level of several types of TJ protein such as ZO-1, claudin-3, claudin-4 and JAM-1 after an inflammatory stimulation mediated by LPS on Caco-2 cell line, while claudin-1 and occludin protein levels remained unchanged. Furthermore, the authors observed an improvement in the loss of TEER due to the LPS treatment. In another study, Singh et al.49 tested the effect of 50 μM of urolithin-A, one of the main polyphenol microbial metabolites on FITC paracellular transport in Caco-2 cells and in HT29 cell lines. Results showed a reduction of FITC in line with our findings. Nevertheless, urolithin-A increased the mRNA expression and the protein level of zo-1, occludin and claudin-4 in both Caco-2 and HT29. Moreover, urolithin-A increased the expression of heme oxygenase-1, an Nrf2-dependent gene with a crucial role in the protection against oxidative stress and inflammation. Conversely, Lian and colleagues50 evaluated the effect of resveratrol, on a co-culture model of Caco-2 and HT29 in a condition of hypoxia and heat-induced epithelial injury. The authors found that the exposure to heat and hypoxia degraded the barrier integrity, as observed by the reduction of TEER values and the expression of zo-1 and claudin-3 proteins. The treatment with resveratrol (25–100 μM) counteracted the loss of TEER values but had no effect on TJ proteins level. However, resveratrol (50 and 100 μM) mitigated the MDA production, a biomarker of lipid peroxidation, enhanced by heat and hypoxia exposure. Also, Cremonini et al. were able to document the ability of anthocyanins (0.1–5 μM) to mitigate oxidative stress induced by TNF-α (5 ng mL−1 for 6 hours) in Caco-2 cells monolayer, which also experienced a recovery in TEER and FITC values.51 In accordance with our findings, evidence deriving from an in vivo study demonstrated that in a knockout and transgenic model of mice (C57BL/6J) fed with a high-fat diet, the administration of a physiologically-relevant dose of 40 mg anthocyanin per kg body weight provided for 14 weeks was capable to counteract the reduction of claudin-1, exhibiting a protective role on the IB.51 In another study, no effect on the expression levels of ZO-1 and occludin was documented in mice fed with a high-fat high-sucrose (HFHS) diet and wild blueberry (Vaccinium angustifolium Aiton) (200 mg kg−1, providing 17 mg of polyphenols per day), in line with our findings. However, the same authors examined the effects of wild blueberry polyphenols on the histomorphology of mouse colon mucosa by measuring the length of crypts and the thickness of the mucus layer. Interestingly, the authors found that HFHS-fed mice had a thinner mucus layer compared to chow-fed mice, but all mice supplemented with wild blueberry showed a significantly thicker mucus layer compared to the HFHS control group treated with vehicle. While the depth of the crypts did not differ among the groups.52 Yan and colleagues (2019) analysed the effect of the administration of 10 mL kg−1 of blueberry juice in male Sprague–Dawley rats exposed to CCl4 experienced disruptions in the integrity of the intestinal epithelial barrier and downregulation of TJ proteins. Overall, the authors found that the treatment with blueberry resulted in an improved colon structure and increased protein and mRNA levels of claudin1, claudin2, and ZO1, which were reduced after CCl4 exposure.53 Cheng and colleagues assessed the mRNA expression levels of structural tight junction (TJ) proteins in mice fed with control or high fat diets with and without different concentrations of fermented blueberry pomace (4, 6 and 8%). Overall, barrier-associated functional proteins (ZO-1, claudin-1, claudin-4, occludin and MUC2) exhibited a significant decrease in mRNA expression following the high-fat diet (HFD) compared to the control diet. However, when mice were supplemented with fermented blueberry pomace (FBP), there was a notable increase in the expression of barrier-associated genes, regardless of whether the mice were on a control or high-fat diet. Although still limited, findings from animal models suggest that blueberries may have the potential to protect the colonic barrier function.54 These studies have examined the modulation of TJ proteins in the context of gut permeability, their results, including those of the current study, are highly heterogeneous. However, this is due to differences in the methodology of the experiments, in the variety of food, extract or polyphenol compound, in the concentrations and treatment time, in the inflammatory stimulus type and cell culture model. Understanding what mechanisms of action are behind the effect of these bioactive compounds is very complex, so further studies are needed to evaluate different markers related to intestinal permeability. Although our study did not directly measure pro-inflammatory cytokine levels, we cannot exclude that one possible mechanism could be the reduction of the inflammatory stimulation. In fact, the association between the consumption of blueberries and the positive impact on human health can be attributed, at least in part, to the modulation of molecular pathways involved in inflammation.55 Further, when exposed to TNF-α stimulation, polarized intestinal epithelial cells exhibit a targeted and directional release of the proinflammatory cytokine IL-8. These results are particularly
noteworthy as they indicate that intestinal epithelial cells possess the ability to coordinate their response to inflammatory signals and generate inflammatory mediators in a focused and directional manner.56 Thus, the reduction in pro-inflammatory cytokines may also have an impact on the intestinal barrier function through the disruption of the integrity of the intestinal barrier, leading to increased permeability and potential translocation of harmful substances.
Also, it is necessary to consider that the integrity of the intestinal barrier can be affected by the inflammatory and oxidative status at the level of the epithelium. Inflammatory processes induce oxidative stress characterized by a rise in the production of reactive oxygen species (ROS). An excess of ROS reduces cellular antioxidant capacity determining an increase in proteins, lipids, and DNA damage. One of the main products of protein oxidation are protein carbonyls (PC) whose formation typically responds to the oxidative deamination of alkaline amino acids such as lysine, arginine, and prolines.57 As protein carbonylation is an irreversible process and the cellular enzymes are unable to repair such oxidative damage, one of the main biological consequences of this process is the loss of protein structure. These modifications may be the cause of alterations (e.g., in the communications, signalling, and functions) at the level of numerous cells including those at the epithelial level with negative consequences on intestinal barrier and permeability.58 The role of berries and berry-polyphenols on PC production has been poorly investigated and the available results failed to document a positive effect both in vitro and in vivo. Our observations seem in line with these findings; in fact, in our experimental conditions, neither the use of TNF-α as an inflammatory stimulus nor the supplementation with WB was able to affect PC levels. Regarding DNA damage, recent studies have also reported that an increase at the epithelial level may compromise intestinal barrier function due to alterations in TJ functionality.59 8-OHdG is one of the most sensitive biomarkers of oxidative stress and therefore, it is widely used as a biomarker of oxidative DNA damage. In this context, a significant increase in 8-OHdG levels in the colon tissue of patients with inflammatory bowel diseases (IBDs),60 a group of pathological conditions associated with an alteration of intestinal permeability and the consequent activation of immune system response and inflammatory processes has been documented.61 Thus, a reduction in oxidative stress at the level of the gut barrier could provide protection against intestinal cell apoptosis and preserve morphological features such as villi and crypt.62 Differently from what was observed for PC, WB supplementation was able to reduce the levels of 8-OHdG. This reduction may in part explain the overall improvement of the barrier integrity and degree of permeabilization observed. Although different human studies demonstrated the capacity of berries, and in particular anthocyanins, to reduce the levels of 8-OHdG,14,63–65 to the best of our knowledge, the current study was the first one showing the effect of WB to hinder an oxidative process directly at the level of intestinal cells.
5 Conclusions
In summary, the present findings suggest that dietary achievable doses of WB and its bioactive components may be potentially valuable in the maintenance of TJs physiology and intestinal barrier health. In fact, in our in vitro model of Caco-2 cells, the treatment with WB could preserve the intestinal barrier integrity and permeability as documented by an increase in TEER and a decrease in FITC. In addition, this was associated with reduced levels of 8-OHdG and the preservation of the integral membrane protein claudin-1. These observations seem to corroborate our previous findings on the contribution of a polyphenol-rich dietary pattern in the modulation of intestinal permeability and add, for the first time, evidence about the potential role of WB. Since this represents the first study, additional in vitro evidence would help to corroborate our findings and reveal potential further mechanisms of action. Finally, an important future step would be to test the efficacy of WB in vivo. In fact, the demonstration of a functional effect of WB in the context of IP in humans could open the possibility to include its use, alone or in combination with other polyphenol-rich foods, as a potential dietary strategy to prevent and/or treat intestinal disorders.
Author contributions
Conceptualization, C. D. B.; methodology, C. D. B. and M. M.; software, M. M.; formal analysis, M. M. and C. G.; investigation, M. M., S. V. and M. R.; resources, C. D. B.; data curation, M. M. and S. V.; writing – original draft preparation, M. M. and S. V.; writing – review and editing, C. D. B., P. R., D. K. Z. and M. P.; supervision, C. D. B., P. R., and M. P.; funding acquisition, C. D. B. All authors have read and agreed to the published version of the manuscript.
Conflicts of interest
The authors declare no conflict of interest.
Acknowledgements
This work was supported by a contribution of the “Piano di sostegno alla ricerca-Linea 2, azione A-grant number PSR2021-CDELB”. Cristian Del Bo’, Dorothy Klimis-Zacas and Patrizia Riso thank the Wild Blueberry Association of North America (WBANA) for the support.
The results provided in the present manuscript were obtained in the DeFENS Cell Culture Laboratory (University of Milan, Italy).
References
- M. Vancamelbeke and S. Vermeire, The intestinal barrier: a fundamental role in health and disease, Expert Rev. Gastroenterol. Hepatol., 2017, 11, 821–834 CrossRef CAS PubMed.
- K. R. Groschwitz and S. P. Hogan, Intestinal barrier function: Molecular regulation and disease pathogenesis, J. Allergy Clin. Immunol., 2009, 124, 3–20 CrossRef CAS PubMed.
- C. M. Niessen, Tight Junctions/Adherens Junctions: Basic Structure and Function, J. Invest. Dermatol., 2007, 127, 2525–2532 CrossRef CAS PubMed.
- G. Meşe, G. Richard and T. W. White, Gap Junctions: Basic Structure and Function, J. Invest. Dermatol., 2007, 127, 2516–2524 CrossRef PubMed.
- Y. Y. Koh, W. K. Jeon, Y. K. Cho, H. J. Kim, W. G. Chung, C. U. Chon, T. Y. Oh and J. H. Shin, The Effect of Intestinal Permeability and Endotoxemia on the Prognosis of Acute Pancreatitis, Gut Liver, 2012, 6, 505–511 CrossRef CAS PubMed.
- N. di Tommaso, A. Gasbarrini and F. R. Ponziani, Intestinal Barrier in Human Health and Disease, Int. J. Environ. Res. Public Health, 2021, 18, 12836 CrossRef CAS PubMed.
- C. Graziani, R. Talocco, R. de Sire, V. Petito, L. R. Lopetuso, J. Gervasoni, S. Persichilli, F. Franceschi, V. Ojetti, A. Gasbarrini and F. Scaldaferri, Intestinal permeability in physiological and pathological conditions: major determinants and assessment modalities, Eur. Rev. Med. Pharmacol. Sci., 2019, 23, 795–810 CAS.
- C. Del Bo’, S. Bernardi, A. Cherubini, M. Porrini, G. Gargari, N. Hidalgo-Liberona, R. González-Domínguez, R. Zamora-Ros, G. Peron, M. Marino, L. Gigliotti, M. S. Winterbone, B. Kirkup, P. A. Kroon, C. Andres-Lacueva, S. Guglielmetti and P. Riso, A polyphenol-rich dietary pattern improves intestinal permeability, evaluated as serum zonulin levels, in older subjects: The MaPLE randomised controlled trial, Clin. Nutr., 2021, 40, 3006–3018 CrossRef PubMed.
- J. A. Sierra, J. S. Escobar, V. Corrales-Agudelo, O. J. Lara-Guzmán, E. P. Velásquez-Mejía, J. C. Henao-Rojas, A. Caro-Quintero, F. Vaillant and K. Muñoz-Durango, Consumption of golden berries (Physalis peruviana L.) might reduce biomarkers of oxidative stress and alter gut permeability in men without changing inflammation status or the gut microbiota, Int. Food Res. J., 2022, 162, 111949 CrossRef CAS PubMed.
- S. Bernardi, C. Del Bo’, M. Marino, G. Gargari, A. Cherubini, C. Andrés-Lacueva, N. Hidalgo-Liberona, G. Peron, R. González-Dominguez, P. Kroon, B. Kirkup, M. Porrini, S. Guglielmetti and P. Riso, Polyphenols and Intestinal Permeability: Rationale and Future Perspectives, J. Agric. Food Chem., 2020, 68, 1816–1829 CrossRef CAS PubMed.
- G. Peron, N. Hidalgo-Liberona, R. González-Domínguez, M. Garcia-Aloy, S. Guglielmetti, S. Bernardi, B. Kirkup, P. A. Kroon, A. Cherubini, P. Riso and C. Andrés-Lacueva, Exploring the Molecular Pathways Behind the Effects of Nutrients and Dietary Polyphenols on Gut Microbiota and Intestinal Permeability: A Perspective on the Potential of Metabolomics and Future Clinical Applications, J. Agric. Food Chem., 2020, 68, 1780–1789 CrossRef CAS PubMed.
- C. Rodriguez-Saona, C. Vincent and R. Isaacs, Blueberry IPM: Past Successes and Future Challenges, Annu. Rev. Entomol., 2019, 64, 95–114 CrossRef CAS PubMed.
- C. Del Bo’, V. Deon, J. Campolo, C. Lanti, M. Parolini, M. Porrini, D. Klimis-Zacas and P. Riso, A serving of blueberry (V. corymbosum) acutely improves peripheral arterial dysfunction in young smokers and non-smokers: two randomized, controlled, crossover pilot studies, Food Funct., 2017, 8, 4108–4117 RSC.
- D. Martini, M. Marino, S. Venturi, M. Tucci, D. Klimis-Zacas, P. Riso, M. Porrini and C. Del Bo’, Blueberries and their bioactives in the modulation of oxidative stress, inflammation and cardio/vascular function markers: a systematic review of human intervention studies, J. Nutr. Biochem., 2023, 111, 109154 CrossRef CAS PubMed.
- V. Taverniti, D. Fracassetti, C. Del Bo’, C. Lanti, M. Minuzzo, D. Klimis-Zacas, P. Riso and S. Guglielmetti, Immunomodulatory Effect of a Wild Blueberry Anthocyanin-Rich Extract in Human Caco-2 Intestinal Cells, J. Agric. Food Chem., 2014, 62, 8346–8351 CrossRef CAS PubMed.
- C. Del Bo’, M. Marino, P. Riso, P. Møller and M. Porrini, Anthocyanins and metabolites resolve TNF-α-mediated production of E-selectin and adhesion of monocytes to endothelial cells, Chem.-Biol. Interact., 2019, 300, 49–55 CrossRef PubMed.
- C. Del Bo’, M. Roursgaard, M. Porrini, S. Loft, P. Møller and P. Riso, Different effects of anthocyanins and phenolic acids from wild blueberry (Vaccinium angustifolium) on monocytes adhesion to endothelial cells in a TNF-α stimulated proinflammatory environment, Mol. Nutr. Food Res., 2016, 60, 2355–2366 CrossRef PubMed.
- S. Vendrame, A. Daugherty, A. S. Kristo and D. Klimis-Zacas, Wild blueberry (Vaccinium angustifolium)-enriched diet improves dyslipidaemia and modulates the expression of genes related to lipid metabolism in obese Zucker rats, Br. J. Nutr., 2014, 111, 194–200 CrossRef CAS PubMed.
- C. Del Bo’, Y. Cao, M. Roursgaard, P. Riso, M. Porrini, S. Loft and P. Møller, Anthocyanins and phenolic acids from a wild blueberry (Vaccinium angustifolium) powder counteract lipid accumulation in THP-1-derived macrophages, Eur. J. Nutr., 2016, 55, 171–182 CrossRef PubMed.
- T. Bloedon, S. Vendrame, J. Bolton, R. Lehnhard, P. Riso and D. Klimis-Zacas, The effect of wild blueberry (Vaccinium angustifolium) consumption on oxidative stress, inflammation, and DNA damage associated with exercise, Comp. Exerc. Physiol., 2015, 11, 173–181 CrossRef.
- P. Riso, D. Klimis-Zacas, C. Del Bo’, D. Martini, J. Campolo, S. Vendrame, P. Møller, S. Loft, R. De Maria and M. Porrini, , Effect of a wild blueberry (Vaccinium angustifolium) drink intervention on markers of oxidative stress, inflammation and endothelial function in humans with cardiovascular risk factors, Eur. J. Nutr., 2013, 52, 949–961 CrossRef CAS PubMed.
- P. Tsakiroglou, J. Weber, S. Ashworth, C. Del Bo and D. Klimis-Zacas, Phenolic and anthocyanin fractions from wild blueberries (V. angustifolium) differentially modulate endothelial cell migration partially through RHOA and RAC1, J. Cell. Biochem., 2019, 120, 11056–11067 CrossRef CAS PubMed.
- P. Tsakiroglou, J. Weber, S. Ashworth, C. Del Bo’ and D. Klimis-Zacas, Angiogenesis is Differentially Modulated by Anthocyanin and Phenolic Acid Extracts from Wild Blueberry (V. angustifolium) Through PI3K Pathway, J. Med. Food, 2021, 24, 226–235 CrossRef CAS PubMed.
- C. Del Bo’, A. S. Kristo, A. Z. Kalea, S. Ciappellano, P. Riso, M. Porrini and D. Klimis-Zacas, The temporal effect of a wild blueberry (Vaccinium angustifolium)-enriched diet on vasomotor tone in the Sprague-Dawley rat, Nutr. Metab. Cardiovasc. Dis., 2012, 22, 127–132 CrossRef PubMed.
- A. S. Kristo, A. Z. Kalea, D. A. Schuschke and D. Klimis-Zacas, Attenuation of alpha-adrenergic-induced vasoconstriction by dietary wild blueberries (Vaccinium angustifolium) is mediated by the NO-cGMP pathway in spontaneously hypertensive rats (SHRs), Int. J. Food Sci. Nutr., 2013, 64, 979–987 CrossRef CAS PubMed.
- S. Vendrame, A. S. Kristo, D. A. Schuschke and D. Klimis-Zacas, Wild blueberry consumption affects aortic vascular function in the obese Zucker rat, Appl. Physiol., Nutr., Metab., 2014, 39, 255–261 CrossRef CAS PubMed.
- S. Vendrame, A. Zhao, T. Merrow and D. Klimis-Zacas, The Effects of Wild Blueberry Consumption on Plasma Markers and Gene Expression Related to Glucose Metabolism in the Obese
Zucker Rat, J. Med. Food, 2015, 18, 619–624 CrossRef CAS PubMed.
- S. Vendrame, S. Guglielmetti, P. Riso, S. Arioli, D. Klimis-Zacas and M. Porrini, Six-Week Consumption of a Wild Blueberry Powder Drink Increases Bifidobacteria in the Human Gut, J. Agric. Food Chem., 2011, 59, 12815–12820 CrossRef CAS PubMed.
- S. Guglielmetti, D. Fracassetti, V. Taverniti, C. Del Bo’, S. Vendrame, D. Klimis-Zacas, S. Arioli, P. Riso and M. Porrini, Differential Modulation of Human Intestinal Bifidobacterium Populations after Consumption of a Wild Blueberry (Vaccinium angustifolium) Drink, J. Agric. Food Chem., 2013, 61, 8134–8140 CrossRef PubMed.
- S. E. Pritchard, L. Marciani, K. C. Garsed, C. L. Hoad, W. Thongborisute, E. Roberts, P. A. Gowland and R. C. Spiller, Fasting and postprandial volumes of the undisturbed colon: normal values and changes in diarrhea–predominant irritable bowel syndrome measured using serial MRI, Neurogastroenterol. Motil., 2014, 26, 124–130 CrossRef CAS PubMed.
- A. Rodriguez-Mateos, R. P. Feliciano, T. Cifuentes-Gomez and J. P. E. Spencer, Bioavailability of wild blueberry (poly)phenols at different levels of intake, J. Berry Res., 2016, 6, 137–148 CAS.
- P. J. Curtis, L. Berends, V. van der Velpen, A. Jennings, L. Haag, P. Chandra, C. D. Kay, E. B. Rimm and A. Cassidy, Blueberry anthocyanin intake attenuates the postprandial cardiometabolic effect of an energy-dense food challenge: Results from a double blind, randomized controlled trial in metabolic syndrome participants, Clin. Nutr., 2022, 41, 165–176 CrossRef CAS PubMed.
- C. Gardana and P. Simonetti, Evaluation of the Degree of Polymerization of the Proanthocyanidins in Cranberry by Molecular Sieving and Characterization of the Low Molecular Weight Fractions by UHPLC-Orbitrap Mass Spectrometry, Molecules, 2019, 24, 1504 CrossRef CAS PubMed.
-
AOAC Method 991.43, Total, insoluble and soluble dietary fiber in food-enzymatic-gravimetric method, MES-TRIS bufferr. Official Methods of Analysis, AOAC International, Gaithersburg, MD, USA, 16th edn, 1995 Search PubMed.
- N. F. Abo El-Magd, P. O. Barbosa, J. Nick, V. Covalero, G. Grignetti and G. Bermano, Selenium, as selenite, prevents adipogenesis by modulating selenoproteins gene expression and oxidative stress–related genes, Nutrition, 2022, 93, 111424 CrossRef CAS PubMed.
- M. Marino, C. Gardana, A. Scialpi, G. Giorgini, P. Simonetti and C. Del Bo’, An in vitro approach to study the absorption of a new oral formulation of berberine, PharmaNutrition, 2021, 18, 100279 CrossRef CAS.
- E. Cremonini, A. Mastaloudis, S. N. Hester, S. V. Verstraeten, M. Anderson, S. M. Wood, A. L. Waterhouse, C. G. Fraga and P. I. Oteiza, Anthocyanins inhibit tumor necrosis alpha-induced loss of Caco-2 cell barrier integrity, Food Funct., 2017, 8, 2915–2923 RSC.
- M. A. Polewski, D. Esquivel-Alvarado, N. S. Wedde, C. G. Kruger and J. D. Reed, Isolation and Characterization of Blueberry Polyphenolic Components and Their Effects on Gut Barrier Dysfunction, J. Agric. Food Chem., 2020, 68, 2940–2947 CrossRef CAS PubMed.
- E. Cremonini, Z. Wang, A. Bettaieb, A. M. Adamo, E. Daveri, D. A. Mills, K. M. Kalanetra, F. G. Haj, S. Karakas and P. I. Oteiza, (-)-Epicatechin protects the intestinal barrier from high fat diet-induced permeabilization: Implications for steatosis and insulin resistance, Redox Biol., 2018, 14, 588–599 CrossRef CAS PubMed.
- T. Suzuki, S. Tanabe and H. Hara, Kaempferol Enhances Intestinal Barrier Function through the Cytoskeletal Association and Expression of Tight Junction Proteins in Caco-2 Cells, J. Nutr., 2011, 141, 87–94 CrossRef CAS PubMed.
- S. Noda, S. Tanabe and T. Suzuki, Differential Effects of Flavonoids on Barrier Integrity in Human Intestinal Caco-2 Cells, J. Agric. Food Chem., 2012, 60, 4628–4633 CrossRef CAS PubMed.
- S. Noda, S. Tanabe and T. Suzuki, Naringenin enhances intestinal barrier function through the expression and cytoskeletal association of tight junction proteins in Caco-2 cells, Mol. Nutr. Food Res., 2013, 57, 2019–2028 CrossRef CAS PubMed.
- J. Remenyik, A. Biró, Á. Klusóczki, K. Z. Juhász, T. Szendi-Szatmári, Á. Kenesei, E. Szőllősi, G. Vasvári, L. Stündl, F. Fenyvesi, J. Váradi and A. Markovics, Comparison of the Modulating Effect of Anthocyanin-Rich Sour Cherry Extract on Occludin and ZO-1 on Caco-2 and HUVEC Cultures, Int. J. Mol. Sci., 2022, 23, 9036 CrossRef CAS PubMed.
- D. Taladrid, D. González de Llano, I. Zorraquín-Peña, A. Tamargo, M. Silva, N. Molinero, M. V. Moreno-Arribas and B. Bartolomé, Gastrointestinal Digestion of a Grape Pomace Extract: Impact on Intestinal Barrier Permeability and Interaction with Gut Microbiome, Nutrients, 2021, 13, 2467 CrossRef CAS PubMed.
- T. Wu, Z. Jiang, J. Yin, H. Long and X. Zheng, Anti-obesity effects of artificial planting blueberry (Vaccinium ashei) anthocyanin in high-fat diet-treated mice, Int. J. Food Sci. Nutr., 2016, 67, 257–264 CrossRef CAS PubMed.
- J. DeFuria, G. Bennett, K. J. Strissel, W. P. James, P. E. Milbury, A. S. Greenberg and M. S. Obin, Dietary Blueberry Attenuates Whole-Body Insulin Resistance in High Fat-Fed Mice by Reducing Adipocyte Death and Its Inflammatory Sequelae, J. Nutr., 2009, 139, 1510–1516 CrossRef CAS PubMed.
- J. C. Valdez, J. Cho and B. W. Bolling, Aronia berry inhibits disruption of Caco-2 intestinal barrier function, Arch. Biochem. Biophys., 2020, 688, 108409 CrossRef CAS PubMed.
- K. J. Kim, Y. Kim, S. G. Jin and J. Y. Kim, Acai berry extract as a regulator of intestinal inflammation pathways in a Caco–2 and RAW 264.7 co-culture model, J. Food Biochem., 2021, 45, e13848 CAS.
- R. Singh, S. Chandrashekharappa, S. R. Bodduluri, B. V. Baby, B. Hegde, N. G. Kotla, A. A. Hiwale, T. Saiyed, P. Patel, M. Vijay-Kumar, M. G. I. Langille, G. M. Douglas, X. Cheng, E. C. Rouchka, S. J. Waigel, G. W. Dryden, H. Alatassi, H.-G. Zhang, B. Haribabu, P. K. Vemula and V. R. Jala, Enhancement of the gut barrier integrity by a microbial metabolite through the Nrf2 pathway, Nat. Commun., 2019, 10, 89 CrossRef PubMed.
- P. Lian, P. A. J. Henricks, H. J. Wichers, G. Folkerts and S. Braber, Differential Effects of Oligosaccharides, Antioxidants, Amino Acids and PUFAs on Heat/Hypoxia-Induced Epithelial Injury in a Caco-2/HT-29 Co-Culture Model, Int. J. Mol. Sci., 2023, 24, 1111 CrossRef CAS PubMed.
- E. Cremonini, E. Daveri, A. Mastaloudis, A. M. Adamo, D. Mills, K. Kalanetra, S. N. Hester, S. M. Wood, C. G. Fraga and P. I. Oteiza, Anthocyanins protect the gastrointestinal tract from high fat diet-induced alterations in redox signaling, barrier integrity and dysbiosis, Redox Biol., 2019, 26, 101269 CrossRef CAS PubMed.
- M. C. Rodríguez-Daza, L. Daoust, L. Boutkrabt, G. Pilon, T. Varin, S. Dudonné, É. Levy, A. Marette, D. Roy and Y. Desjardins, Wild blueberry proanthocyanidins shape distinct gut microbiota profile and influence glucose homeostasis and intestinal phenotypes in high-fat high-sucrose fed mice, Sci. Rep., 2020, 10, 2217 CrossRef PubMed.
- Z. Yan, F. Yang, Z. Hong, S. Wang, Z. Jinjuan, B. Han, R. Xie, F. Leng and Q. Yang, Blueberry Attenuates Liver Fibrosis, Protects Intestinal Epithelial Barrier, and Maintains Gut Microbiota Homeostasis, Can J. Gastroenterol. Hepatol., 2019, 2019, 1–11 CrossRef PubMed.
- Y. Cheng, S. Tang, T. Wu, S. Pan and X. Xu, Lactobacillus casei-fermented blueberry pomace ameliorates colonic barrier function in high fat diet mice through MAPK-NF-κB-MLCK signaling pathway, J. Funct. Foods, 2022, 95, 105139 CrossRef CAS.
- L. Felgus-Lavefve, L. Howard, S. H. Adams and J. I. Baum, The Effects of Blueberry Phytochemicals on Cell Models of Inflammation and Oxidative Stress, Adv. Nutr., 2022, 13, 1279–1309 CrossRef PubMed.
- D. I. Sonnier, S. R. Bailey, R. M. Schuster, A. B. Lentsch and T. A. Pritts, TNF-α Induces Vectorial Secretion of IL-8 in Caco-2 Cells, J. Gastrointest. Surg., 2010, 14, 1592–1599 CrossRef PubMed.
- M. Fedorova, R. C. Bollineni and R. Hoffmann, Protein carbonylation as a major hallmark of oxidative damage: update of analytical strategies, Mass Spectrom. Rev., 2014, 33, 79–97 CrossRef CAS PubMed.
- Y. Wang, Y. Chen, X. Zhang, Y. Lu and H. Chen, New insights in intestinal oxidative stress damage and the health intervention effects of nutrients: A review, J. Funct. Foods, 2020, 75, 104248 CrossRef CAS.
- A. Watari, M. Hasegawa, K. Yagi and M. Kondoh, Checkpoint Kinase 1 Activation Enhances Intestinal Epithelial Barrier Function via Regulation of Claudin-5 Expression, PLoS One, 2016, 11, e0145631 CrossRef PubMed.
- R. D'Incà, R. Cardin, L. Benazzato, I. Angriman, D. Martines and G. C. Sturniolo, Oxidative DNA Damage in the Mucosa of Ulcerative Colitis Increases With Disease Duration and Dysplasia, Inflammatory Bowel Dis., 2004, 10, 23–27 CrossRef PubMed.
- A. Michielan and R. D'Incà, Intestinal Permeability in Inflammatory Bowel Disease: Pathogenesis, Clinical Evaluation, and Therapy of Leaky Gut, Mediators Inflammation, 2015, 2015, 1–10 CrossRef PubMed.
- X. Cai, F. Yang, L. Zhu, Y. Xia, Q. Wu, H. Xue and Y. Lu, Rosmarinic Acid, the Main Effective Constituent of Orthosiphon stamineus, Inhibits Intestinal Epithelial Apoptosis Via Regulation of the Nrf2 Pathway in Mice, Molecules, 2019, 24, 3027 CrossRef CAS PubMed.
- L. A. Kresty, W. L. Frankel, C. D. Hammond, M. E. Baird, J. M. Mele, G. D. Stoner and J. J. Fromkes, Transitioning From Preclinical to Clinical Chemopreventive Assessments of Lyophilized Black Raspberries: Interim Results Show Berries Modulate Markers of Oxidative Stress in Barrett's Esophagus Patients, Nutr. Cancer, 2006, 54, 148–156 CrossRef CAS PubMed.
- C. Del Bo’, M. Porrini, J. Campolo, M. Parolini, C. Lanti, D. Klimis-Zacas and P. Riso, A single blueberry (Vaccinium corymbosum) portion does not affect markers of antioxidant defence and oxidative stress in healthy volunteers following cigarette smoking, Mutagenesis, 2016, 31, 215–224 CrossRef PubMed.
- S. A. Johnson, R. G. Feresin, N. Navaei, A. Figueroa, M. L. Elam, N. S. Akhavan, S. Hooshmand, S. Pourafshar, M. E. Payton and B. H. Arjmandi, Effects of daily blueberry consumption on circulating biomarkers of oxidative stress, inflammation, and antioxidant defense in postmenopausal women with pre- and stage 1-hypertension: a randomized controlled trial, Food Funct., 2017, 8, 372–380 RSC.
|
This journal is © The Royal Society of Chemistry 2023 |
Click here to see how this site uses Cookies. View our privacy policy here.