DOI:
10.1039/D2LC00873D
(Critical Review)
Lab Chip, 2023,
23, 1128-1150
Beyond biology: alternative uses of cantilever-based technologies
Received
21st September 2022
, Accepted 25th November 2022
First published on 13th January 2023
Abstract
Micromechanical cantilever sensors are attracting a lot of attention because of the need for characterizing, detecting, and monitoring chemical and physical properties, as well as compounds at the nanoscale. The fields of application of micro-cantilever sensors span from biological and point-of-care, to military or industrial sectors. The purpose of this work focuses on thermal and mechanical characterization, environmental monitoring, and chemical detection, in order to provide a technical review of the most recent technical advances and applications, as well as the future prospective of micro-cantilever sensor research.
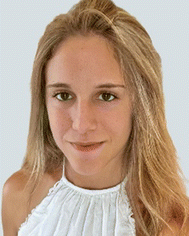 Helena Muñoz-Galán | Helena Muñoz-Galán got her MCs in Nanotechnology from Universitat de Barcelona in 2017. She is currently a PhD student at the Universitat Politècnica de Catalunya-BarcelonaTech (UPC). Her PhD in Polymers and Biopolymers is being supervised by Dr. Maria M. Pérez-Madrigal and Prof. Carlos Alemán (UPC). Her research hub is focused on developing a functional and sustainable device for glycemic control minimizing the required invasion of current treatments. |
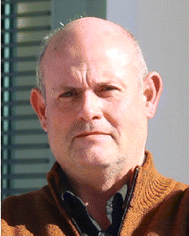 Carlos Alemán | Carlos Alemán graduated with a degree in Chemistry at the University of Barcelona (Spain). He received his Ph.D. from Universitat Politècnica de Catalunya-BarcelonaTech (UPC) in 1994, where he was promoted to the position of Full Professor. He was a postdoctoral researcher at the ETH and a visiting professor at the Università di Napoli Federico II, University of Twente and Universidade Federal do Rio Grande do Sul. Since 2003 he has been the leader of the “Innovation in Materials and Molecular Engineering” group. His main research interests focus on polymers for biomedical applications, especially biosensors, energy storage devices and, most recently, the development of electrothermal catalysts. |
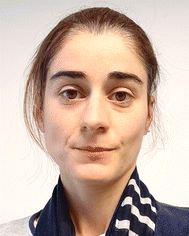 Maria M. Pérez-Madrigal | Maria M. Pérez-Madrigal is a Beatriz Galindo Fellow and lecturer at the Chemical Department, at Universitat Politècnica de Catalunya-BarcelonaTech (UPC). She obtained her Ph.D. in Polymers and Biopolymers in 2015 under the supervision of Prof. Elaine Armelin and Prof. Carlos Alemán (UPC). After receiving her Ph.D., she carried out a postdoctoral research stay at the University of Warwick (2016–2018) and, later, at the University of Birmingham (2018–2020). Her research aims at developing novel and functional (bio)material platforms for technical and biomedical applications by exploiting the benefits of polymer chemistry, biopolymers, and hydrogel-based systems. |
1. Introduction
In their pioneering work, Barnes et al. proposed microcantilevers as a new class of sensors for a wide range of applications.1 Almost three decades later, highly sensitive biological, physical and chemical sensor devices have been developed based on cantilever-based technologies.2 Although the detection of biomolecules, bacteria, and viruses is still the predominant use, the application of nanomechanical sensors has broadened including other applications, such as electronics, biophysics, automotive and aerospace systems, environmental monitoring, and medical diagnosis devices, among others.3 Indeed, advances made in microfabrication tools and manufacturing methods have allowed real-time sensing at the micro- and nanoscale with higher sensitivity, better compactness and lower limits of detection in comparison to standard diagnostic assays. Despite this, as revised by Calleja et al.,4 nanomechanical biosensing still faces some challenges and constraints and has not been fully accepted yet as an alternative to other well established bioanalytical techniques (i.e. ELISA, microarrays or electrophoresis methods).
Microcantilevers (approximately 100–200 μm long, 20–40 μm wide, and 0.5–1 μm thick, and typically made of silicon or silicon nitride) remain unequaled when responding to the demanding requirements of biosensing applications, where fast, straightforward, cost-effective, and reliable methods are desirable for the recognition of molecules. On such terms, back in 2011, Buchapudi et al. reported that nearly 50 analytes, chemical and biological, had been successfully detected by cantilever sensors.5 From a more general point of view, cantilever-based technologies are still attracting growing interest on account of their features, which include cheap and easy batch-fabrication, high sensitivity and specificity, short time response, multiplexing and functionalization capability, easy integration in electronic circuits (silicon technology) and versatility, among others. Hence, the design of robust cantilever-like sensors is vital to reach novel applications and progress in other aspects, such as device miniaturization, power consumption, sensor architecture, and compactness.
Considering the relevance and importance of this field of study, we find in the literature abundant reviews that deal with nanomechanical biosensing.6,7 Specifically, we direct the reader to the following: Ziegler provided a general introduction into the theory of cantilever sensors, including practical design concepts,8 whereas the basis of nanomechanical sensors, materials and fabrication procedures have been also described.9,10
Microcantilever-based sensors appeared as an innovative application of micromechanical analysis methods (i.e. scanning force microscopy, SFM). In those, the small forces, attractive and/or repulsive, between a surface sample and a sharp tip on a cantilever (thin beam clamped at one side) are measured.11 Specifically, the forces bend the cantilever, which acts as a force transducer. Being able to measure piconewtons, the applications of SFM span from topography reconstruction and single molecule detection12 to intramolecular force measurements,13 among many others.14,15 Although the results are susceptible to ambient parameters, such as temperature, pressure, or humidity when not working under vacuum conditions,16 SFM can operate in a variety of environments, which results in an attractive feature, thus enabling the simultaneous morphological and mechanical characterization of living biological systems.17
As mentioned earlier, cantilever-based devices display high sensitivity, even at the femtojoule-scale, and hence are able to detect small variations in mass, which results in a competitive technology in comparison to conventional methods.18 Therefore, such a set of high-end advantages results in a diverse range of detection applications, including cancer biomarkers,19,20 viruses,21,22 oligonucleotides,23 drug screening,24 cells,25,26 and carbohydrate–protein interactions,27 among others.
Motivated by the above considerations, in this contribution, we do not intend to exhaustively revise again the biologically related applications of micro- and nanocantilevers, which has been recently done.2 We rather focus on alternative uses and the state of the art of this technology applied to material characterization, calorimetry and innovative functions. In particular, we briefly introduce the working principle and operational considerations of nanomechanical sensors. Then, we summarize recent examples of nanomechanical sensors beyond biological applications and, finally, discuss the current trends and perspectives of this field of study. By doing so, we fully explore the scope of micro- and nanocantilevers and present them as really versatile devices for advanced applications.
2. Technical overview
2.1. Working principle and operation modes
Microcantilevers are flat, horizontal structures, usually rectangular, that have one free end and are clamped at the other end to a wafer, and act as force transducers where the recognition event is transduced into a micromechanical motion. Specifically, the cantilever transduction principles can be summarized in the following three working modes (Fig. 1a): i) surface stress sensor (the cantilever bends due to changes in the surface stress at one of its sides – static mode); ii) microbalance (the frequency changes as a consequence of additional mass loading – dynamic mode); and, finally, iii) the bimetallic effect (the cantilever bends as a temperature sensor).28–31 Hence, the nanomechanical information obtained from each one of these three different operation modes can be combined, thus providing a better understanding of the sensing event and proving the high versatility of cantilever-based devices.
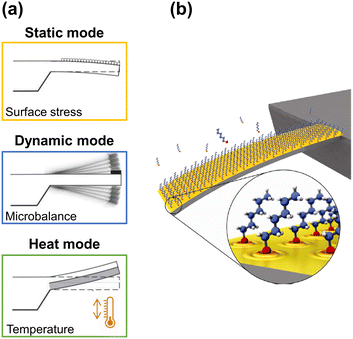 |
| Fig. 1 (a) Working modes of cantilever-based sensors. Adapted from ref. 32 (under a Creative Commons license CC BY-NC-ND 3.0); (b) artistic view of an alkanethiol self-assembled monolayer (SAM) on a gold-coated microcantilever formed from the gas phase. Reprinted with permission from reference Nanotechnology, 2010, 21, 075501.33 Copyright 2010 IOP Publishing. | |
Briefly, in the static mode, the deflection profile of the cantilever is obtained: the cantilever bends as a result of the change in surface stress between the active side and the non-activated side of the cantilever (Fig. 1b). Such differential surface stress arises from molecular redistribution events (for instance, the electron cloud on the surface33) or by a molecular adsorption process,34–36 where steric interactions, electrostatic interactions, hydrogen bonding, or de-solvation interactions take place.37,38 Additionally, electric or magnetic fluctuations,39 as well as chemical reactions, can also cause the deflection of the cantilever.40
In contrast, in the dynamic mode, the cantilever is excited at its resonance frequency with a piezoelectric actuator system. When mass is adsorbed on the cantilever, the resonance frequency of the cantilever changes to lower frequencies, and the readable shift quantifies the adsorbed mass (1 Hz corresponds to approximately 1 picogram if the mechanical properties of the cantilever are not significantly affected), while changes in the shape of the resonant frequency profile can indicate chemical or physical events.41,42
Finally, in the remaining mode, the heating mode, which is based on the bimetallic effect,43,44 the difference in the thermal expansion coefficients of the materials that compose a cantilever results in its bending when the temperature differs from room temperature, either by cooling or heating. Even increments of the order of 10−5 K enable the cantilever to bend by some nanometers.45
Moreover, the mechanical properties of the cantilever, which affect the optimization of both the sensitivity and geometry,46,47 are conditioned by five variables at the micrometric scale: the Poisson's ratio, the Young's modulus, which is directly related to the stiffness of the material, the yield of strength, the fracture strength, and, finally, the residual stress.
It is beyond the scope of this review to describe in detail the mathematical models that govern the physics behind each recognition event, as it has been done before48–52 and, by this reason, we direct the reader to comprehensive studies on this matter.53–55 Before concluding this section, though, we highlight a series of recent advances and aspects we find to be of relevant importance as they relate to the application of the cantilever-based technology. For instance, Gao et al. reported a combined theoretical and experimental investigation on effective excitation of microcantilevers by using photoacoustic waves – a universal method used for the detection of small signals in which detrimental thermal effects must be avoided.56
Considering the influence of harsh environmental conditions on the response of silicon microcantilever sensors, several investigations focused on innovative materials and cantilever shapes that allow the cantilever sensors to work under high temperature conditions.57 For instance, Dounkal et al. studied the effect of axial compressive force, tensile forces, stress gradient and transverse loading on gallium nitride and silicon carbide cantilevers that will improve the performance of cantilever sensors in harsh environments, i.e. extreme temperatures.58
Finally, the detection of volatile organic compounds (VOCs), explosives, and pesticides, as well as biomolecules, usually requires the functionalization of the cantilever. McCaig et al. investigated a way to enhance the adsorption of vapors by a uniform polymer-brush coating based on PMMA, which was produced by surface-initiated atom-transfer radical polymerization.59 Other studies also applied a similar approach to design microcantilevers with end-grafted polymer brushes for actuation and sensing.60,61
2.2. Materials and processing
Usually, cantilevers are made of silicon, silicon nitride, silicon oxide, or a polymeric material. Silicon is used for single layer cantilevers, while polymer coatings based on polyethylene terephthalate (PET) or SU-8, a commonly used epoxy-based negative photoresist, have been applied to modify the surface properties of the cantilevers.62,63
Notably, in a short period, cantilever sensors have been able to sense from picograms to attograms by modifying the properties of the cantilever (resonance frequency, stiffness, geometry, etc.) and optimizing the fabrication process (scaling down).49,50,53 Indeed, the complex interplay between all the design parameters involved affects the sensing performance (i.e. sensitivity) to the addition of material. In fact, although rectangular cantilevers are the most commonly used, other geometries might be also taken into consideration in terms of achieving sensitive devices, with larger frequency responses.55 Size and especially thickness are important in that a high surface to volume ratio increases sensitivity.69
The material of choice to construct the cantilever device also determines its resonant response. For instance, the resonance frequency (f) increases with increasing spring constant, which is proportional to the flexural rigidity of the cantilever (i.e. product of the Young's modulus and the moment of inertia), as well as with decreasing cantilever mass.49,50,53 In general, since the Young's modulus values of polymers are lower than the one shown by silicon, which is in the order of 100 GPa, the stiffness of a microcantilever significantly decreases when using a polymeric material, whereas its fabrication process is easier and more affordable. The limitation of these kinds of cantilevers relies on their temperature sensitivity. For this reason, it is vital to keep the environmental conditions under strict control.43
The processing of the cantilevers is usually done by bulk micromachining64 or LIGA65 (German acronym for lithography, plating and molding), and all of them involve photolithography and dry-etching. As advantage, these methods facilitate multiplexing the fabrication of hundreds of microcantilevers at a very low cost, and with a very high shape accuracy, which is one of the reasons for which microcantilever-based sensors have attracted much attention. For instance, in the field of genetics and drug discovery, hundreds of replications are required for thousands of interactions.66,67 Finally, as mentioned earlier, although the shape of microcantilevers is usually rectangular, some studies have reported the benefits of other shapes68 and proportions.69–72
2.3. Detection methods
The subnanometric accuracy of the cantilever technology is achieved by measuring the bending of the cantilever. Although the miniaturization in the size of the cantilever enables the detection of smaller amounts than attograms of absorbate,47 the sensitivity of the readout system has been an issue for the past few years.73
To measure the resonance frequency of the cantilever and its deflection, different readout methods have been used to transduce these magnitudes into an electrical signal. Among the several available possibilities based on piezoresistivity, piezoelectricity, or interferometry, for example, the most used is the optical technique since it presents a better resolution (i.e. in the order of angstroms).74 Specifically, in this technique, a low power laser beam is projected onto the active side of a microcantilever, where it is reflected off and projected to a photodetector (PSD). When the cantilever bends, the reflected beam bends and, consequently, the projected spot on the photodetector changes its position, thus allowing the monitoring of the deflection.75,76 The main drawback of this technique relies on the fact that the laser alignment must be very precise, which requires high accuracy during the installation process. Moreover, the number of lasers involved has to match the number of cantilevers in the array, displaying the same pitch distance, as well. Besides, each of those lasers is connected to a sequential switcher source to avoid the overlapping of the beams when reflecting off onto the photodetector.
In contrast, the piezoresistive readout method does not present such a limitation since the piezoresistor is coupled with the cantilever beam, and the readout system is integrated into the chip. Here, the mechanical deformation induces a resistance in the piezoresistor that is directly transduced into an electrical signal.30,77 Unfortunately, the resolution of this method only reaches the nanometric scale.
In other methods, such as the capacitive method, the cantilever acts as one of the two plates of a capacitor, while the substrate acts as the other. Hence, the deflection of the cantilever, when an analyte is adsorbed, for instance, is monitored by the change in the capacitance value.78,79 Although this detection mode is highly sensitive, it is more limited than the others: it is not adequate for the measuring of large displacements or liquid working conditions (e.g. electrolyte solutions) because of the occurrence of faradic currents between the plates. Finally, in interferometric displacement detection, the distance between the fiber end delivering and collecting light and a highly reflective micro-cantilever forms the interferometric cavity.80 For a precise measurement of the cantilever displacement, the relative positioning of the fiber and cantilever is of critical importance.
In order to improve the sensitivity of micromechanical cantilever sensors, different cantilever shapes have been deeply compared.81,82 Recently, Gao et al. designed a new shape for the cantilever in order to improve the high order mode sensitivity for resonant mass sensors without any need for reducing dimensions.83 The sensitivity of the dynamic mode can also be tuned applying higher frequency modes, which result in the minimization of the frequency noise. The higher the frequency mode, the less sensitive the cantilever is to external vibrations.84
Other ways of improving the sensitivity have been suggested for specific systems. For example, liquid environments cause a damping effect on the cantilever resonation that significantly decreases the sensitivity of cantilever sensors. However, Linden et al. managed to produce a liquid cantilever sensor with a high quality factor by reducing the viscous damping.85 Only one side of the cantilever is exposed to the sample while the other remains under partial wetting stabilized with a clip. Besides, since cantilever sensors are used for very sensitive measurements, the calibration of the readout system needs to be reliable and simple. To make that possible, theoretical and experimental models have been developed.86,87 Finally, some cantilever-based detection systems might experience drifting problems. To alleviate this issue, some authors do equilibrate the prepared cantilevers in their buffer solutions to obtain a good baseline with little noise.
3. Microcantilever-based devices: applications
The interest regarding cantilever-based sensors appeared in the field of disease diagnostics and biomolecular research because of the possibility of functionalizing the cantilever for selective and/or specific detection. Indeed, the detection of single DNA bases and biomolecular bindings was possible by coating the cantilevers with the specific targets and, then, monitoring the deflection profile.34,88 This powerful technology also enabled the detection of molecular adsorption processes and, even, conformational changes of immobilized proteins on the surface by means of acoustic sensors.89 By measuring the mechanical responses of the cantilever, not only were molecular interactions detected, but also changes in surface stress.90
In the following sub-sections, we present advanced applications of cantilever-based devices in a wide range of fields: thermal characterization, mechanical characterization, mass detection of molecules using different approaches, humidity differential detection, absolute and differential pressure detection, and differential heat detection, among others. To show the reader the versatility and scope of application of microcantilever-based platforms, Table 1 summarizes selected studies described in the following sections.
Table 1 Scope of application of microcantilever-based platforms: examples of selected applications described in this review
Sensor application |
Description |
Mode |
Cantilever geometry/dimensions/material |
Ref. |
Mechanical and thermal properties |
Temperature-dependent thermal stress for several polymer coatings |
Static |
Rectangular silicon-triangular free end (500 μm long, 100 μm wide, and 1 μm thick; spring constant of 0.03 N m−1) |
102
|
Bimetallic |
Mechanical properties of poly(vinyl acetate) |
Static |
Rectangular silicon-triangular free end (500 μm long, 100 μm wide, and 1 μm thick; spring constant of 0.03 N m−1) |
103
|
Dynamic |
Bimetallic |
Thermal properties of PLA coatings with nanopores |
Static |
Rectangular silicon (500 μm long, 90 μm wide, and 1 μm thick) |
106
|
Bimetallic |
Humidity |
Low level of moisture by Al2O3-modified cantilevers |
Static |
V-Shaped silicon (180 μm long, 25 μm wide, and 0.5–1.5 μm thick) |
130
|
Conducting polymer-based cantilever sensors |
Static |
Rectangular silicon (350 μm long, 30 μm wide, and 1 μm thick; resonance frequency of 12 (±2) kHz, spring constant of 0.03–0.13 N m−1) |
137
|
Explosives |
Sensitive detection with SAM-coated cantilever |
Static |
V-Shaped silicon (180 μm long, 25 μm wide, and 0.5–1.5 μm thick) |
157
|
Dynamic |
Photothermal deflection spectroscopy |
Dynamic |
Rectangular silicon (350 μm long, 35 μm wide, and 1 μm thick) |
163
|
Ultralow concentrations of explosives – TiO2 nanotubes |
Dynamic |
Rectangular silicon (225 μm long, 28 μm wide, and 3 μm thick) |
176
|
Piezoresistive sensors – Cu-based nanorods |
Dynamic |
Rectangular silicon (350 μm long, 150 μm wide, and 11 μm thick) |
|
Pesticides |
Trace-level organophosphorus pesticide |
Dynamic |
Lab-made resonant rectangular silicon cantilever (200 μm long, 100 μm wide, and 3 μm thick) |
187
|
Organophosphorus compounds |
Static |
V-Shaped silicon (180 μm long, 25 μm wide, and 1 μm thick) |
188
|
CO/CO2 |
Adsorption and desorption of CO2 on amine-functionalized microcantilevers |
Dynamic |
Arrays of eight rectangular silicon (each: 450 μm long, 90 μm wide, and 5 μm thick; spring constant of 3.8 N m−1) |
192
|
Piezoresistive cantilever with Fe(III)porphyrin for CO sensing |
Static |
SU-8/carbon black nanocomposite-based cantilevers (200 μm long, 50 μm wide, and 3.5 μm thick) |
195
|
VOCs |
Quantification of individual components in a gas mixture |
Dynamic |
Polymer coated rectangular silicon (280/240 μm long, 20 μm wide, and 0.5 μm thick) |
197
|
3.1. Characterization of mechanical and thermal properties
Thermal and mechanical characterization are probably the least exploited applications of cantilever-based sensors but, at the same time, present the best prospective. The evaluation of the features displayed by micro- and nanometric samples represents an important step when optimizing their design at such scales. However, the strong scale dependence of thermomechanical properties limits the use of conventional characterization methods when considering micrometric samples. Moreover, to perform conventional experiments, large samples are required, which implies loss of homogeneity and, hence, entails low reproducibility and reliability. The evaluation of the mechanical properties of macroscopic thin film specimens has been usually carried out through internal stress measurements by wafer buckling,91 while other useful techniques, such as nanoindentation92 or nanotribology,93,94 yield information on hardness, adhesion or wear resistance. For extremely thin films, only nanomechanical characterization via cantilever-based technologies offers new possibilities regarding film characterization at the nanoscale.95
In 1994, Thundat et al. reported the behavior of a coated cantilever for which a noticeable bending was observed because of the heating produced by the laser beam.16 Nevertheless, this effect turned out to be the fundamental principle for high sensitivity thermometers. In the same work, they stated that when the temperature was kept constant, and the humidity changed, the cantilever also bended. The same year Barnes et al. reported a microcantilever sensor capable of detecting smaller heat changes than a picojoule.1,96 Indeed, compared to conventional methods (Differential Scanning Calorimetry (DSC), for instance), microcantilever thermosensors, which are based on the bimetallic effect, display high sensitivity (∼1 pJ) and rapid response time (∼1 ms), which makes them the most suitable for measuring enthalpy changes of attomolar amount samples.
Later, based on the same principle, several studies monitored the thermally induced bending of a bimaterial cantilever,97,98 or the frequency shift of a coated cantilever compared to the uncoated one.99 Hence, phase transition studies became possible on samples of few picograms.100 Based on these two phenomena, other thermal and mechanical properties of materials, such as stiffness101 (that can produce even more significant frequency shifts than mass adsorption), physical aging of polymers102 and glass transition events,103 have been studied. Generally, in these studies, the cantilever is coated with the material under study, e.g. poly(styrene)/poly(methyl methacrylate) (PS/PMMA) copolymers, polythiophene and alkanes, among others. Once the cantilever is homogeneously coated, the system is heated at a constant rate, for example 5 °C min−1. The resonance frequency, as well as the deflection of the cantilever, is monitored and compared to the uncoated cantilever. For instance, Kim et al. reported the ultraviolet (UV) radiation-induced physical, thermal, and mechanical properties of a photosensitive polymer, poly(vinyl cinnamate) (PVCN), determined by using a microcantilever sensor and a nanogram amount of material (Fig. 2).104 As bimaterial microcantilevers can be used also as highly sensitive infrared detectors,105 nanomechanical IR spectra from the same PVCN-coated silicon microcantilever were acquired as a function of UV irradiation time at room temperature. Moreover, deflection profiles revealed information about the Tg and the cross-linking degree of the photosensitive polymer as a function of time exposure to UV radiation.
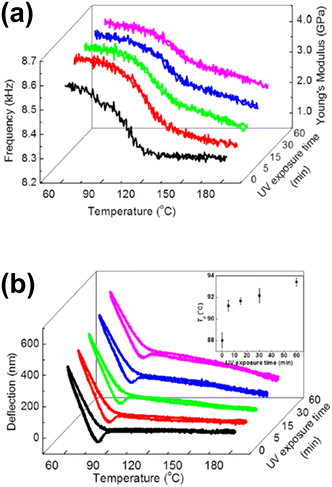 |
| Fig. 2 (a) Measured temperature-dependent shifts in the resonance frequency of PVCN-coated cantilevers and the estimated Young's modulus after various periods of UV irradiation time; (b) measured temperature-dependent variations in the deflection of PVCN-coated cantilevers after various periods of UV irradiation time. The inset shows variations in the Tg as a function of UV irradiation time. Reprinted with permission from reference Appl. Phys. Lett., 2013, 102(2), 024103.104 Copyright 2013 AIP Publishing. | |
Most recently, the thermal properties of nanomembranes, namely, glass transition and cold crystallization temperatures (Tg and Tcc, respectively), were reported by monitoring the microcantilever deflection as a function of temperature. Different poly lactic acid (PLA)-based nanomembranes with varying nanostructures (compact, nanopored, or nanoperforated) and composition (curcumin- or stiripentol-loaded) were examined.106 Overall, the presence of nanofeatures affected both the Tg and Tcc thermal features, which indicates the importance of characterizing nanomaterials with techniques that take into account scale-related considerations.
Other thermomechanical properties, such as thermal diffusivity and thermal conductivity of diamond nanocrystalline and microcrystalline structures, have been studied by photothermal excitation, which induces surface stress on a bimaterial cantilever.107
Regarding the mechanical properties of polymer brushes, to establish a direct correlation between the molecular structure and the swelling that these kinds of polymers undergo, microcantilever sensors have been applied alternatively.108–111 Attractive interactions between polymers in a brush lead to a positive bending of the cantilever, while solvent incorporation due to the swelling causes compressive stress and, consequently, the cantilever bends away from the coated side. Moreover, swelling entails a decrease in the Tg, an effect known as plasticization. Alves et al. investigated the variation in Tg as a function of humidity.112 To do so, the deflection of the cantilever at different values of humidity, from 1.4% to 70%, was monitored while increasing the temperature at a constant rate, from 25 °C to 50 °C (Fig. 3). As a result, Tg decreases from 42 °C (1.4%) down to 34 °C (70%). In line with these investigations, the influence of pH in the swelling of other polymeric materials, such as polymethacryloyl ethylene phosphate brushes (PMEP) or poly(methacrylic acid) hydrogels, was also examined.113,114
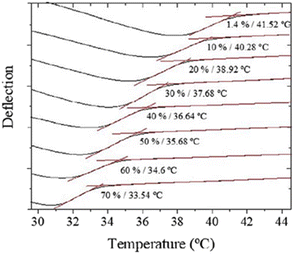 |
| Fig. 3
T
g variation (from 42 °C to 34 °C) as a function of humidity in the range between 1.4% and 70%. Reprinted with permission from reference Polym. Test., 2018, 65, 407–413.112 Copyright 2018 Elsevier. | |
Lately, Alves et al. have continued their investigation on thermomechanical analysis by performing temperature modulation on cantilever sensors.115 Specifically, temperature modulation refers to the superposition of two different heating rates at the same time, a constant heating rate (1 °C min−1, from 20 to 50 °C) and a variable, usually sinusoidal, heating rate (0.3 °C of amplitude and 0.025 Hz of frequency; 40 seconds period). By doing so, the thermal history contribution (non-reversing signal) and the effects arising from the heating rate (reversing signal) were decoupled. Indeed, such temperature modulation allowed for a more precise and faster Tg determination. Moreover, physical aging was studied quantitatively, measuring the average relaxation time on the peak of the non-reversing signal. Also, it was observed that the reversing sensitivity did not depend on the state of the initial deflection and presented minimum variation between aging times. Hence, the sigmoidal curve was used to extract the Tg without quenching or erasing the thermal history, which allowed a better comparison of glasses aged close to the glass transition temperature.
Overall, the main drawback of such recognition events when characterizing the properties of thin coatings relies on reproducibility ascribed to the non-homogeneous coating of the cantilever. Hence, even though the prospects for this application are positive and advances are being made to solve it, changes regarding the manufacturing techniques need to be considered to produce reliable coatings and, thus repetitive results.
3.2. Microcalorimeters
Heat variations of chemical, physical, and biological reactions are usually detected with conventional calorimeters, allowing a thermodynamical or analytical study of the reaction to be carried out. This approach, which has significant advantages, such as real-time monitoring and being non-destructive, has become an essential technique, especially in the biological field. However, to maximize the sensitivity of small volume samples, the calorimeter requires miniaturization.
Back in 1993, Gimzewski et al. presented a microcantilever-based calorimeter.116 During their experiments, the catalytic reaction H2 + O2 → H2O was studied by monitoring heat variations, thus becoming the first ones to develop a heat flow sensor using silicon microcantilever-based technology. Nowadays, the heating mode is widely used to monitor enzymatic reactions and characterize picoliter biological samples.117,118
Among other features, the tiny amount of a sample needed, fast response, and the reversibility of this type of sensor attracted a lot of interest. Since then, other investigators have presented studies taking advantage of the heat mode of bimetallic cantilevers. In 2001, Abedinov et al. reported the comparison of the bending of two different bimetallic cantilevers, SiO2/Si and SiO2/Al, under infrared (IR) radiation, with the latter showing higher sensitivity.119 With a similar system, Su and Dravid studied the photocatalytic oxidation of volatile hydrocarbons in order to monitor the reactions on a surface combustion microengine.120 To do so, a bimetallic (SiO2/TiO2) microcantilever was exposed to hydrocarbon vapors that were irradiated with ultraviolet light. More recently, Alodhayb et al. reviewed the application of nanomechanical calorimetric infrared spectroscopy using bi-material cantilevers for the molecular recognition in picoliter volumes of liquid samples.121
Cantilever-type calorimeters have been used for differential thermal analysis (DTA) and differential scanning calorimetry (DSC) to study metallic samples, such as indium, at the nanoscale. The sensor developed by Nakabeppu and Deno was able to perform fast temperature scans (over 1000 K s−1).122 Two types of Si/SiO2 cantilevers were developed for such nano-DTA and nano-DSC characterization. Other research studies have focused on the optimization of the coating thickness of biomaterial cantilevers to improve sensitivity from the theoretical point of view.123
Indeed, bimetallic based microcantilevers are, by far, the most used microcalorimeters in micro-electronic mechanical systems (MEMS) technology. Nevertheless, other alternatives for microcalorimetric characterization have been recently reported. For example, TiO2 nanowell-patterned microcantilevers owe their thermomechanical sensitivity to the different expansion coefficients and Young's modulus.124 This structure has proved to be a sensitive way to detect organophosphorus compounds by photothermal spectroscopy. Other research groups have also been working on developing a very sensitive photothermal spectroscopy technique.125,126
3.3. Environmental monitoring
3.3.1. Humidity sensors.
As mentioned above, in 1994, Allison and coworkers reported the variations in the resonance frequency of a bimetallic scanning force microscope cantilever produced by thermal oscillations and relative humidity variations.16 One year later, they developed a humidity sensor using gelatin or hygroscopic phosphoric acid as a sensing material.127
Nowadays, cantilever-based sensors with piezoresistive or capacitive readout systems are the most widely used for humidity sensing. Indeed, as reviewed by Xu et al.,128 piezoresistive cantilever devices coated with large-surface-area nanostructures of hygroscopic materials, such as metal oxides, ceramics, organics, or organic/inorganic composites, offer an ideal platform for highly sensitive humidity detection by displaying adequate adsorption/desorption characteristics and a large number of binding sites for the highly efficient adhesion of water molecules that, ultimately, alter the resonance frequency of the microcantilever and/or induce its deflection, as well. For instance, in the context of agricultural development, real-time humidity monitoring is essential because of water scarcity. For this reason, a significant part of the research related to humidity sensors is orientated towards this specific field.129
Metal oxide coatings are used in humidity sensor configurations because of their porosity. Ji et al. reported a limit of detection of 10 ppm of moisture in nitrogen within three minutes using a microcantilever modified with aluminum oxide (Al2O3).130 Indeed, Al2O3 is one of the most common ceramic materials used for humidity sensing on account of its small pore, thus enabling the detection of very small amounts of water molecules. In their work, an innovative way was reported to precisely control the coating of the cantilever, which consisted of a gold layer over a chromium layer on one side of the cantilever, while the other side was coated with Al2O3.
However, one of the main problems that ceramics display as sensing materials is the low reproducibility of the coating structure. Hence, overcoming this problem and controlling the coating pattern has become a huge matter of interest. Lee et al. achieved building in situ a well-structured hexagonal pattern of anodic aluminum oxide nanowells as moisture sensors by a complex multistep procedure that included electropolishing, anodization and wet-etching, thus achieving a sensitivity of 2.83 nm ppm−1.131 Other metal oxides, such as ZnO, have also been used as humidity sensing materials, but the problems that arise from the lack of morphological reproducibility and the thickness are similar to those displayed by Al2O3.132–134 For instance, sensors based on ZnO displayed the following reported sensitivity values: 101.5 ± 12.0 ppm/RH% (ZnO nanorods132); 4.4–16.9 ppm/% RH (ZnO nanorods133); and 21.4 Hz/% RH (ZnO nanoneedles with a defined morphology134).
Polymeric materials are also used for humidity sensing based on their high specificity, reversibility and short time response. Concretely, poly(3,4-ethylenedioxythiophene)/poly-styrene-sulfonic acid (PEDOT/PSS) was used as a humidity sensor material for its stability, high conductivity, and straightforward maunfacturing.135 During the fabrication process, the cantilever was functionalized by drop casting the polymer onto the silicon surface, but the heterogeneity of the polymeric thin film affected the reproducibility of the sensor. To overcome this problem, Sappat et al. developed an inkjet printing method.136 Nonetheless, other polymeric materials are also being studied and compared considering their efficiency as humidity sensing materials. For example, Steffens et al. tested the sensitivity to moisture of different polymers, such as polyaniline (26.4–100 nm/% RH), poly(o-ethoxyaniline) (24.1–74.2 nm/% RH), and polypyrrole (70.1–682.6 nm/% RH), the last one displaying the lowest limit of detection (170 ppbv), in addition to the best reproducibility.137
As mentioned earlier, some polymeric materials swell under humid conditions, and this swelling bends the cantilever. Such a phenomenon is the basis of a new kind of humidity sensor. The configuration of one of these sensors consists of two stationary electrodes on each side of a cantilever (Fig. 4).138 The cantilever is coated with a polymeric material (polyimide) that swells with longitudinal asymmetry. When the sensor is exposed to a humid environment, the polymer swells producing a lateral bending that is transduced to a change of capacitance.
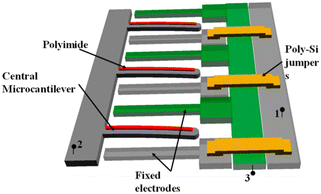 |
| Fig. 4 Array structure of parallel polymer-based laterally amplified chemo-mechanical (LACM) unit cells. Reprinted from ref. 138 (under a Creative Commons license (CC BY 4.0)). | |
A different approach uses graphene oxide (GO) as a sensing material.139–142 For instance, Le et al. operated the cantilever with high order frequency modes, thus obtaining a ten times better sensitivity than other humidity sensors (the sensitivity increased from 9.67 to 84.41 Hz/% RH).139 To reach those modes, an interdigital transducer fabricated on an aluminum nitride (AIN) layer was used to excite the piezoelectric layer. The cantilevers were uniformly coated with GO by vacuum filtration, and the humidity sensing capacity of the system relied on the surface to volume ratio and the hydrophilicity of GO. Overall, the sensor showed very good repeatability, fast response, and short recovery time (∼10 s). For comparison, in the other studies were GO was employed, the sensitivity values achieved were: 39.2 Hz·cm2 ng−1 at 10–90% RH,140 27.3 Hz/% RH,141 and 5 kHz/% RH in the range between 3% RH and 70% RH.142
Finally, Grogan et al. combined the working principle of electronic readouts with the readout system of holographic sensors to obtain a low-cost, but high-sensitive readout system, with a detection limit of ca. 1.2% RH.143 The hybrid sensor included a polymeric bilayer composed of polydimethylsiloxane, and a holographic patterned thin photopolymer as a diffractive element. In conclusion, relative humidity measurements showed that the sensitivity can be tuned by controlling the thickness of the polymeric layers and the spatial frequency.
3.3.2. Pressure sensors.
Microcantilever-based pressure sensors have become relevant because of their significant advantages, which include low power-consumption, easy batch fabrication, small size, and high sensitivity, among others. The effect of pressure on the resonance frequency of a cantilever has been studied experimentally and theoretically by several research groups with cantilevers that differ in the material, the shape, and the readout system, either for measuring absolute pressure,144,145 or differential pressure.146,147
For instance, over the past few years, Shimoyama and co-workers146–148 have developed a differential pressure sensor consisting of a cantilever placed on an open chamber. When the barometric pressure changes, a temporary difference between the inside and the outside of the chamber bends the cantilever towards the side with lower pressure. In later investigations, they proved an inversely proportional relation between the frequency response and the air leakage produced at the gap by the tip of the cantilever, by varying the size of the chamber and the gap.149
Capacitive pressure sensors are the most widely used pressure sensors, and their sensitivity has been improved over the past few years. Krakover et al., for example, developed a sensor consisting of a Si cantilever placed within a pressure-driven deformable membrane, where the deflection of the cantilever perturbed the electrostatic field generated by the side electrodes leading to a resonant frequency shift.150 However, to please the industry demand of cantilever-based pressure sensors, their stability under harsh conditions is yet a requirement to fulfill. To overcome this problem and provide better chemical and thermal stability, Fu et al. developed a single crystal diamond capacitive sensor by applying selective high-energy ion implantation, inductive coupled plasma (ICP) etching and electrochemical etching, and metal evaporation techniques.151 Besides, Subbiah et al. worked on the thermal stability of cantilever-based pressure sensors using different materials,152 which included a ceramic substrate, a glass solder, and high temperature resistant metals.
In a finale example, Shimoyama and coworkers developed a pulse sensor using a deformable 50 μm-thick polyimide membrane placed under the cantilever, all in a closed chamber.153 When the membrane was placed over a vessel, the pulse generated the deformation of the membrane, thus changing the pressure inside the chamber and, consequently, deflecting the cantilever.
3.4. Chemical detection
Following their investigation, Thundat et al. reported in 1995 the constant bending of a cantilever when it was exposed to mercury vapors,154 which indicated that small amounts of absorbate can be quantified by measuring the change in the resonant frequency of the cantilever (i.e. frequency shift). This work opened the door to a wide research area: detection of specific compounds by cantilever-based technologies (Fig. 5). Indeed, later in 2003, an optimized version of the system was reported where the cantilever was covered with a thin film of ZnO.155
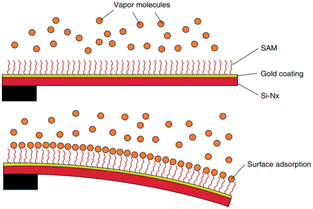 |
| Fig. 5 Schematic diagram of a cantilever bending due to differential adsorption of analyte molecules. Reprinted with permission from reference Elsevier, 2007, pp. 109–130.156 Copyright 2007 Elsevier. | |
3.4.1. Explosives.
Detection of explosive materials is a matter of interest regarding homeland security in airports, ports, and border controls, as well as being significantly relevant to military use, demining, and criminal investigations. Hence, cantilever sensors, which detect small amounts of molecules in a rapid fashion, have become a very suitable solution for this area. Indeed, during the past few years, research on high-specificity cantilever functionalization to produce more suitable materials for explosive detection and surface modification to enlarge the surface area to volume ratio has attracted a lot of interest.
Thundat et al. continued their investigation on explosive detection by functionalizing the surface of a gold coated silicon cantilever with a self-assembled monolayer of 4-mercaptobenzoic acid.157 This functionalization enabled the detection of pentaerythritol tetranitrate and hexahydro-1,3,5-triazine in concentrations as small as 10–30 ppm. Other research groups also functionalized cantilevers with self-assembled monolayers (SAMs)158,159 or with polymers, imprinting them directly onto the cantilever.160 The latter technique allowed for the specific orientation of the monomer, thus favoring the binding between the functionalized cantilever and the analyte.
Still, though, in this configuration, external parameters, such as temperature, can shield the response of the absorbed explosives. Similarly, the humidity from the environment or the presence of other molecules, such as acetonitrile, anisole, benzaldehyde or toluene, interferes with the sensor performance.161 In order to consider a realistic environment, Shemesh et al.162 functionalized silicon cantilevers with poly 3-aminopropyl silane (APS). The interaction between trinitrotoluene (trinitrotoluene) molecules and APS resulted in an irreversible nucleophilic reaction. On the contrary, the interaction with water and volatile organic compounds was reversible. By heating the cantilever after the absorption process, molecules that underwent a reversible reaction with APS were released, enabling the detection of 2,4,6-trinitrotoluene (TNT). Later on, focusing on the reversibility of the absorption step after detection to yield reusable explosive detectors, Thundat and coworkers monitored the resonance frequency shift induced by the absorption of explosive molecules, such as pentaerythritol tetranitrate (PETN) or cyclotrimethylene trinitramine (RDX), in addition to TNT.163
One step further in complexity, multisensing platforms for detecting several explosive materials simultaneously were designed following different functionalization pathways for each cantilever on an array.164–166 In this area, Palaparthy et al. presented an algorithm for multisensing combining SU-8 and silicon nitride coated cantilevers that operated in two different modes for explosive material detection: a rapid testing mode, less accurate but faster, and a comprehensive mode, which reached an accuracy of ∼80%.167 To improve selectivity, these sensors were coated with surface receptors (4-mercaptobenzoic acid (4-MBA), 6-methoxy naphthalene acetic acid (6-MNA), and 4-adenosine triphosphate (4-ATP)), and explosive compounds were detected in a controlled environment at concentration as low as 16 ppb of TNT, 56 ppb of RDX, or 134 ppb of PETN.
Rao and co-workers investigated alternative semi-conductive and piezoresistive materials to substitute traditional silicon cantilevers for explosive-detection purposes.168–173 Polymeric cantilevers that favor their functionalization with different SAMs to specifically detect explosive vapors were the most promising candidates.
The sensitivity of cantilevers towards explosive materials improved by texturizing the surface to increase the surface to volume ratio (Fig. 6).165 Hence, a larger active surface enables the absorption of a greater amount of analyte, lowering the detection limit. In the search for the best material for such superficial modification, TiO2 nanotubes appeared as a suitable candidate to build patterned microstructures onto a cantilever beam, enhancing the explosive detection because of their selectivity towards NO2 that is present in many explosive compounds.174–176 For instance, Keller and co-workers developed a silicon cantilever with a vertically aligned TiO2 nanotube network prepared by anodization, which allowed the detection of concentrations of TNT as low as 0.8 ppb.176 Cu(OH)2 and CuO nanorods were also used to texturize the surface of the cantilever, allowing the detection of NO2 with high sensitivity.177 The significance of the surface area was also considered when texturizing a cantilever array with Co-BEA, a type of zeolite, for the sensing of 2-nitrotoluene.178 With this material, a multisensing platform of 4 cantilevers with an integrated electric readout interface was developed.
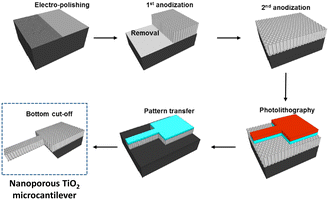 |
| Fig. 6 Schematic illustration of the fabrication process of a nanoporous TiO2 microcantilever. Reprinted (adapted) with permission from Anal. Chem., 2014, 86(10), 5077–5082.165 Copyright 2014 American Chemical Society. | |
Finally, Wang and co-workers used carbon nanotubes (CNT) in combination with a coupled heating system to induce deflagration of the explosive absorbed molecules, which enhanced the bending of the cantilever because of the volume expansion produced by the heat released from the deflagrations (Fig. 7).179,180 The working principle of this sensor was further investigated for detecting RDX and PETN.181 In a similar way, the simultaneous detection of TNT, RDX and 2,4-dinitrotoluene (DNT) in the range of ppb was achieved.182 As it can be seen, this field of study is attracting a lot of attention, possibly because of the relevance and importance of the outcome in terms of security. Nevertheless, although interesting possibilities have been proposed so that such systems are translated to our everyday items and activities (for instance, adapted devices can be used in airports or crowded places to detect explosives and, thus, prevent large accidents), feasible translation has yet to be achieved.
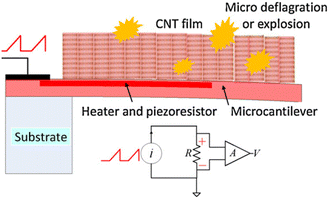 |
| Fig. 7 Schematic illustration of the sensor configuration and the operation principle vapors adsorbed on the CNT sensor after heating to micro deflagration. Reprinted with permission from ref. 179. Copyright 2013 Elsevier. | |
3.4.2. Pesticides.
Pesticides, including herbicides and insecticides, are being used worldwide before and after harvesting to prevent plagues, pests, and diseases, and ensure successful production and safe food consumption. Global agricultural productivity has been growing in accordance to population growth. Hence, such intensification has become, in turn, a source of contamination for crops, air, soil, and water because of underground leaching.183 Although chromatography is the most widespread pesticide detection method, its complexity, cost, and time consumption motivated the investigation of sensitive, fast and low-cost alternatives. Nanomechanical sensors quickly became a leading alternative to chromatographic analysis.
Many pesticides are organophosphate compounds with high insecticide activity. Although these compounds show low persistence, traces of organophosphates can be harmful to humans. Among this group, glyphosate (i.e. N-(phosphomethyl) glycine) is the most used organophosphate herbicide for weed control. Steffens and co-workers developed a glyphosate cantilever sensor with peroxidase enzyme extracted from zucchini.184 The enzyme was covalently bonded to the gold coated cantilever through a carbodiimide reaction. The conformation of the enzyme changes upon the adsorption of the glyphosate, inducing a negative bending of the cantilever that is transduced into an electric readout signal. This system worked also for detecting atrazine, the second most used herbicide, an environmental contaminant and a xenobiotic.185 In this case, although the atrazine detector was developed by the same approach, the cantilevers were functionalized with tyrosinase, extracted from bananas. Similarly, synthetic hapten conjugated with bovine serum albumin (BSA) was covalently immobilized onto a cantilever to detect dichlorodiphenyltrichloroethane (DDT).186
Interestingly, organophosphate compounds undergo a condensation reaction in the presence of carboxylic acids. Based on that, Xia et al. functionalized the inner channels of mesoporous silica nanoparticles with carboxylate groups and loaded them onto a silicon cantilever. By applying the dynamic mode, the resulting cantilever detected 200 ppb of acephate, an organophosphate foliar and soil insecticide.187
Karnati et al. reported a cantilever sensor for monitoring the presence glyphosate or chlorpyrifos exploiting their specificity towards organophosphorus hydrolase (OPH, also known as phosphotriesterase),188 which is a bacterial enzyme that is capable of degrading a wide range of neurotoxic organophosphate agents.189 A gold coated V-shaped microcantilever was functionalized with OPH reaching a limit of detection of 10−7 M for a solution of paraoxon, a synthetic aryl dialkyl phosphate compound that is the active metabolite of the insecticide parathion. Zourob et al. developed a pesticide detector by functionalizing a cantilever by dispersing OPH into a pH-responsive hydrogel matrix.190 The interaction between OPH and specific pesticides, paraoxon and parathion, led to a pH decrease, a change in the elasticity, as well as in the volume of the hydrogel, which bended the cantilever.
As Keller and co-workers did for enhancing the detection sensitivity of TNT detection,176 Biapo et al. investigated the controlled arrangement of TiO2 nanotubes on a wafer to later build them on a cantilever.191 The large surface area of the nanostructured cantilever, in addition to the specific functionalization with oxime, caused a significant frequency shift of the resonance frequency of the cantilever when working under the dynamic mode, enabling the detection of dimethyl methylphosphonate (DMMP). Overall, a lot of research is being undertaken to detect organochloride and organophosphorus pesticides and herbicides at the ng L−1 scale in aqueous media. Indeed, we believe that the use of microcantilever sensors in such a field requires precise and highly sensitive devices; moreover, if most of these detection events are going to happen on site, robustness, cost and versatility are also important requirements for portable systems.
3.4.3. CO2 and CO.
The excess of carbon dioxide (CO2) in the atmosphere, which is formed during combustion, fermentation, or respiration, is responsible for the greenhouse effect, while dizziness, headaches, unconsciousness and oxygen deprivation happen at high concentration values. Besides, any uncompleted combustion generates carbon monoxide (CO), which competes with oxygen for the binding sites of hemoglobin, and thus causes dizziness, unconsciousness and, ultimately, death if the exposure is prolonged. Therefore, the detection and monitoring of these gases becomes essential in specific healthcare, engineering, and environmental applications.
One strategy to revert the greenhouse effect relies on developing carbon capture storage (CCS) devices. Within this context, amine-functionalized nanoporous silica is one of the most outstanding materials due to: i) the large surface area of silica; and ii) the specificity of the amine functional group towards CO2. To perform a fast and low-cost deep investigation of several amine-functionalized nanoporous silica systems, Lee et al. deposited the functionalized nanoporous silica on top of the free end of a cantilever beam.192 The authors examined the absorption and desorption process of CO2, analyzing the resonance frequencies of each cantilever beam (Fig. 8).
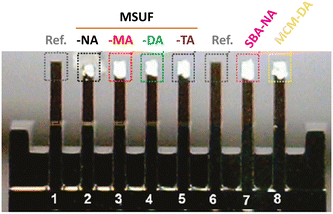 |
| Fig. 8 Optical microscopy image of the sorbent-coated microcantilever array. Reprinted (adapted) with permission from reference Environ. Sci. Technol., 2011, 45(13), 5704–5709.192 Copyright 2011 American Chemical Society. | |
In the same line of action, a green energy alternative to traditional fossil fuels focuses on designing fuel cells, which convert the chemical energy of hydrogen or other fuels to cleanly and efficiently produce electricity. Caliskan et al. have recently been testing the monitoring of the gas mixture composition with a microcantilever sensor, instead of the more usual spectral analyzers, to detect minute concentrations of CO that, if present, can cause fuel cell poisoning and significantly reduce the life expectancy of the cell.193 Similar to the proposal of García-Romeo et al. for the detection of TNT,178 the CO sensing was based on the frequency shift produced by the absorption of CO onto cantilevers coated with copper Y (CuY) zeolite. In this study, the sensor was optimized in terms of coating thickness and temperature. Other groups also used a zeolite-based functionalization for the detection of ethanol and water.194
Polymeric microcantilevers based on a bisphenol A novolac epoxy (SU-8) were modified to detect CO by coating them with Fe(III)porphyrin.195 Overall, this sensor configuration was very sensitive to CO, as well as selective, when compared to other gases, such as N2, CO2, O2, ethanolamine, N2O, and moisture. In this area of research, microcantilever technology is expected to contribute greatly although there is still room for improvement. Specifically, tailored functionalization strategies need to be applied to improve sensitivity, as well as selectivity, and contribution from other research fields, such as organic and inorganic chemistry, or catalysis, for instance, need to be considered.
Finally, CO2 detection is also used for biomedical applications, where the CO2 produced from patients under anesthesia is monitored to control their well-being. From a theoretical point of view, computational simulations were used to optimize the parameters of microcantilever-based sensors. Ahmad et al. did so by utilizing one of the most stable sensing materials, ZnO.196
3.4.4. VOC detection.
The emissions of some volatile organic compounds (VOCs) that are harmful to humans or detrimental to the environment need to be controlled. Therefore, the in situ detection of these kind of compounds is a matter of interest. Similar to explosive material sensors, VOC detection requires highly sensitive portable devices, thus making microcantilever sensors an excellent choice.
In 2001, Kim et al. reported the detection of n-octane and toluene using a polydimethylsiloxane (PDMS) and polyetherurethane (PEUT) functionalized microcantilever.197 Later on, a closed feed-back system driven by a magnetic and an electrostatic actuator was developed to improve the detection sensitivity.198 That work also expanded the analytes and polymers under consideration, which included 1-butanol as an analyte, while poly(cyanopropylmethyldimethylsiloxane) (CYP), phenyl-vinyl-polydimethylsiloxane (SE54), poly(phenylmethyldimethylsiloxane) (MTP-OV-1701), and polyethyleneglycol (CARBOWAX) were applied to functionalize the cantilevers. Such polymers were chosen because of their different chemical behavior and polarity. The proposed detection mechanism was based on the swelling of the polymer after the absorption of VOC molecules, which generated surface stress on the cantilever, thus inducing its bending, while the increment of mass caused a change in the frequency shift, as well. Similarly, Toda et al. embedded a Si cantilever in a methyl methacrylate acrylic resin to detect water, ethanol, and acetone.199
Other microcantilever-based sensors for VOC detection have been designed controlling features at the nanoscale, as in the case of 1D ZnO nanostructures.200 Recently, VOCs in solution have been detected with an improved gas sensor that contained a sensor chamber divided into two sections (Fig. 9). The solvent stays aside from the cantilever by placing a waterproof and breathable expanded polytetrafluoroethylene (ePTFE) film,201 and VOC molecules reach the cantilever without interference of the solvent.
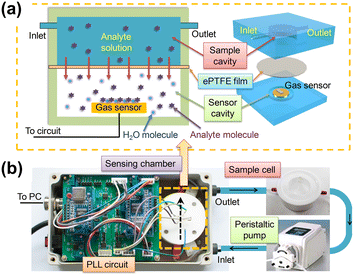 |
| Fig. 9 Schematic of the sensing platform. (a) Cross sectional (left) and 3D (right) schematic view of the sensing chamber of the platform. (b) Photos of the main components of the sensing platform. Arrows show the fluid flow direction. Reprinted with permission from reference Talanta, 2018, 182, 148–155.201 Copyright 2018 Elsevier. | |
Even though several polymeric coatings have rendered promising sensor systems, their reproducibility is not accurate enough, mainly because of the low homogeneity of the polymeric thin film. Indeed, the preparation of the cantilever platform is a delicate one that needs to be carefully performed to avoid defects. As mentioned earlier, the fast development of manufacturing techniques might solve this issue by yielding reliable coating techniques.
3.4.5. Other analytes.
Hydrogen sulfide is a corrosive, toxic, and inflammable gas mainly produced in the petroleum and natural gas industry, as well as in sewage. One of the main problems of traditional H2S sensors is their low selectivity. Therefore, most recent studies have focused their attention on increasing the level of selectivity towards H2S.202 For example, Tang et al. developed a double sensor that is composed of two cantilevers, where one is built to sense H2S, while the other only detects it.203 The sensing cantilever was coated with a zeolitic imidazolate framework (ZIF-8) and nitrogen-doped nanoporous carbon (NPC), resulting in an extremely sensitive system. However, it was not very selective, so the second cantilever solved this weakness. The selective cantilever was covered with basic copper carbonate (BCC) which reacted with H2S to produce CuS. This reaction resulted in a loss of mass of the cantilever which causes a positive frequency shift. In such a manner, a selective H2S sensor was produced with a limit of detection lower than 1 ppb.
Finally, some metals are carcinogenic for human beings in both particle and ion form. Beryllium, in particular, is known to generate chronic beryllium disease (CBD). Peng et al. developed a functionalization process towards a sensitive beryllium sensor.204 The cantilever was covered with two layers of chromium and gold, and benzo-9-crown-3 functionalized polymer brushes were built on top by radical polymerization. The large surface-area ratio and the electrostatic repulsive forces made the polymer brushes a very interesting choice to develop highly sensitive microcantilever sensors. In fact, those materials are widely used for the specific functionalization of cantilevers, allowing for the detection of small changes in potential when a bias voltage is applied, as well as several types of analytes separately, such as potassium or glucose.205–207 Indeed, further research on selective cantilever platforms can widen the scope of detection and enlarge the list of other analytes to be measured.
3.5. Other applications
In addition to all the fields that have been reviewed, it is worth mentioning a couple of less exploited applications. Nordström et al. have been working on an integrated optical readout system based on single-mode waveguides for improving the sensitivity of microcantilever sensors. In that system, the cantilever bending was measured by monitoring changes in the optical intensity of light transmitted through the cantilever that also acted as a waveguide.208
Besides, the mechanical properties of hydrogels, polymer brushes, and ionizable surfaces have been exploited to detect changes in pH. For example, aluminum oxide (Al2O3) cantilevers have been reported to detect electrolyte concentration, with the bending of the cantilever being triggered upon the ionization of the cantilever surface as a function of pH.209–210
Moreover, magnetic susceptibilities have been measured using nanogram amounts of magnetic materials. Finot et al. developed a miniature magnetic force balance based upon the simultaneous measurement of the cantilever change regarding bending and resonance frequency. In this set-up, the cantilever acted as a Faraday balance.211
Finally, we address the reader to the use of microcantilever-based devices in the field of microfluidics. Back in 2010, Ricciardi et al. focused on the integration of the microcantilever with microfluidic technology to perform real-time detection of specific proteins (angiopoietin-1, Ang-1, a putative marker in tumor progression) in liquids.212 In addition to optimizing the sensor geometry (aspect ratio of microplates) and the choice of materials for the microfluidic platform (pirex), Ang-1 was successfully detected and antibody–antigen real-time kinetics were observed. Also, a bi-material U-shaped microchannel cantilever (16 μm wide, 1050 μm long and 3 μm high) was developed on top of a plain cantilever to perform both the physical and chemical analysis of 50 pL volume samples of liquid reagents.213 The system, which was turned into a bimaterial beam by depositing a 500 nm thick layer of aluminum on its bottom side using e-beam evaporation, was conceived to be potentially used in micro-bioreactors.
Similarly, Etayash et al. also designed a bimaterial microcantilever with an embedded microfluidic channel to selectively capture bacteria passing through the channel.214 The working principle relied on the fact that bacterial adsorption on the cantilever changed both the resonance frequency (added mass) and cantilever deflection (adsorption stress). Specifically, the device was fabricated using silicon nitride with a 300 nm-thick layer of gold on one side for enhanced thermal sensitivity and an optical-beam-deflection method was added. The lowest detection limit was established at 100 cells per 100 μl (a single cell per μl), for a signal-to-noise ratio of 3.
More recent studies have also exploited the benefits of the cantilever technology to produce non-invasive, low-cost, and easy-to-fabricate tools for characterizing the flow rate in paper microfluidic systems, for instance,215 or examining the thermal characteristics of liquid analytes using the photothermal heating effect.216 Also, simulations from computational models are being validated with experimental data to predict the change in the resonance frequency as a function of the change in mass for hollow resonant-based microfluidic microcantilever systems.217 The event determined was the measured evaporation rate of ethanol at the picoliter scale.
4. Outlook and perspectives
During the past few years, nanomechanical sensors have been presented as essential devices for the characterization, monitoring, and sensing of specific compounds. Even though advances in the microfabrication process, materials, geometries, and readout systems have allowed the expansion of this technology to several fields, thus covering a large number of very specific applications, still some challenges should be overcome for an impactful translation of this technology into commercial and clinical applications. In that regard, we carefully address some of those next.
Countless types of functionalization strategies have already been reported to improve sensitivity, as well as selectivity, for example, volatile compound detection by polymeric coatings. However, the reproducibility of these sensors is not accurate enough because of the low homogeneity of the polymeric thin film. Coating the cantilever is a very delicate, and not an easy process. Thus, we envisage further research on the development of reliable coating techniques.
Although a great number of applications have been reviewed under different environments, that is vacuum, gas, or liquid, the performance of the cantilever in the latter is still not accurate. The damping of the resonation of the microcantilever causes a significant effect on the sensitivity. Although there are already some optimized sensors that allow sensing in liquid with good sensitivity, there is still a meaningful gap in terms of performance between microcantilever sensors working in liquid or under vacuum. In the upcoming years, we expect an increase of innovative solutions and contributions to solve this drawback.
The readout system plays a key role when optimizing microcantilever-based sensors. To date, optical readout systems are the most common choice because of their high resolution, simplicity, high sensitivity, and versatility. On the other hand, the tedious calibration associated with this readout system is a major disadvantage. Although other readout methods, such as interferometry-based readout, capacitive readout, or piezoresistive cantilevers, are also used on microcantilever sensors, they do not yet fulfill all the requirements. For instance, capacitive methods are not suitable for solutions, while interferometry-based readout systems are not sensitive enough. In order to normalize the use of microcantilever sensors in any field, such as point-of-care applications, a robust, cheap, portable, highly sensitive, and versatile readout system needs to be developed.
5. Conclusions
In this Review, we have focused our attention on the application of microcantilever technology in various fields to characterize materials, as well as conduct environmental monitoring and chemical and physical sensing. Such areas of study have attracted a lot of interest during the past decade based on the potential of commercial translation, with numerous studies being reported. Indeed, the resulting sensors display promising advantages, such as high sensitivity, fast time-response, low-cost, portable size, small sample volume required, and low power consumption, in addition to reliability. However, much remains to be done – we consider essential to continue researching these advanced sensing materials to attain improved selectivity and further decrease the limit of detection. Besides, the future envisaged applications, more complex in nature, would require highly interdisciplinary science, with contributions from physics, mathematics, biology, chemistry, and engineering. In that line, complementary computational studies and theoretical simulations, although challenging, can guide in the design of the next generation of microcantilever sensing devices.
Conflicts of interest
There are no conflicts to declare.
Acknowledgements
This publication is part of the I+D+i project PID2021-125767OB-I00 funded by MCIN/AEI/10.13039/501100011033 and, as appropriate, by “ERDF A way of making Europe”, by the European Union. H. M.-G. thanks the Generalitat de Catalunya for a FI-SDUR 2020 Fellowship. M. M. P.-M. thanks the Spanish Ministry of Educación y Formación Profesional for the Junior Beatriz Galindo Award (BG20/00216).
References
- J. R. Barnes, R. J. Stephenson, M. E. Welland, C. Gerber and J. K. Gimzewski, Photothermal Spectroscopy with Femtojoule Sensitivity Using a Micromechanical Device, Nature, 1994, 372(6501), 79–81, DOI:10.1038/372079a0.
- A. K. Basu, A. Basu and S. Bhattacharya, Micro/Nano Fabricated Cantilever Based Biosensor Platform: A Review and Recent Progress, Enzyme Microb. Technol., 2020, 139, 109558, DOI:10.1016/j.enzmictec.2020.109558.
- B. O. Alunda and Y. J. Lee, Review: Cantilever-Based Sensors for High Speed Atomic Force Microscopy, Sensors, 2020, 20(17), 1–39, DOI:10.3390/s20174784.
- M. Calleja, P. M. Kosaka, Á. San Paulo and J. Tamayo, Challenges for Nanomechanical Sensors in Biological Detection, Nanoscale, 2012, 4(16), 4925–4938, 10.1039/c2nr31102j.
- K. R. Buchapudi, X. Huang, X. Yang, H.-F. Ji and T. Thundat, Microcantilever Biosensors for Chemicals and Bioorganisms, Analyst, 2011, 136(8), 1539–1556, 10.1039/C0AN01007C.
- S. Xu and R. Mutharasan, Cantilever Biosensors in Drug Discovery, Expert Opin. Drug Discovery, 2009, 4(12), 1237–1251, DOI:10.1517/17460440903386643.
- J. L. Arlett, E. B. Myers and M. L. Roukes, Comparative Advantages of Mechanical Biosensors, Nat. Nanotechnol., 2011, 6(4), 203–215, DOI:10.1038/nnano.2011.44.
- C. Ziegler, Cantilever-Based Biosensors, Anal. Bioanal. Chem., 2004, 379(7–8), 946–959, DOI:10.1007/s00216-004-2694-y.
- A. Boisen, S. Dohn, S. S. Keller, S. Schmid and M. Tenje, Cantilever-like Micromechanical Sensors, Rep. Prog. Phys., 2011, 74(3), 36101, DOI:10.1088/0034-4885/74/3/036101.
- K. Eom, H. S. Park, D. S. Yoon and T. Kwon, Nanomechanical Resonators and Their Applications in Biological/Chemical Detection: Nanomechanics Principles, Phys. Rep., 2011, 503(4), 115–163, DOI:10.1016/j.physrep.2011.03.002.
- G. Binnig, C. F. Quate and C. Gerber, Atomic Force Microscope, Phys. Rev. Lett., 1986, 56(9), 930–933, DOI:10.1103/PhysRevLett.56.930.
- C. Stroh, H. Wang, R. Bash, B. Ashcroft, J. Nelson, H. Gruber, D. Lohr, S. M. Lindsay and P. Hinterdorfer, Single-Molecule Recognition Imaging Microscopy, Proc. Natl. Acad. Sci. U. S. A., 2004, 101(34), 12503–12507, DOI:10.1073/pnas.0403538101.
- Y. J. Wang, P. Rico-Lastres, A. Lezamiz, M. Mora, C. Solsona, G. Stirnemann and S. Garcia-Manyes, DNA Binding Induces a Nanomechanical Switch in the RRM1 Domain of TDP-43, J. Phys. Chem. Lett., 2018, 9(14), 3800–3807, DOI:10.1021/acs.jpclett.8b01494.
- P. Nguyen-Tri, P. Ghassemi, P. Carriere, S. Nanda, A. A. Assadi and D. D. Nguyen, Recent Applications of Advanced Atomic Force Microscopy in Polymer Science: A Review, Polymers, 2020, 12(15), 1142, DOI:10.3390/polym12051142.
- M. Emmrich, F. Huber, F. Pielmeier, J. Welker, T. Hofmann, M. Schneiderbauer, D. Meuer, S. Polesya, S. Mankovsky, D. Ködderitzsch, H. Ebert and F. J. Giessibl, Subatomic Resolution Force Microscopy Reveals Internal Structure and Adsorption Sites of Small Iron Clusters, Science, 2015, 348(6232), 308 LP–311, DOI:10.1126/science.aaa5329.
- T. Thundat, R. J. Warmack, G. Y. Chen and D. P. Allison, Thermal and Ambient-Induced Deflections of Scanning Force Microscope Cantilevers, Appl. Phys. Lett., 1994, 64(21), 2894–2896, DOI:10.1063/1.111407.
- M. Krieg, G. Fläschner, D. Alsteens, B. M. Gaub, W. H. Roos, G. J. L. Wuite, H. E. Gaub, C. Gerber, Y. F. Dufrêne and D. J. Müller, Atomic Force Microscopy-Based Mechanobiology, Nat. Rev. Phys., 2019, 1(1), 41–57, DOI:10.1038/s42254-018-0001-7.
- A. Gupta, D. Akin and R. Bashir, Single Virus Particle Mass Detection Using Microresonators with Nanoscale Thickness, Appl. Phys. Lett., 2004, 84(11), 1976–1978, DOI:10.1063/1.1667011.
- J. Wang, L. Wang, Y. Zhu, J. Zhang, J. Liao, S. Wang, J. Yang and F. Yang, A High Accuracy Cantilever Array Sensor for Early Liver Cancer Diagnosis, Biomed. Microdevices, 2016, 18(6), 1–10, DOI:10.1007/s10544-016-0132-5.
- J. Wang, Y. Zhu, X. Wang, S. Wang, J. Yang and F. Yang, A High-Throughput Cantilever Array Sensor for Multiple Liver Cancer Biomarkers Detection, IEEE Sens. J., 2016, 16(12), 4675–4682, DOI:10.1109/JSEN.2016.2524515.
- B. Ilic, Y. Yang and H. G. Craighead, Virus Detection Using Nanoelectromechanical Devices, Appl. Phys. Lett., 2004, 85(13), 2604–2606, DOI:10.1063/1.1794378.
- E. Timurdogan, B. E. Alaca, I. H. Kavakli and H. Urey, MEMS Biosensor for Detection of Hepatitis A and C Viruses in Serum, Biosens. Bioelectron., 2011, 28(1), 189–194, DOI:10.1016/j.bios.2011.07.014.
- K. M. Hansen, H. F. Ji, G. Wu, R. Datar, R. Cote, A. Majumdar and T. Thundat, Cantilever-Based Optical Deflection Assay for Discrimination of DNA Single-Nucleotide Mismatches, Anal. Chem., 2001, 73(7), 1567–1571, DOI:10.1021/ac0012748.
- J. W. Ndieyira, M. Watari, A. D. Barrera, D. Zhou, M. Vögtli, M. Batchelor, M. A. Cooper, T. Strunz, M. A. Horton, C. Abell, T. Rayment, G. Aeppli and R. A. McKendry, Nanomechanical Detection of Antibiotic-Mucopeptide Binding in a Model for Superbug Drug Resistance, Nat. Nanotechnol., 2008, 3(11), 691–696, DOI:10.1038/nnano.2008.275.
- S. Shameem and P. S. S. Babu, Detection of Colorectal Carcinoma Cell Using Cantilever Based Mems Biosensor, Proc. - Int. Conf. Trends Electron. Informatics, ICEI 2017, 2018, 2018-Janua, 1005–1009, DOI:10.1109/ICOEI.2017.8300859.
- N. Inomata, M. Toda, M. Sato, A. Ishijima and T. Ono, Pico Calorimeter for Detection of Heat Produced in an Individual Brown Fat Cell, Appl. Phys. Lett., 2012, 100(15), 154104, DOI:10.1063/1.3701720.
- K. Gruber, T. Horlacher, R. Castelli, A. Mader, P. H. Seeberger and B. A. Hermann, Cantilever Array Sensors Detect Specific Carbohydrate−Protein Interactions with Picomolar Sensitivity, ACS Nano, 2011, 5(5), 3670–3678, DOI:10.1021/nn103626q.
- H. P. Lang, M. Hegner and C. Gerber, Nanomechanics - The Link to Biology and Chemistry, Chimia, 2002, 56(10), 515–519, DOI:10.2533/000942902777680216.
- R. Berger, C. Gerber, H. P. Lang and J. K. Gimzewski, Micromechanics: A Toolbox for Femtoscale Science: “Towards a Laboratory on a Tip.”, Microelectron. Eng., 1997, 35, 373–379, DOI:10.1016/S0167-9317(96)00201-8.
- J. Thaysen, A. D. Yalçinkaya, P. Vettiger and A. Menon, Polymer-Based Stress Sensor with Integrated Readout, J. Phys. D. Appl. Phys., 2002, 35(21), 2698–2703, DOI:10.1088/0022-3727/35/21/302.
- D. Grochla, L. Banko, J. Pfetzing-Micklich, H. Behm, R. Dahlmann and A. Ludwig, Si Micro-Cantilever Sensor Chips for Space-Resolved Stress Measurements in Physical and Plasma-Enhanced Chemical Vapour Deposition, Sensors Actuators A, 2018, 270, 271–277, DOI:10.1016/j.sna.2017.12.050.
- H. P. Lang, M. Hegner and C. Gerber, Cantilever Array Sensors, Mater. Today, 2005, 8(4), 30–36, DOI:10.1016/S1369-7021(05)00792-3.
- M. Godin, V. Tabard-Cossa, Y. Miyahara, T. Monga, P. J. Williams, L. Y. Beaulieu, B. Lennox and P. Grutter, Cantilever-Based Sensing: The Origin of Surface Stress and Optimization Strategies, Nanotechnology, 2010, 21, 075501, DOI:10.1088/0957-4484/21/7/075501.
- J. Fritz, M. K. Baller, H. P. Lang, H. Rothuizen, P. Vettiger, E. Meyer, H.-J. Güntherodt, C. Gerber and J. K. Gimzewski, Translating Biomolecular Recognition into Nanomechanics, Science, 2000, 288(5464), 316–318, DOI:10.1126/science.288.5464.316.
- R. Berger, E. Delamarche, H. P. Lang, C. Gerber, J. K. Gimzewski, E. Meyer and H.-J. Güntherodt, Surface Stress in the Self-Assembly of Alkanethiols on Gold, Science, 1997, 276(5321), 2021–2024, DOI:10.1126/science.276.5321.2021.
- J. Fritz, M. K. Baller, H. P. Lang, T. Strunz, E. Meyer, H.-J. Güntherodt, E. Delamarche, C. Gerber and J. K. Gimzewski, Stress at the Solid−Liquid Interface of Self-Assembled Monolayers on Gold Investigated with a Nanomechanical Sensor, Langmuir, 2000, 16(25), 9694–9696, DOI:10.1021/la000975x.
- D. Kramer, R. Nadar Viswanath and J. Weissmüller, Surface-Stress Induced Macroscopic Bending of Nanoporous Gold Cantilevers, Nano Lett., 2004, 4(5), 793–796, DOI:10.1021/nl049927d.
- H. Ibach, C. E. Bach, M. Giesen and A. Grossmann, Potential-Induced Stress in the Solid-Liquid Interface: Au(111) and Au(100) in an HC10 4 Electrolyte, Surf. Sci., 1997, 375, 107–119 CrossRef CAS.
- J. A. Sidles, J. L. Garbini, K. J. Bruland, D. Rugar, O. Züger, S. Hoen and C. S. Yannoni, Magnetic Resonance Force Microscopy, Rev. Mod. Phys., 1995, 67(1), 249–265, DOI:10.1103/RevModPhys.67.249.
- Y. Tang, J. Fang, X. Xu, H.-F. Ji, G. M. Brown and T. Thundat, Detection of Femtomolar Concentrations of HF Using an SiO2 Microcantilever, Anal. Chem., 2004, 76(9), 2478–2481, DOI:10.1021/ac035140g.
-
J. X. J. Zhang and K. Hoshino, Mechanical Transducers: Cantilevers, Acoustic Wave Sensors, and Thermal Sensors, in Micro and Nano Technologies, ed. J. X. J. Zhang and K. Hoshino, Academic Press, 2nd edn, 2019, ch. 6, pp. 311–412, DOI:10.1016/B978-0-12-814862-4.00006-5.
- T. Thundat, P. I. Oden and R. J. Warmack, Microcantilever Sensors, Microscale Thermophys. Eng., 1997, 1(3), 185–199, DOI:10.1080/108939597200214.
- D. Ramos, J. Mertens, M. Calleja and J. Tamayo, Study of the Origin of Bending Induced by Bimetallic Effect on Microcantilever, Sensors, 2007, 7(9), 1757–1765, DOI:10.3390/s7091757.
- W.-H. Chu, M. Mehregany and R. L. Mullen, Analysis of Tip Deflection and Force of a Bimetallic Cantilever Microactuator, J. Micromech. Microeng., 1993, 3(1), 4–7, DOI:10.1088/0960-1317/3/1/002.
- J. R. Barnes, R. J. Stephenson, M. E. Welland, C. Gerber and J. K. Gimzewski, Photothermal Spectroscopy with Femtojoule Sensitivity Using a Micromechanical Device, Nature, 1994, 372(6501), 79–81, DOI:10.1038/372079a0.
- R. Saeidpourazar and N. Jalili, Towards Microcantilever-Based Force Sensing and Manipulation: Modeling, Control Development and Implementation, Int. J. Robot. Res., 2009, 28(4), 464–483, DOI:10.1177/0278364908097584.
- J. Yi, W. Shih and W.-H. Shih, Effect of Length, Width, and Mode on the Mass Detection Sensitivity of Piezoelectric Unimorph Cantilevers, J. Appl. Phys., 2002, 91(3), 1680–1686, DOI:10.1063/1.1427403.
- J. Mouro, R. Pinto, P. Paoletti and B. Tiribilli, Microcantilever: Dynamical Response for Mass Sensing and Fluid Characterization, Sensors, 2021, 1–35, DOI:10.3390/s21010115.
- A. W. McFarland, M. A. Poggi, M. J. Doyle, L. A. Bottomley and J. S. Colton, Influence of Surface Stress on the Resonance Behavior of Microcantilevers, Appl. Phys. Lett., 2005, 87 (5), DOI:10.1063/1.2006212.
- X. C. Zhang, B. S. Xu, H. D. Wang, Y. X. Wu and Y. Jiang, Underlying Mechanisms of the Stress Generation in Surface Coatings, Surf. Coat. Technol., 2007, 201(15), 6715–6718, DOI:10.1016/j.surfcoat.2006.09.032.
- K. Dahmen, S. Lehwald and H. Ibach, Bending of Crystalline Plates under the Influence of Surface Stress-a Finite Element Analysis, Surf. Sci., 2000, 446(1–2), 161–173, DOI:10.1016/S0039-6028(99)01174-7.
- Y. Zhang and Y. P. Zhao, Applicability Range of Stoney's Formula and Modified Formulas for a Film/Substrate Bilayer, J. Appl. Phys., 2006, 99(5), 053513, DOI:10.1063/1.2178400.
- J. Tamayo, J. J. Ruz, V. Pini, P. Kosaka and M. Calleja, Quantification of the Surface Stress in Microcantilever Biosensors: Revisiting Stoneys Equation, Nanotechnology, 2012, 23(47), 475702, DOI:10.1088/0957-4484/23/47/475702.
- D. W. Dareing, T. Thundat, S. Jeon and M. Nicholson, Modal Analysis of Microcantilever Sensors with Environmental Damping, J. Appl. Phys., 2005, 97(8), 084902, DOI:10.1063/1.1880472.
- E. Finot, A. Passian and T. Thundat, Measurement of Mechanical Properties of Cantilever Shaped Materials, Sensors, 2008, 8(5), 3497–3541, DOI:10.3390/s8053497.
- N. Gao, D. Zhao, R. Jia and D. Liu, Microcantilever Actuation by Laser Induced Photoacoustic Waves, Sci. Rep., 2016, 6, 1–7, DOI:10.1038/srep19935.
- C. Zhang, W. Jiang, A. Ghosh, G. Wang, F. Wu and H. Zhang, Miniaturized Langasite MEMS Micro-Cantilever Beam Structured Resonator for High Temperature Gas Sensing, Smart Mater. Struct., 2020, 29(5), 055002, DOI:10.1088/1361-665X/ab78b6.
- M. K. Dounkal, R. K. Bhan and N. Kumar, Effects of Various Loading on the Performance of MEMS Cantilever Beam for In-Field Tuning of Sensors and Actuators for High Temperature and Harsh Environment Applications, Microsyst. Technol., 2020, 26(2), 377–394, DOI:10.1007/s00542-019-04551-8.
- H. C. McCaig, E. Myers, N. S. Lewis and M. L. Roukes, Vapor Sensing Characteristics of Nanoelectromechanical Chemical Sensors Functionalized Using Surface-Initiated Polymerization, Nano Lett., 2014, 14(7), 3728–3732, DOI:10.1021/nl500475b.
- N. I. Abu-Lail, M. Kaholek, B. LaMattina, R. L. Clark and S. Zauscher, Micro-Cantilevers with End-Grafted Stimulus-Responsive Polymer Brushes for Actuation and Sensing, Sens. Actuators, B, 2006, 114(1), 371–378, DOI:10.1016/j.snb.2005.06.003.
- G. G. Bumbu, G. Kircher, M. Wolkenhauer, R. Berger and J. S. Gutmann, Synthesis and Characterization of Polymer Brushes on Micromechanical Cantilevers, Macromol. Chem. Phys., 2004, 205(13), 1713–1720, DOI:10.1002/macp.200400195.
- A. Johansson, G. Blagoi and A. Boisen, Polymeric Cantilever-Based Biosensors with Integrated Readout, Appl. Phys. Lett., 2006, 89(17), 173505, DOI:10.1063/1.2364843.
- J. H. T. Ransley, M. Watari, D. Sukumaran, R. A. McKendry and A. A. Seshia, SU8 Bio-Chemical Sensor Microarrays, Microelectron. Eng., 2006, 83(4), 1621–1625, DOI:10.1016/j.mee.2006.01.175.
- R. W. Johnstone and M. Parameswaran, Theoretical Limits on the Freestanding Length of Cantilevers Produced by Surface Micromachining Technology, J. Micromech. Microeng., 2002, 12(6), 855, DOI:10.1088/0960-1317/12/6/317.
- C. Burbaum, J. Mohr, P. Bley and W. Ehrfeld, Fabrication of Capacitive Acceleration Sensors by the LIGA Technique, Sens. Actuators, A, 1991, 27(1–3), 559–563, DOI:10.1016/0924-4247(91)87051-4.
- H. Kawakatsu, D. Saya, A. Kato, K. Fukushima, H. Toshiyoshi and H. Fujita, Millions of Cantilevers for Atomic Force Microscopy, Rev. Sci. Instrum., 2002, 73(3), 1188–1192, DOI:10.1063/1.1448137.
- E. M. Chow, G. G. Yaralioglu, C. F. Quate and T. W. Kenny, Characterization of a Two-Dimensional Cantilever Array with through-Wafer Electrical Interconnects, Appl. Phys. Lett., 2002, 80(4), 664–666, DOI:10.1063/1.1435804.
- A. K. Basu, A. Basak and S. Bhattacharya, Geometry and Thickness Dependant Anomalous Mechanical Behavior of Fabricated SU-8 Thin Film Micro-Cantilevers, J. Micromanufacturing, 2020, 3(2), 113–120, DOI:10.1177/2516598420930988.
- M. Z. Ansari and C. Cho, A Study on Increasing Sensitivity of Rectangular Microcantilevers Used in Biosensors, Sensors, 2008, 8(11), 7530–7544, DOI:10.3390/s8117530.
- M. Z. Ansari, C. Cho, J. Kim and B. Bang, Comparison between Deflection and Vibration Characteristics of Rectangular and Trapezoidal Profile Microcantilevers, Sensors, 2009, 9(4), 2706–2718, DOI:10.3390/s90402706.
- S. Fernando, M. Austin and J. Chaffey, Improved Cantilever Profiles for Sensor Elements, J. Phys. D: Appl. Phys., 2007, 40(24), 7652–7655, DOI:10.1088/0022-3727/40/24/009.
- G. Villanueva, J. A. Plaza, J. Montserrat, F. Perez-Murano and J. Bausells, Crystalline Silicon Cantilevers for Piezoresistive Detection of Biomolecular Forces, Microelectron. Eng., 2008, 85(5), 1120–1123, DOI:10.1016/j.mee.2008.01.082.
- M. Calleja, P. M. Kosaka, Á. San Paulo and J. Tamayo, Challenges for Nanomechanical Sensors in Biological Detection, Nanoscale, 2012, 4(16), 4925–4938, 10.1039/c2nr31102j.
- N. F. Martnez, P. M. Kosaka, J. Tamayo, J. Ramrez, O. Ahumada, J. Mertens, T. D. Hien, C. V. Rijn and M. Calleja, High Throughput Optical Readout of Dense Arrays of Nanomechanical Systems for Sensing Applications, Rev. Sci. Instrum., 2010, 81(12), 125109, DOI:10.1063/1.3525090.
- N. F. Martínez, P. M. Kosaka, J. Tamayo, J. Ramírez, O. Ahumada, J. Mertens, T. D. Hien, C. V. Rijn and M. Calleja, High Throughput Optical Readout of Dense Arrays of Nanomechanical Systems for Sensing Applications, Rev. Sci. Instrum., 2010, 81(12), 125109, DOI:10.1063/1.3525090.
- G. Meyer and N. M. Amer, Novel Optical Approach to Atomic Force Microscopy, Appl. Phys. Lett., 1988, 53(12), 1045–1047, DOI:10.1063/1.100061.
- S. R. Ahmed, M. R. Khan, K. M. S. Islam and M. W. Uddin, Investigation of Stresses at the Fixed End of Deep Cantilever Beams, Comput. Struct., 1998, 69(3), 329–338, DOI:10.1016/S0045-7949(98)00127-8.
- D. L. McCorkle, R. J. Warmack, S. V. Patel, T. Mlsna, S. R. Hunter and T. L. Ferrell, Ethanol Vapor Detection in Aqueous Environments Using Micro-Capacitors and Dielectric Polymers, Sens. Actuators, B, 2005, 107(2), 892–903, DOI:10.1016/j.snb.2004.12.051.
- H. Mahmoodnia and B. A. Ganji, A Novel High Tuning Ratio MEMS Cantilever Variable Capacitor, Microsyst. Technol., 2013, 19(12), 1913–1918, DOI:10.1007/s00542-013-1835-7.
- A. von Schmidsfeld, T. Nörenberg, M. Temmen and M. Reichling, Understanding Interferometry for Micro-Cantilever Displacement Detection, Beilstein J. Nanotechnol., 2016, 7(1), 841–851, DOI:10.3762/bjnano.7.76.
- M. Z. Ansari, C. Cho, J. Kim and B. Bang, Comparison between Deflection and Vibration Characteristics of Rectangular and Trapezoidal Profile Microcantilevers, Sensors, 2009, 9(4), 2706–2718, DOI:10.3390/s90402706.
- M. J. Lachut and J. E. Sader, Effect of Surface Stress on the Stiffness of Cantilever Plates: Influence of Cantilever Geometry, Appl. Phys. Lett., 2009, 95(19), 193505, DOI:10.1063/1.3262347.
- R. Gao, Y. Huang, X. Wen, J. Zhao and S. Liu, Method to Further Improve Sensitivity for High-Order Vibration Mode Mass Sensors with Stepped Cantilevers, IEEE Sens. J., 2017, 17(14), 4405–4411, DOI:10.1109/JSEN.2017.2712629.
- S. Dohn, R. Sandberg, W. Svendsen and A. Boisen, Enhanced Functionality of Cantilever Based Mass Sensors Using Higher Modes, Appl. Phys. Lett., 2005, 86(23), 1–3, DOI:10.1063/1.1948521.
- J. Linden and E. Oesterschulze, Improving the Quality Factor of Cantilevers in Viscous Fluids by the Adaptation of Their Interface, Appl. Phys. Lett., 2012, 100(11), 113511, DOI:10.1063/1.3694264.
- L. Y. Beaulieu, M. Godin, O. Laroche, V. Tabard-Cossa and P. Grütter, Calibrating Laser Beam Deflection Systems for Use in Atomic Force Microscopes and Cantilever Sensors, Appl. Phys. Lett., 2006, 88(8), 083108, DOI:10.1063/1.2177542.
- R. Mishra, W. Grange and M. Hegner, Rapid and Reliable Calibration of Laser Beam Deflection System for Microcantilever-Based Sensor Setups, J. Sensors, 2012, 617386, DOI:10.1155/2012/617386.
- C. Li, X. Ma, Y. Guan, J. Tang and B. Zhang, Microcantilever Array Biosensor for Simultaneous Detection of Carcinoembryonic Antigens and α-Fetoprotein Based on Real-Time Monitoring of the Profile of Cantilever, ACS Sens., 2019, 4(11), 3034–3041, DOI:10.1021/acssensors.9b01604.
- C. Grogan, G. Amarandei, S. Lawless, F. Pedreschi, F. Lyng, F. Benito-Lopez, R. Raiteri and L. Florea, Silicon Microcantilever Sensors to Detect the Reversible Conformational Change of a Molecular Switch, Spiropyan, Sensors, 2020, 20(3), 854, DOI:10.3390/s20030854.
- D. K. Agarwal, A. Kushagra, S. Mukherji and V. Ramgopal Rao, A Study of Surface Stress and Flexural Rigidity of Symmetrically and Asymmetrically Biofunctionalized Microcantilevers, J. Micromech. Microeng., 2020, 30(2), 25009, DOI:10.1088/1361-6439/ab61c7.
- E. Iwase, P.-C. Hui, D. Woolf, A. W. Rodriguez, S. G. Johnson, F. Capasso and M. Lončar, Control of Buckling in Large Micromembranes Using Engineered Support Structures, J. Micromech. Microeng., 2012, 22(6), 065028, DOI:10.1088/0960-1317/22/6/065028.
- H. Pelletier, J. Krier and P. Mille, Characterization of Mechanical Properties of Thin Films Using Nanoindentation Test, Mech. Mater., 2006, 38(12), 1182–1198, DOI:10.1016/J.MECHMAT.2006.02.011.
- R. Maboudian and C. Carraro, Surface chemistry and tribology of MEMS, Annu. Rev. Phys. Chem., 2004, 55(1), 35–54, DOI:10.1146/annurev.physchem.55.091602.094445.
- C. Bîrleanu, M. Pustan, F. Şerdean and V. Merie, AFM Nanotribomechanical Characterization of Thin Films for MEMS Applications, Micromachines, 2021, 13(1), 23, DOI:10.3390/MI13010023.
- J.-Å. Schweitz, Mechanical Characterization of Thin Films by Micromechanical Techniques, MRS Bull., 1992, 17(7), 34–45, DOI:10.1557/S0883769400041646.
- J. R. Barnes, R. J. Stephenson, C. N. Woodburn, S. J. O'Shea, M. E. Welland, T. Rayment, J. K. Gimzewski and C. Gerber, A Femtojoule Calorimeter Using Micromechanical Sensors, Rev. Sci. Instrum., 1994, 65(12), 3793–3798, DOI:10.1063/1.1144509.
- R. Berger, C. Gerber, J. K. Gimzewski, E. Meyer, H. J. Güntherodt, H. J. Gü, H. J. Güntherodt and H. J. Gü, Thermal Analysis Using a Micromechanical Calorimeter, Appl. Phys. Lett., 1996, 69(1), 40–42, DOI:10.1063/1.118111.
- W. Fang, H.-C. Tsai and C.-Y. Lo, Determining Thermal Expansion Coefficients of Thin Films Using Micromachined Cantilevers, Sens. Actuators, A, 1999, 77(1), 21–27, DOI:10.1016/S0924-4247(99)00019-9.
- Y. Nakagawa, R. Schäfer and H.-J. J. Güntherodt, Picojoule and Submillisecond Calorimetry with Micromechanical Probes, Appl. Phys. Lett., 1998, 73(16), 2296–2298, DOI:10.1063/1.121802.
- O. Ahumada, M. M. Pérez-Madrigal, J. Ramirez, D. Curcó, C. Esteves, A. Salvador-Matar, G. Luongo, E. Armelin, J. Puiggalí and C. Alemán, Sensitive Thermal Transitions of Nanoscale Polymer Samples Using the Bimetallic Effect: Application to Ultra-Thin Polythiophene, Rev. Sci. Instrum., 2013, 84(5), 053904, DOI:10.1063/1.4804395.
- J. Tamayo, D. Ramos, J. Mertens and M. Calleja, Effect of the Adsorbate Stiffness on the Resonance Response of Microcantilever Sensors, Appl. Phys. Lett., 2006, 89(22), 224104, DOI:10.1063/1.2388925.
- M. Yun, N. Jung, C. Yim and S. Jeon, Nanomechanical Thermal Analysis of the Effects of Physical Aging on Glass Transitions in PS/PMMA Blend and PS-PMMA Diblock Copolymers, Polymer, 2011, 52(18), 4136–4140, DOI:10.1016/j.polymer.2011.06.051.
- J. Namchul and J. Sangmin, Nanomechanical Thermal Analysis with Silicon Cantilevers of the Mechanical Properties of Poly(Vinyl Acetate) near the Glass Transition Temperature, Macromolecules, 2008, 41(24), 9819–9822, DOI:10.1021/ma8020695.
- S. Kim, D. Lee, M. Yun, N. Jung, S. Jeon and T. Thundat, Multi-Modal Characterization of Nanogram Amounts of a Photosensitive Polymer, Appl. Phys. Lett., 2013, 102(2), 024103, DOI:10.1063/1.4788740.
- J. L. Corbeil, N. V. Lavrik, S. Rajic and P. G. Datskos, “Self-Leveling” Uncooled Microcantilever Thermal Detector, Appl. Phys. Lett., 2002, 81(7), 1306–1308, DOI:10.1063/1.1498870.
- M. Lopes-Rodrigues, D. Martí-Balleste, C. Michaux, E. A. Perpète, J. Puiggalí, M. M. Pérez-Madrigal and C. Alemán, Nanofeatures Affect the Thermal Transitions of Polymer Thin Films: A Microcantilever-Based Investigation, Mater. Adv., 2020, 1(6), 2084–2094, 10.1039/D0MA00459F.
- L. Saturday, L. Wilson, S. Retterer, N. J. Evans, D. Briggs, P. D. Rack and N. Lavrik, Thermal Conductivity of Nano- and Micro-Crystalline Diamond Films Studied by Photothermal Excitation of Cantilever Structures, Diamond Relat. Mater., 2021, 113, 108279, DOI:10.1016/j.diamond.2021.108279.
- M. del Rey, R. A. da Silva, D. Meneses, D. F. S. Petri, J. Tamayo, M. Calleja and P. M. Kosaka, Monitoring Swelling and Deswelling of Thin Polymer Films by Microcantilever Sensors, Sens. Actuators, B, 2014, 204, 602–610, DOI:10.1016/j.snb.2014.08.021.
- S. Lenz, A. Rühm, J. Major, R. Berger and J. S. Gutmann, Softening of PMMA Brushes upon Collapse/Swelling Transition. A Combined Neutron Reflectivity and Nanomechanical Cantilever Sensor Study, Macromolecules, 2011, 44(2), 360–367, DOI:10.1021/ma1021715.
- G.-G. Bumbu, M. Wolkenhauer, G. Kircher, J. S. Gutmann and R. Berger, Micromechanical Cantilever Technique: A Tool for Investigating the Swelling of Polymer Brushes, Langmuir, 2007, 23(4), 2203–2207, DOI:10.1021/la062137u.
- S. Igarashi, A. N. Itakura, M. Toda, M. Kitajima, L. Chu, A. N. Chifen, R. Förch and R. Berger, Swelling Signals of Polymer Films Measured by a Combination of Micromechanical Cantilever Sensor and Surface Plasmon Resonance Spectroscopy, Sens. Actuators, B, 2006, 117(1), 43–49, DOI:10.1016/j.snb.2005.11.001.
- G. M. A. Alves, S. B. Goswami, R. D. Mansano and A. Boisen, Using Microcantilever Sensors to Measure Poly(Lactic-Co-Glycolic Acid) Plasticization by Moisture Uptake, Polym. Test., 2018, 65, 407–413, DOI:10.1016/j.polymertesting.2017.12.017.
- F. Zhou, W. Shu, M. E. Welland and W. T. S. Huck, Highly Reversible and Multi-Stage Cantilever Actuation Driven by Polyelectrolyte Brushes, J. Am. Chem. Soc., 2006, 128(16), 5326–5327, DOI:10.1021/ja060649p.
- D. S. VanBlarcom and N. A. Peppas, Microcantilever Sensing Arrays from Biodegradable, PH-Responsive Hydrogels, Biomed. Microdevices, 2011, 13(5), 829–836, DOI:10.1007/s10544-011-9553-3.
- G. M. A. Alves, S. B. Goswami, R. D. Mansano and A. Boisen, Temperature Modulated Nanomechanical Thermal Analysis, IEEE Sens. J., 2018, 18(10), 4001–4007, DOI:10.1109/JSEN.2018.2819898.
- J. K. Gimzewski, Ch. Gerber, E. Meyer and R. R. Schlittler, Observation of a Chemical Reaction Using a Micromechanical Sensor, Chem. Phys. Lett., 1994, 217(5–6), 589–594, DOI:10.1016/0009-2614(93)E1419-H.
- K. Jiang, F. Khan, J. Thomas, P. R. Desai, A. Phani, S. Das and T. Thundat, Thermomechanical Responses of Microfluidic Cantilever Capture DNA Melting and Properties of DNA Premelting States Using Picoliters of DNA Solution, Appl. Phys. Lett., 2019, 114(17), 173703, DOI:10.1063/1.5092333.
- Z. Wang, M. Kimura and T. Ono, Manufacturing and Characterization of Simple Cantilever Thermal Biosensor with Si-Metal Thermocouple Structure for Enzymatic Reaction Detection, Thermochim. Acta, 2018, 668, 110–115, DOI:10.1016/J.TCA.2018.08.020.
- N. Abedinov, P. Grabiec, T. Gotszalk, T. Ivanov, J. Voigt and I. W. Rangelow, Micromachined Piezoresistive Cantilever Array with Integrated Resistive Microheater for Calorimetry and Mass Detection, J. Vac. Sci. Technol., A, 2001, 19(6), 2884, DOI:10.1116/1.1412654.
- M. Su and V. P. Dravid, Surface Combustion Microengines Based on Photocatalytic Oxidations of Hydrocarbons at Room Temperature, Nano Lett., 2005, 5(10), 2023–2028, DOI:10.1021/nl0515605.
- A. Alodhayb, F. Khan, H. Etayash and T. Thundat, Nanomechanical Calorimetric Infrared Spectroscopy Using Bi-Material Microfluidic Cantilevers, J. Electrochem. Soc., 2020, 167(3), 037504, DOI:10.1149/2.0042003JES.
- O. Nakabeppu and K. Deno, Nano-DTA and Nano-DSC with Cantilever-Type Calorimeter, Thermochim. Acta, 2016, 637, 1–10, DOI:10.1016/j.tca.2016.05.005.
- H. T. Huy, L. T. Dat and N. D. Vy, Nonmonotonic Dependence of Bimaterial Cantilever Deflection on the Coating Thickness and the Optimum Thermal Sensitivity, J. Appl. Phys., 2017, 122(22), 224502, DOI:10.1063/1.5003870.
- D. Lee, S. Kim, I. Chae, S. Jeon and T. Thundat, Nanowell-Patterned TiO 2 Microcantilevers for Calorimetric Chemical Sensing, Appl. Phys. Lett., 2014, 104(14), 141903, DOI:10.1063/1.4870535.
- M. Yun, S. Kim, D. Lee, N. Jung, I. Chae, S. Jeon and T. Thundat, Photothermal Cantilever Deflection Spectroscopy of a Photosensitive Polymer, Appl. Phys. Lett., 2012, 100(20), 204103, DOI:10.1063/1.4719521.
- G. Li, L. W. Burggraf and W. P. Baker, Photothermal Spectroscopy Using Multilayer Cantilever for Chemical Detection, Appl. Phys. Lett., 2000, 76(9), 1122–1124, DOI:10.1063/1.125988.
- T. Thundat, G. Y. Chen, R. J. Warmack, D. P. Allison and E. A. Wachter, Vapor Detection Using Resonating Microcantilevers, Anal. Chem., 1995, 67(3), 519–521, DOI:10.1021/ac00099a006.
- J. Xu, M. Bertke, H. S. Wasisto and E. Peiner, Piezoresistive Microcantilevers for Humidity Sensing, J. Micromech. Microeng., 2019, 29(5), 053003, DOI:10.1088/1361-6439/ab0cf5.
-
N. Kalaiyazhagan and T. Shanmuganantham, Performance Analysis of MEMS Cantilever Sensor for Agriculture Applications, 2017 IEEE International Conference on Circuits and Systems (ICCS), 2017, 186–190, DOI:10.1109/ICCS1.2017.8325987.
- X. Shi, Q. Chen, J. Fang, K. Varahramyan and H.-F. Ji, Al2O3-Coated Microcantilevers for Detection of Moisture at Ppm Level, Sens. Actuators, B, 2008, 129(1), 241–245, DOI:10.1016/j.snb.2007.08.019.
- D. Lee, N. Shin, K.-H. Lee and S. Jeon, Microcantilevers with Nanowells as Moisture Sensors, Sens. Actuators, B, 2009, 137(2), 561–565, DOI:10.1016/j.snb.2009.01.031.
-
J. Xu, M. Bertke, X. Li, A. Gad, H. Zhou, H. S. Wasisto and E. Peiner, Gravimetric Humidity Sensor Based on ZnO Nanorods Covered Piezoresistive Si Microcantilever, ed. L. Fonseca, M. Prunnila and E. Peiner, 2017, p. 102460C, DOI:10.1117/12.2264897.
-
J. Xu, M. Bertke, X. Li, H. Mu, F. Yu, A. Schmidt, A. Bakin and E. Peiner, Self-Actuating and Self-Sensing ZNO Nanorods/Chitosan Coated Piezoresistive Silicon Microcantilever for Humidit Y Sensing, 2018 IEEE Micro Electro Mechanical Systems (MEMS), 2018, 206–209, DOI:10.1109/MEMSYS.2018.8346520.
- X. Cha, F. Yu, Y. Fan, J. Chen, L. Wang, Q. Xiang, Z. Duan and J. Xu, Superhydrophilic ZnO Nanoneedle Array: Controllable in Situ Growth on QCM Transducer and Enhanced Humidity Sensing Properties and Mechanism, Sens. Actuators, B, 2018, 263, 436–444, DOI:10.1016/J.SNB.2018.01.110.
- M. Kuş and S. Okur, Electrical Characterization of PEDOT:PSS beyond Humidity Saturation, Sens. Actuators, B, 2009, 143(1), 177–181, DOI:10.1016/J.SNB.2009.08.055.
-
A. Sappat, A. Wisitsoraat, C. Sriprachuabwong, K. Jaruwongrungsee, T. Lomas and A. Tuantranont, Humidity Sensor Based on Piezoresistive Microcantilever with Inkjet Printed PEDOT/PSS Sensing Layers, in The 8th Electrical Engineering/ Electronics, Computer, Telecommunications and Information Technology (ECTI) Association of Thailand - Conference 2011, 2011, pp. 34–37, DOI:10.1109/ECTICON.2011.5947764.
- C. Steffens, A. N. Brezolin and J. Steffens, Conducting Polymer-Based Cantilever Sensors for Detection Humidity, Scanning, 2018, 2018, 1–6, DOI:10.1155/2018/4782685.
- R. Likhite, A. Banerjee, A. Majumder, M. Karkhanis, H. Kim and C. H. Mastrangelo, Parametrically Amplified Low-Power MEMS Capacitive Humidity Sensor, Sensors, 2019, 19(18), 3954, DOI:10.3390/s19183954.
- X. Le, L. Peng, J. Pang, Z. Xu, C. Gao and J. Xie, Humidity Sensors Based on AlN Microcantilevers Excited at High-Order Resonant Modes and Sensing Layers of Uniform Graphene Oxide, Sens. Actuators, B, 2019, 283, 198–206, DOI:10.1016/j.snb.2018.12.021.
- J. Pang, X. Le, K. Pang, Z. Xu, C. Gao and J. Xie, Modified Coefficient of Equivalent Mass to Explain Decreased Relative Sensitivity of Piezoelectric Cantilever Humidity Sensor in High Mode, J. Microelectromech. Syst., 2020, 29(4), 452–454, DOI:10.1109/JMEMS.2020.2992673.
- Z. Yuan, H. Tai, Z. Ye, C. Liu, G. Xie, X. Du and Y. Jiang, Novel Highly Sensitive QCM Humidity Sensor with Low Hysteresis Based on Graphene Oxide (GO)/Poly(Ethyleneimine)
Layered Film, Sens. Actuators, B, 2016, 234, 145–154, DOI:10.1016/j.snb.2016.04.070.
- W. Xuan, M. Cole, J. W. Gardner, S. Thomas, F.-H. Villa-López, X. Wang, S. Dong and J. Luo, A Film Bulk Acoustic Resonator Oscillator Based Humidity Sensor with Graphene Oxide as the Sensitive Layer, J. Micromech. Microeng., 2017, 27(5), 055017, DOI:10.1088/1361-6439/aa654e.
- C. Grogan, F. R. McGovern, R. Staines, G. Amarandei and I. Naydenova, Cantilever-Based Sensor Utilizing a Diffractive Optical Element with High Sensitivity to Relative Humidity, Sensors, 2021, 21(5), 1673, DOI:10.3390/s21051673.
- S. Bianco, M. Cocuzza, S. Ferrero, E. Giuri, G. Piacenza, C. F. Pirri, A. Ricci, L. Scaltrito, D. Bich, A. Merialdo, P. Schina and R. Correale, Silicon Resonant Microcantilevers for Absolute Pressure Measurement, J. Vac. Sci. Technol., B: Microelectron. Nanometer Struct.--Process., Meas., Phenom., 2006, 24(4), 1803, DOI:10.1116/1.2214698.
- G. Keskar, B. Elliott, J. Gaillard, M. J. Skove and A. M. Rao, Using Electric Actuation and Detection of Oscillations in Microcantilevers for Pressure Measurements, Sens. Actuators, A, 2008, 147(1), 203–209, DOI:10.1016/j.sna.2008.04.017.
- H. Takahashi, N. M. Dung, K. Matsumoto and I. Shimoyama, Differential Pressure Sensor Using a Piezoresistive Cantilever, J. Micromech. Microeng., 2012, 22(5), 055015, DOI:10.1088/0960-1317/22/5/055015.
- N. Minh-Dung, H. Takahashi, T. Uchiyama, K. Matsumoto and I. Shimoyama, A Barometric Pressure Sensor Based on the Air-Gap Scale Effect in a Cantilever, Appl. Phys. Lett., 2013, 103(14), 143505, DOI:10.1063/1.4824027.
-
R. Watanabe, N. Minh-Dung, H. Takahashi, T. Takahata, K. Matsumoto and I. Shimoyama, Fusion of Cantilever and Diaphragm Pressure Sensors According to Frequency Characteristics, in 2015 28th IEEE International Conference on Micro Electro Mechanical Systems (MEMS), 2015, pp. 212–214, DOI:10.1109/MEMSYS.2015.7050924.
- R. Wada and H. Takahashi, Time Response Characteristics of a Highly Sensitive Barometric Pressure Change Sensor Based on MEMS Piezoresistive Cantilevers, Jpn. J. Appl. Phys., 2020, 59(7), 070906, DOI:10.35848/1347-4065/ab9ba1.
-
N. Krakover, R. Ilic and S. Krylov, Resonant Pressure Sensing Using a Micromechanical Cantilever Actuated by Fringing Electrostatic Fields, in 2018 IEEE Micro Electro Mechanical Systems (MEMS), 2018, pp. 846–849, DOI:10.1109/MEMSYS.2018.8346688.
- J. Fu, T. Zhu, Y. Liang, Z. Liu, R. Wang, X. Zhang and H.-X. Wang, Fabrication of Capacitive Pressure Sensor Using Single Crystal Diamond Cantilever Beam, Sci. Rep., 2019, 9(1), 4699, DOI:10.1038/s41598-019-40582-x.
-
N. Subbiah, Q. Feng, J. Wilde and G. Bruckner, High-Temperature Pressure Sensor Package and Characterization of Thermal Stress in the Assembly up to 500 °C, in 2019 IEEE 69th Electronic Components and Technology Conference (ECTC), 2019, pp. 878–883, DOI:10.1109/ECTC.2019.00137.
- T.-V. Nguyen, Y. Mizuki, T. Tsukagoshi, T. Takahata, M. Ichiki and I. Shimoyama, MEMS-Based Pulse Wave Sensor Utilizing a Piezoresistive Cantilever, Sensors, 2020, 20(4), 1052, DOI:10.3390/s20041052.
- T. Thundat, E. A. Wachter, S. L. Sharp and R. J. Warmack, Detection of Mercury Vapor Using Resonating Microcantilevers, Appl. Phys. Lett., 1995, 66(13), 1695–1697, DOI:10.1063/1.113896.
- B. Rogers, L. Manning, M. Jones, T. Sulchek, K. Murray, B. Beneschott, J. D. Adams, Z. Hu, T. Thundat, H. Cavazos and S. C. Minne, Mercury Vapor Detection with a Self-Sensing,
Resonating Piezoelectric Cantilever, Rev. Sci. Instrum., 2003, 74(11), 4899–4901, DOI:10.1063/1.1614876.
-
L. Senesac and T. Thundat, Explosive Vapor Detection Using Microcantilever Sensors, in Counterterrorist Detection Techniques of Explosives, Elsevier, 2007, pp. 109–130, DOI:10.1016/B978-044452204-7/50024-9.
- L. A. Pinnaduwage, V. Boiadjiev, J. E. Hawk and T. Thundat, Sensitive Detection of Plastic Explosives with Self-Assembled Monolayer-Coated Microcantilevers, Appl. Phys. Lett., 2003, 83(7), 1471–1473, DOI:10.1063/1.1602156.
- Y. Chen, P. Xu and X. Li, Self-Assembling Siloxane Bilayer Directly on SiO 2 Surface of Micro-Cantilevers for Long-Term Highly Repeatable Sensing to Trace Explosives, Nanotechnology, 2010, 21(26), 265501, DOI:10.1088/0957-4484/21/26/265501.
- D. Strle, B. Štefane, E. Zupanič, M. Trifkovič, M. Maček, G. Jakša, I. Kvasič and I. Muševič, Sensitivity Comparison of Vapor Trace Detection of Explosives Based on Chemo-Mechanical Sensing with Optical Detection and Capacitive Sensing with Electronic Detection, Sensors, 2014, 14(7), 11467–11491, DOI:10.3390/s140711467.
-
E. Holthoff, L. Li, T. Hiller and K. Foster, A Molecularly Imprinted Polymer (MIP)-Coated Microbeam MEMS Sensor for Chemical Detection, Chemical, Biological, Radiological, Nuclear, and Explosives (CBRNE) Sensing XVI, 2015, p. 94550W, DOI:10.1117/12.2179694.
- S. Udara, H. C. Hadimani and P. K. Revankar, Sensitivity and Selectivity Enhancement of MEMS/NEMS Cantilever by Coating of Polyvinylpyrrolidone, Mater. Today: Proc., 2019, 18, 1610–1619, DOI:10.1016/j.matpr.2019.05.255.
- A. Shemesh, T. Blank, S. Meltzman, S. Stolyarova, R. Edrei, E. Borzin, Y. Nemirovsky and Y. Eichen, Plasticization of a Polymer Layer Harnessed to a Silicon Microcantilever as a Highly Sensitive and Selective Means to Detect Nitroaromatic Derivatives, J. Polym. Sci., Part A: Polym. Chem., 2014, 52(15), 2124–2130, DOI:10.1002/pola.27219.
- A. R. Krause, C. Van Neste, L. Senesac, T. Thundat and E. Finot, Trace Explosive Detection Using Photothermal Deflection Spectroscopy, J. Appl. Phys., 2008, 103(9), 094906, DOI:10.1063/1.2908181.
- M. P. Pina Iritia, F. Almazan, A. Eguizabal, I. Pellejero, M. Urbiztondo, J. Sese, J. Santamaria, D. Garcia-Romeo, B. Calvo and N. Medrano, Explosives Detection by Array of Si Micro-Cantilevers Coated With Titanosilicate-Type Nanoporous Materials, IEEE Sens. J., 2016, 16(10), 3435–3443, DOI:10.1109/JSEN.2015.2451732.
- D. Lee, S. Kim, S. Jeon and T. Thundat, Direct Detection and Speciation of Trace Explosives Using a Nanoporous Multifunctional Microcantilever, Anal. Chem., 2014, 86(10), 5077–5082, DOI:10.1021/ac500745g.
- D. Lee, S. Kim, C. W. Van Neste, M. Lee, S. Jeon and T. Thundat, Photoacoustic Spectroscopy of Surface Adsorbed Molecules Using a Nanostructured Coupled Resonator Array, Nanotechnology, 2014, 25(3), 035501, DOI:10.1088/0957-4484/25/3/035501.
- V. S. Palaparthy, S. N. Doddapujar, S. G. Surya, S. A. Chandorkar, S. Mukherji, M. S. Baghini and V. R. Rao, Hybrid Pattern Recognition for Rapid Explosive Sensing With Comprehensive Analysis, IEEE Sens. J., 2021, 21(6), 8011–8019, DOI:10.1109/JSEN.2020.3047271.
- S. J. Patil, N. Duragkar and V. R. Rao, An Ultra-Sensitive Piezoresistive Polymer Nano-Composite Microcantilever Sensor Electronic Nose Platform for Explosive Vapor Detection, Sens. Actuators, B, 2014, 192, 444–451, DOI:10.1016/j.snb.2013.10.111.
- P. Ray, S. Pandey and V. Ramgopal Rao, Development of Graphene Nanoplatelet Embedded Polymer Microcantilever for Vapour Phase Explosive Detection Applications, J. Appl. Phys., 2014, 116(12), 124902, DOI:10.1063/1.4896255.
- P. Ray and V. Ramgopal
Rao, Al-Doped ZnO Thin-Film Transistor Embedded Micro-Cantilever as a Piezoresistive Sensor, Appl. Phys. Lett., 2013, 102(6), 064101, DOI:10.1063/1.4792062.
- M. Kandpal, S. N. Behera, J. Singh, V. Palaparthy and S. Singh, Residual Stress Compensated Silicon Nitride Microcantilever Array with Integrated Poly-Si Piezoresistor for Gas Sensing Applications, Microsyst. Technol., 2020, 26(4), 1379–1385, DOI:10.1007/s00542-019-04670-2.
- V. Seena, A. Fernandes, P. Pant, S. Mukherji and V. Ramgopal Rao, Polymer Nanocomposite Nanomechanical Cantilever Sensors: Material Characterization, Device Development and Application in Explosive Vapour Detection, Nanotechnology, 2011, 22(29), 295501, DOI:10.1088/0957-4484/22/29/295501.
- V. Seena, A. Fernandes, S. Mukherji and V. R. Rao, Photoplastic Microcantilever Sensor Platform for Explosive Detection, Int. J. Nanosci., 2011, 10(04n05), 739–743, DOI:10.1142/S0219581X11008861.
- T. Cottineau, S. N. Pronkin, M. Acosta, C. Mény, D. Spitzer and V. Keller, Synthesis of Vertically Aligned Titanium Dioxide Nanotubes on Microcantilevers for New Nanostructured Micromechanical Sensors for Explosive Detection, Sens. Actuators, B, 2013, 182, 489–497, DOI:10.1016/j.snb.2013.03.049.
- D. Wang, A. Chen, S. H. Jang, H. L. Yip and A. K. Y. Jen, Sensitivity of Titania(B) Nanowires to Nitroaromatic and Nitroamino Explosives at Room Temperature via Surface Hydroxyl Groups, J. Mater. Chem., 2011, 21(20), 7269–7273, 10.1039/C1JM10124B.
- D. Spitzer, T. Cottineau, N. Piazzon, S. Josset, F. Schnell, S. N. Pronkin, E. R. Savinova and V. Keller, Bio-Inspired Nanostructured Sensor for the Detection of Ultralow Concentrations of Explosives, Angew. Chem., Int. Ed., 2012, 51(22), 5334–5338, DOI:10.1002/anie.201108251.
- L. Schlur, M. Hofer, A. Ahmad, K. Bonnot, M. Holz and D. Spitzer, Cu(OH)2 and CuO Nanorod Synthesis on Piezoresistive Cantilevers for the Selective Detection of Nitrogen Dioxide, Sensors, 2018, 18(4), 1108, DOI:10.3390/s18041108.
-
D. García-Romeo, B. Calvo, N. Medrano, M. P. Pina, F. Almazan, I. Pellejero, M. Urbiztondo, J. Sese and J. Santamaria, Portable Lock-in Amplifier for Microcantilever Based Sensor Array. Application to Explosives Detection Using Co-BEA Type Zeolites as Sensing Materials, IEEE Sensors 2014 Proceedings, 2014, pp. 1419–1422, DOI:10.1109/ICSENS.2014.6985279.
- W. Ruan, Y. Li, Z. Tan, L. Liu, K. Jiang and Z. Wang, In Situ Synthesized Carbon Nanotube Networks on a Microcantilever for Sensitive Detection of Explosive Vapors, Sens. Actuators, B, 2013, 176, 141–148, DOI:10.1016/j.snb.2012.10.026.
- W. Ruan, Z. Wang, Y. Li and L. Liu, A Microcalorimeter Integrated With Carbon Nanotube Interface Layers for Fast Detection of Trace Energetic Chemicals, J. Microelectromech. Syst., 2013, 22(1), 152–162, DOI:10.1109/JMEMS.2012.2220526.
- O. Zandieh and S. Kim, Sensitive and Selective Detection of Adsorbed Explosive Molecules Using Opto-Calorimetric Infrared Spectroscopy and Micro-Differential Thermal Analysis, Sens. Actuators, B, 2016, 231, 393–398, DOI:10.1016/j.snb.2016.03.046.
- Y. Tao, D. Kong, T. Mei, T. Zhang, W. Lu and D. Zhang, Development of an Explosive Detector with a MEMS Sensor Array, Int. J. Inf. Acquis., 2004, 01(04), 337–343, DOI:10.1142/S0219878904000343.
- A. Ccanccapa, A. Masiá, V. Andreu and Y. Picó, Spatio-Temporal Patterns of Pesticide Residues in the Turia and Júcar Rivers (Spain), Sci. Total Environ., 2016, 540, 200–210, DOI:10.1016/j.scitotenv.2015.06.063.
- D. K. Muenchen, J. Martinazzo, A. N. Brezolin, A. M. de Cezaro, A. A. Rigo, M. N. Mezarroba, A. Manzoli, F. de Lima Leite, J. Steffens and C. Steffens, Cantilever Functionalization Using Peroxidase Extract of Low Cost for Glyphosate Detection, Appl. Biochem. Biotechnol., 2018, 186(4), 1061–1073, DOI:10.1007/s12010-018-2799-y.
- J. Martinazzo, D. K. Muenchen, A. N. Brezolin, A. M. Cezaro, A. A. Rigo, A. Manzoli, L. Hoehne, F. L. Leite, J. Steffens and C. Steffens, Cantilever Nanobiosensor Using Tyrosinase to Detect Atrazine in Liquid Medium, J. Environ. Sci. Health, Part B, 2018, 53(4), 229–236, DOI:10.1080/03601234.2017.1421833.
- M. Alvarez, A. Calle, J. Tamayo, L. M. Lechuga, A. Abad and A. Montoya, Development of Nanomechanical Biosensors for Detection of the Pesticide DDT, Biosens. Bioelectron., 2003, 18(5–6), 649–653, DOI:10.1016/S0956-5663(03)00035-6.
-
X. Xia, P. Xu, H. Yu and X. Li, Resonant Micro-Cantilever Chemical Sensor with One-Step Synthesis of -COOH Functionalized Mesoporous-Silica Nanoparticles for Detection of Trace-Level Organophosphorus Pesticide, 2012 IEEE Sensors, 2012, pp. 1–4, DOI:10.1109/ICSENS.2012.6411235.
- C. Karnati, H. Du, H. F. Ji, X. Xu, Y. Lvov, A. Mulchandani, P. Mulchandani and W. Chen, Organophosphorus Hydrolase Multilayer Modified Microcantilevers for Organophosphorus Detection, Biosens. Bioelectron., 2007, 22(11), 2636–2642, DOI:10.1016/J.BIOS.2006.10.027.
- C. Mee-Hie Cho, A. Mulchandani and W. Chen, Functional Analysis of Organophosphorus Hydrolase Variants with High Degradation Activity towards Organophosphate Pesticides, Protein Eng., Des. Sel., 2006, 19(3), 99–105, DOI:10.1093/protein/gzj007.
- M. Zourob, K. G. Ong, K. Zeng, F. Mouffouk and C. A. Grimes, A Wireless Magnetoelastic Biosensor for the Direct Detection of Organophosphorus Pesticides, Analyst, 2007, 132(4), 338–343, 10.1039/B616035B.
-
U. Biapo, A. Ghisolfi, G. Gerer, D. Spitzer, V. Keller and T. Cottineau, Nanostructured and Functionalized Cantilever for Sensing Organophosphorous Compounds, 2019 IEEE Sensors, 2019, pp. 1–4, DOI:10.1109/SENSORS43011.2019.8956854.
- D. Lee, Y. Jin, N. Jung, J. J. Lee, J. J. Lee, Y. S. Jeong and S. Jeon, Gravimetric Analysis of the Adsorption and Desorption of CO2 on Amine-Functionalized Mesoporous Silica Mounted on a Microcantilever Array, Environ. Sci. Technol., 2011, 45(13), 5704–5709, DOI:10.1021/es200680v.
- T. D. Caliskan, D. A. Bruce and M. F. Daqaq, Micro-Cantilever Sensors for Monitoring Carbon Monoxide Concentration in Fuel Cells, J. Micromech. Microeng., 2020, 30(4), 045005, DOI:10.1088/1361-6439/ab6df2.
- M. Denoual, D. Robbes, S. Inoue, Y. Mita, J. Grand, H. Awala and S. Mintova, Thermal Resonant Zeolite-Based Gas Sensor, Sens. Actuators, B, 2017, 245, 179–182, DOI:10.1016/j.snb.2017.01.131.
- C. V. B. Reddy, V. R. Rao, M. A. Khaderbad, S. Gandhi, M. Kandpal, S. Patil, K. N. Chetty, K. G. Rajulu, P. C. K. Chary and M. Ravikanth, Piezoresistive SU-8 Cantilever With Fe(III)Porphyrin Coating for CO Sensing, IEEE Trans. Nanotechnol., 2012, 11(4), 701–706, DOI:10.1109/TNANO.2012.2190619.
-
F. Ahmad, Z. Tufail, S. Farid, S. M. A. Gilani, R. T. Mehmood and T. Izhar, Optimization of MEMS PZR Carbon Dioxide Sensor, 2019 International Conference on Computer and Information Sciences (ICCIS), 2019, pp. 1–5, DOI:10.1109/ICCISci.2019.8716440.
- B. H. Kim, F. E. Prins, D. P. Kern, S. Raible and U. Weimar, Multicomponent Analysis and Prediction with a Cantilever Array Based Gas Sensor, Sens. Actuators, B, 2001, 78(1–3), 12–18, DOI:10.1016/S0925-4005(01)00785-7.
- D. Then, A. Vidic and C. Ziegler, A Highly Sensitive Self-Oscillating Cantilever Array for the Quantitative and Qualitative Analysis of Organic Vapor Mixtures, Sens. Actuators, B, 2006, 117(1), 1–9, DOI:10.1016/j.snb.2005.07.069.
- M. Toda, K. Moorthi, T. Hokama, Z. Wang, M. Yamazaki and T. Ono, Miniature Piezoresistive Sensor for Detecting Volatile Organic Components, Sens. Actuators, B, 2021, 333, 129524, DOI:10.1016/j.snb.2021.129524.
- N. Kilinc, O. Cakmak, A. Kosemen, E. Ermek, S. Ozturk, Y. Yerli, Z. Z. Ozturk and H. Urey, Fabrication of 1D ZnO Nanostructures on MEMS Cantilever for VOC Sensor Application, Sens. Actuators, B, 2014, 202, 357–364, DOI:10.1016/j.snb.2014.05.078.
- Y. Bao, P. Xu, S. Cai, H. Yu and X. Li, Detection of Volatile-Organic-Compounds (VOCs) in Solution Using Cantilever-Based Gas Sensors, Talanta, 2018, 182, 148–155, DOI:10.1016/j.talanta.2018.01.086.
- X. Zhang, Z. Cheng and X. Li, Cantilever Enhanced Photoacoustic Spectrometry: Quantitative Analysis of the Trace H2S Produced by SF6 Decomposition, Infrared Phys. Technol., 2016, 78, 31–39, DOI:10.1016/j.infrared.2016.07.004.
- L. Tang, P. Xu, M. Li, H. Yu and X. Li, H2S Gas Sensor Based on Integrated Resonant Dual-Microcantilevers with High Sensitivity and Identification Capability, Chin. Chem. Lett., 2020, 31(8), 2155–2158, DOI:10.1016/j.cclet.2020.01.018.
- R.-P. Peng, L.-B. Xing, X.-J. Wang, C.-J. Wu, B. Chen, H.-F. Ji, L.-Z. Wu and C.-H. Tung, A Beryllium-Selective Microcantilever Sensor Modified with Benzo-9-Crown-3 Functionalized Polymer Brushes, Anal. Methods, 2017, 9(22), 3356–3360, 10.1039/C7AY00490G.
- F. Zhou, P. M. Biesheuvel, E.-Y. Choi, W. Shu, R. Poetes, U. Steiner and W. T. S. Huck, Polyelectrolyte Brush Amplified Electroactuation of Microcantilevers, Nano Lett., 2008, 8(2), 725–730, DOI:10.1021/nl073157z.
- N. Schüwer and H. A. Klok, A Potassium-Selective Quartz Crystal Microbalance Sensor Based on Crown-Ether Functionalized Polymer Brushes, Adv. Mater., 2010, 22(30), 3251–3255, DOI:10.1002/ADMA.201000377.
- T. Chen, D. P. Chang, T. Liu, R. Desikan, R. Datar, T. Thundat, R. Berger and S. Zauscher, Glucose-Responsive Polymer Brushes for Microcantilever Sensing, J. Mater. Chem., 2010, 20(17), 3391–3395, 10.1039/b925583d.
- M. Nordström, D. A. Zauner, M. Calleja, J. Hübner and A. Boisen, Integrated Optical Readout for Miniaturization of Cantilever-Based Sensor System, Appl. Phys. Lett., 2007, 91, 103512, DOI:10.1063/1.2779851.
- H.-F. Ji, K. M. Hansen, Z. Hu and T. Thundat, Detection of PH Variation Using Modified Microcantilever Sensors, Sens. Actuators, B, 2001, 72(3), 233–238, DOI:10.1016/S0925-4005(00)00678-X.
- R. Bashir, J. Z. Hilt, O. Elibol, A. Gupta and N. A. Peppas, Micromechanical Cantilever as an Ultrasensitive PH Microsensor, Appl. Phys. Lett., 2002, 81(16), 3091–3093, DOI:10.1063/1.1514825.
- E. Finot, T. Thundat, E. Lesniewska and J. P. Goudonnet, Measuring Magnetic Susceptibilities of Nanogram Quantities of Materials Using Microcantilevers, Ultramicroscopy, 2001, 86(1–2), 175–180, DOI:10.1016/s0304-3991(00)00080-2.
- C. Ricciardi, G. Canavese, R. Castagna, I. Ferrante, A. Ricci, S. L. Marasso, L. Napione and F. Bussolino, Integration of microfluidic and cantilever technology for biosensing application in liquid environment, Biosens. Bioelectron., 2010, 26(4), 1565–1570, DOI:10.1016/j.bios.2010.07.114.
- M. Faheem Khan, S. Kim, D. Lee, S. Schmid, A. Boisen and T. Thundat, Nanomechanical identification of liquid reagents in a microfluidic channel, Lab Chip, 2014, 14, 1302–1307, 10.1039/C3LC51273H.
- H. Etayash, M. F. Khan, K. Kaur and T. Thundat, Microfluidic cantilever detects bacteria and measures their susceptibility to antibiotics in small confined volumes, Nat. Commun., 2016, 7, 12947, DOI:10.1038/ncomms12947.
- O. Mohd, M. S. Sotoudegan, F. S. Ligler and G. M. Walker, A simple cantilever system for measurement of flow rates in paper microfluidic devices, Eng. Res. Express, 2019, 1, 025019, DOI:10.1088/2631-8695/ab4ee5.
- R. Abraham, Y. Yoon, F. Khan, S. A. Bukhari, C. Kim, T. Thundat, H.-J. Chung and J. Lee, Measurement of thermal properties of liquid analytes using microfluidic resonators via photothermal modulation, Sens. Actuators, A, 2022, 347, 113994, DOI:10.1016/j.sna.2022.113994.
- A. Hajesfandiari, V. Sukhotskiy, A. Alodhay, F. Khan, T. Thundat and E. P. Furlani, Microfluidic microcantilever as a sensitive platform to measure evaporation rate of picoliters of ethanol, Measurement, 2021, 173, 108617, DOI:10.1016/j.measurement.2020.108617.
|
This journal is © The Royal Society of Chemistry 2023 |
Click here to see how this site uses Cookies. View our privacy policy here.