DOI:
10.1039/D2MA00896C
(Review Article)
Mater. Adv., 2023,
4, 432-457
Prospects of nanostructure-based electrochemical sensors for drug detection: a review
Received
10th September 2022
, Accepted 21st November 2022
First published on 25th November 2022
Abstract
The present study represents the advancements achieved over the past ten years towards the development of electrochemical sensors based on nanomaterials. The versatility, sensitivity, selectivity, and capability of analyzing samples with minimal to no pre-treatment means that electrochemical sensors are an attractive and powerful tool for detecting some analgesic and antipyretic drugs such as acetaminophen (AP), ibuprofen (IB), aspirin (ASP), and diclofenac (DCF). These analgesic and antipyretic drugs are very popular as minor pain and fever medications. Controlled doses of these drugs do not harm the human body, but higher concentrations can be hazardous for humans. These drugs are also considered to be emerging chemical pollutants in the environment. Reliable and powerful analytical techniques are thus necessary for the detection of these drugs, for the quality control of pharmaceuticals as well as for environmental control. This review emphasizes the synthesis of nanostructured materials and their use in the electrochemical sensing of analgesic and antipyretic drugs.
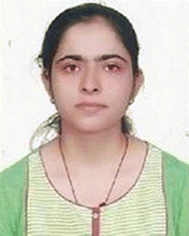
Manika Chaudhary
| Manika Chaudhary has received her MSc in 2016 and MPhil in 2017 from C.C.S University, Meerut (UP), India. Presently, she is pursuing PhD from C.C.S University, Meerut (UP), India. She has published six research papers and one book chapter in reputed joumals. Her research interests comprise of nanostructured materials and metal oxide semiconducting materials for energy storage devices and sensors. |
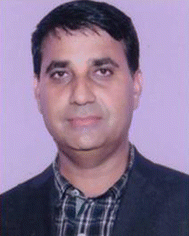
Beer Pal Singh
| Beer Pal Singh has received his MSc (1997), MPhil (1998) and PhD (2002) from C.C.S. University, Meerut (UP), India. He is holding faculty position in Physics at C.C.S. University, Meerut since 2004. Presently, he is working as “Professor” and Head in Department of Physics, CCS University, Meerut. In addition to this, he is also holding the post of Proctor, Security Officer and Dy Director, Centre for International Cooperation in University Administration. Recently, he had worked as Visiting Professor in Tokyo University of Science, Tokyo, Japan and Visiting Scientist (Raman Fellow) in University of Puerto Rico, Mayaguez PR, USA for one year. He has also visited Germany, France, China, and Boston and presented his research work. He has supervised 09 PhD and more than 40 MPhil Students for their research thesis. He has published more than 60 research papers in reputed journals and serving as a reviewer of several national/international journal or repute. His research interests comprise of thin films, 2D materials, nanostructured materials, metal oxides, semiconducting materials, thin film transistors, sensors and energy storage devices. |
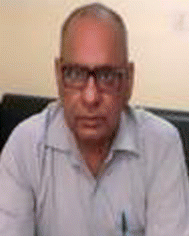
Bansi D. Malhotra
| Dr B. D. Malhotra received his PhD from the University of Delhi, Delhi in 1980. He has published 340 papers in refereed international journals (Citations: 26 883 Research-Index: 85), has filed 11 patents (in India and overseas), and has co-authored text books on ‘Nanomaterials for Biosensors: Fundamentals and Applications’ and ‘Biosensors: Fundamentals and Applications’. He is a recipient of the Narional Research Development Corporation Award 2005 for invention on ‘Blood Glucose Biochemical Analyzer’ and is a Fellow of the Indian National Science Academy, the National Academy of Sciences, India and an Academician of the Asia Pacific Academy of Materials (APAM). Dr Malhotra is a former DST-Science & Engineering Rsearch Board (SERB, Govt of India) Distinguished Fellow. |
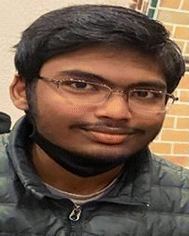
Kushagr Singhal
| Kushagr Singhal is currently a High-school Junior at Rocky Hill High School, CT, USA. He is actively engaged in the research related to synthesis and characterizations of transition metal oxide nanomaterials for electrochemical energy storage devices. His interests are in data analysis, finance, and materials science & engineering. |
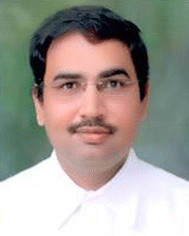
Rakesh K. Sharma
| Dr Rakesh K Sharma, FRSC is an Associate Professor at the Department of Chemistry at IIT Jodhpur, India. He received his BSc and MSc from the University of Rajasthan Jaipur and PhD from the Indian Institute of Science Bangalore in 2008. He worked as a postdoctoral researcher from 2007 to 2010 at the Ohio State University Columbus, USA. He has nine patents and transferred five technologies in bio-fuel, energy storage, environmental technologies, and automotive applications. He has published over 100 articles in peer-reviewed journals, including Journal of American Chemical Society, Chemical Science, ACS Sustainable and Engineering, to name a few. He has also published ten books/book chapters. His research interest includes catalysis for biofuels and fine chemicals, natural clay catalyst, plasma catalysis for environmental remediation, and advanced materials for energy generation and storage. |
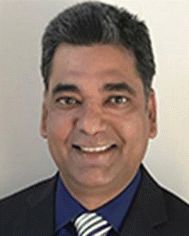
Rahul Singhal
| Dr Rahul Singhal is an Associate Professor at the Department of Physics and Engineering Physics at Central Connecticut State University, New Britain, CT, USA. He received his MSc degree in physics from G.B. Pant University of Agriculture and Technology, Pantnagar in 1997 and PhD degree in physics from University of Delhi, India in 2003. He worked as researcher from 1997–2005 at National Physical Laboratory, New Delhi, India. He worked as postdoctoral fellow from 2005–2012 at various universities in USA, including University of Puerto Rico, Mayaguez; University of Puerto Rico, San Juan; University of South Florida, Tampa, FL. He published over 60 articles in peer-reviewed jornals and 4 book chapters. His research interest includes biosensors based on conducting polymers, metal oxide/sulfide nanomaterial's, and materials for energy storage devices such as super capacitors and rechargeable batteries. |
1. Introduction
Nowadays, drugs have become a part of our daily lives because of their therapeutic and recreational purposes.1 Drugs can be categorized in three groups: (a) therapeutic drugs, (b) legal drugs, and (c) illicit drugs. Therapeutic drugs are those that are generally prescribed by doctors for the treatment of diseases, for example, theophylline to treat lung diseases, and propofol to induce anesthesia during surgical procedures. Legal drugs, such as alcohol, caffeine, and nicotine, are generally consumed for recreational use in commercial products. These drugs, when consumed, provide psychoactive effects in the body. The third group of drugs, i.e., illicit drugs, are consumed for recreational purposes, which harm the central nervous system and thus invite various prolonged health issues.2,3 Although the last category was developed principally for pharmaceutical purposes, their therapeutic use was soon overshadowed by their potential for misuse. For instance, in 1898, Bayer first used ‘heroin’ as a new constituent in cough medicine.3,4 The utilization of therapeutic drugs is continually expanding, with a projected worldwide expenditure of 1.52 trillion US dollars by 2023.5 These sudden increasing trends reflect the essentially aging total populace and the spread of new infections and pandemics, for example, the ongoing existence of COVID-19.6,7 There are various types of therapeutic drugs, such as anesthetics, antibiotics, analgesics, cardioactive drugs, antineoplastic drugs, etc., and various analgesic and antipyretic drugs, such as acetaminophen (paracetamol), ibuprofen, aspirin, diclofenac, ascorbic acid, etc., are consumed by humans in their daily lives. Acetaminophen (AP; paracetamol or N-acetyl-p-aminophenol) is a popular, safe, effective, and extensively used analgesic and antipyretic drug. Acetaminophen is used as fever reducer, and to relieve pain associated with various parts of the body, such as headaches, arthralgia, cancer pain, neuralgia and pain associated with any surgical treatment.8,9 In spite of the fact that AP is a relatively secure medicine, an overdose of any drug may have harmful effects.10 An excess dose of AP may cause nephrotoxicity and lethal hepatotoxicity.11 Ibuprofen (IB) falls in the category of painkillers and anti-pyretic drugs. This drug blocks the enzyme cyclooxygenase, thus inhibiting prostaglandin biosynthesis. Approximately 90% of ibuprofen is converted to the hydroxyl and carboxyl metabolites of ibuprofen in the liver, with the remaining 10% being passed unchanged in urine and bile.12,13 Ibuprofen is high-selling drug worldwide, which is why it is usually the first option for different short-term non-specific indications. It is most commonly used to treat fever symptoms, headaches, arthritis, and a variety of other common aches and pains. The ease of availability and popularity of ibuprofen make it one of the most commonly detected and quantified drugs in pharmaceutical analysis. Novel and progressive analytical approaches with high accuracy are therefore required for the strict control of these drugs in pharmaceutical dosages and different biological fluids.14,15 In the family of analgesic drugs, aspirin (ASP), also known as acetylsalicylic acid, is consumed to relieve minor pain and to reduce fever. It can also be used as a blood thinner. Aspirin decomposes quickly in solutions of ammonium acetate or the acetates, citrates, carbonates, or hydroxides of alkali metals. It is stable in dry air, but when it comes into contact with moisture it slowly hydrolysis to acetic and salicylic acids. It has an adverse effect on the stomach, which can involve stomach ulcers, stomach bleeding, and worsening asthma.16–18 Diclofenac (DCF) is 2-(2-((2,6-dichlorophenyl)amino)phenyl)acetic acid, and is a commonly prescribed analgesic and antipyretic drug because it has strong antipyretic, analgesic, and anti-inflammatory properties.19 It is efficient in acute joint inflammation, rheumatic complaints, and for mild to moderate pain.20 If it is taken in normal therapeutic doses, it is safe and does not have toxic effects on the human body. A high dose of DCF may cause negative effects such as gastrointestinal disorders, aplastic anemia, and a disturbance in renal function.21,22 Generally, all these drugs (except AP, because it has low anti-inflammatory properties) fall in the category of non-steroidal anti-inflammatory drugs (NSAIDs), which are frequently used to treat fever and pain, and control inflammation. However, an overdose of any drug may cause severe problems for the human body; therefore, the precise detection of pharmaceutical specimens is useful for the quality control of medication, avoiding major risks to humans. In this context, various analytical techniques, such as titrimetry,23 spectrofluorometry,24 chemiluminescence,25 liquid chromatography,26 spectrophotometry,27 and electrochemical analysis,28,29 have been proposed for the determination of the concentration of different drugs. In titrimetric, spectrophotometric, and chemiluminescence techniques, an extraction process is needed before detection, whereas liquid chromatography is a time consuming process, which makes these strategies unsuitable for routine examination. For the detection of various drugs, this necessitates a chemical sensor that is fast, precise, reliable, and cost effective. An outline of analytical chemistry development displays that electrochemical sensors constitute the foremost and fastest-developing class of chemical sensors. They deliver continuous data about the presence of chemicals in their surroundings. Ideally, a chemical sensor offers an explicit kind of response that is directly related to the amount of a selected chemical species. The oxidation or reduction of the analyte in an electrolyte is the fundamental principle of electrochemical sensors. The change in electrical parameters that result from such redox reactions are then measured. Cyclic voltammetry (CV), anodic stripping voltammetry (ASV), linear sweep voltammetry (LSV), and differential pulse voltammetry (DPV) are the most commonly used techniques for electrochemical sensors. Among these techniques, the electrochemical method has many advantages, including simplicity, affordability, rapidity, ease of monitoring, and high sensitivity, that play an important role in pharmaceutical analysis. Fig. 1 shows electrochemical sensors based on nanomaterials for the detection of various drugs. These drugs are electroactive compounds that can be electrochemically oxidized. Electrochemical sensors show a captivating choice for the fast determination and measurement of drugs. Using conventional materials, the electrochemical oxidation of these drugs is an irreversible process; however, it becomes reversible due to the existence of catalytic compounds, such as metallic particles, carbon-based nanomaterials and conductive polymers.29–32
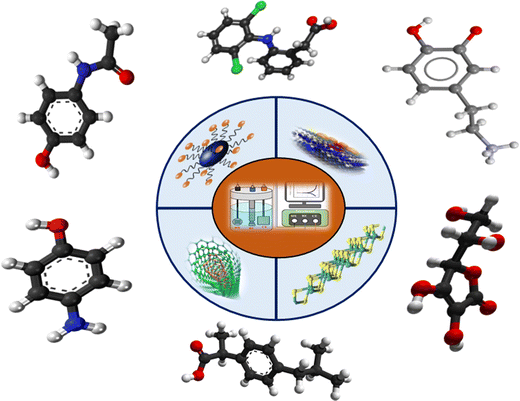 |
| Fig. 1 Schematic of nanomaterials based electrochemical sensors for the detection of different drugs. | |
With noticeable accomplishments in nanoscience and nanotechnology, nanomaterial-based electrochemical signal amplification has acquired an incredible capability for improving both the selectivity and sensitivity of electrochemical sensors. It is broadly known that the electrode materials play a crucial role in the development of superior electrochemical sensing platforms that can distinguish target molecules through different analytical principles. Furthermore, useful nanomaterials not only produce a synergic impact on the conductivity, biocompatibility, and catalytic activity, which speeds up signal transduction, but also enhance biorecognition events using explicitly designed signal labels, leading to highly sensitive biosensing.33,34 In their 2018 report, Montaseri et al. discussed different detection techniques, such as chromatography-mass spectrometry, spectroscopic methods, capillary electrophoresis methods, and electrochemical methods, for acetaminophen detection, and they focused on the water treatment and toxicity of acetaminophen.35 Recently, Qian et al.20 & Chen et al. reported an article in which they discussed that carbon based materials and noble metal nanomaterials play a crucial role in drug sensing due to their high surface-to-volume ratio and high electrical conductivity.36 Li et al. dispersed Pd nanoparticles on a GO sheet to prepare a Pd/GO nanocomposite. The prepared nanocomposite based sensor showed excellent reproducibility and stability, with wider linear concentration ranges (0.005–0.5 μM and 0.5–80 μM) and a low detection limit (2.2 nM) towards the sensing of paracetamol.37 Adekunle et al. prepared an electrochemical sensor using an edge-plane pyrolytic graphite electrode (EPPGE) and modified it with SWCNT–iron(III) oxide (SWCNT/Fe2O3) nanoparticles for the detection of dopamine.38 Ozcelikay et al. developed a sensor for the detection of daptomycin using the integration of Au decorated Pt nanoparticles on a nanocomposite thin film, which showed higher sensitivity.39 So, the main motive of this report is to deliver a general analysis of electrochemical sensors and their sensing capabilities using different nanostructured materials for some previously discussed analgesic and antipyretic drugs. This report also discusses some common methods for the synthesis of nanomaterials and their use in electrochemical sensors for the detection of different drugs.
2. Fabrication of nanostructured materials
2.1 Hydrothermal or solvothermal techniques
The hydrothermal method is an easy and efficient route for the fabrication of nanomaterials. With this process, single and multi-component metal oxide based nanoparticles can be produced with high purity. The crystal growth process is executed using apparatus called autoclave, in which precursors are supplied with water. A temperature difference is sustained at the opposite terminals of the growth chamber so that the cooler end causes seeds to make additional growth and the hotter end dissolves the nutrient. The main superiority of this technique is the synthesis of fine quality crystals with a controlled structure in terms of shape and size. However, this process is difficult to control and it can show limited reliability and reproducibility.40,41 The general synthesis procedure for this method is shown in Fig. 2. Annadurai et al. reported their work on hydrothermally prepared nickel oxide (NiO) modified glassy carbon electrodes. The morphology reveals that the size of the prepared NiO nanoparticles varies between 15 and 20 nm. These NiO modified electrodes were utilized for the sensitive detection of 4-acetaminophen via DPV, CV, and chronoamperometry (CA) techniques.42 Nurzulaikha and co-workers prepared a modified electrode using a graphene/SnO2 nanocomposite, synthesized via a hydrothermal route. They used the fabricated electrodes for the detection of dopamine in the presence of ascorbic acid (AA). The electrode manifested good selectivity, sensitivity and limit of detection in the presence of AA.43 Phosphorus-doped graphene was hydrothermally prepared by the Zhang group and was utilized as the electrode material in order to fabricate electrochemical sensors for AP sensing.44 Xu et al. synthesized the poly(3,4-ethylenedioxythiophene)–MnO2 (PEDOT–MnO2) nanocomposite via a hydrothermal method and used it for the amperometric detection of paracetamol.45 Recently, Ponnada et al. reported the synthesis of the Ag–Cu decorated ZnO nanoflower like composite (NFLC) via a single step hydrothermal method and found it suitable for the detection of dopamine, with a high sensitivity of 0.68 μA mM−1 cm−2 and a low detection limit of 0.21 μM, detected via the DPV method.46
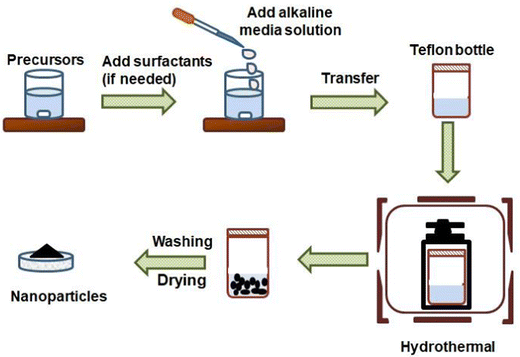 |
| Fig. 2 Schematic of a typical hydrothermal method to synthesize nanomaterials. | |
Lu and co-workers constructed an innovative sensing system by modifying glassy carbon electrodes with a biomass carbon/metal–organic framework derived Co3O4/FeCo2O4(BC/Co3O4/FeCo2O4) composite. The BC/Co3O4/FeCo2O4 composite was prepared via a simple in situ growth process and calcination treatment combined with hydrothermal treatment. First pinecones were carbonized into the BC material, and then the Co-based zeolitic imidazolate framework ZIF-67 was grown in situ on a porous BC matrix; the product was used as the precursor material. Afterwards, the precursor was pyrolyzed to generate the BC/Co3O4 nanocomposite, and then the BC/Co3O4/FeCo2O4 composite was synthesized via a hydrothermal method and calcination. It was reported that the ZIF-67 crystals show rhombic dodecahedral shapes (Fig. 3(A)), and the particle size was found to be in the range of 400–600 nm. The FeCo2O4 powder showed a nanorod like morphology with a diameter in the range of 100–150 nm (Fig. 3(B)), and the FESEM images of the biomass carbon and BC/Co3O4 composite are shown in Fig. 3(C) and (D), respectively. It was also observed that upon BC/Co3O4/FeCo2O4 composite formation (Fig. 3(E) and (F)), the rhombic dodecahedral morphology of the MOF-derived Co3O4 grown on the BC surface turned into nanosheets. The electrochemical sensor developed using this prepared composite exhibited superior electro-conductivity and a vast active surface area because of the synergy effects of the BC, MOF-derived Co3O4 and FeCo2O4.47 Razmi et al. synthesized an Fe3O4/MWCNT nanocomposite using a hydrothermal method and then prepared the TiO2/Fe3O4/MWCNT nanocomposite using a sol–gel method. They developed a sensor with the prepared nanocomposite and used it for the simultaneous detection of morphine and diclofenac.48
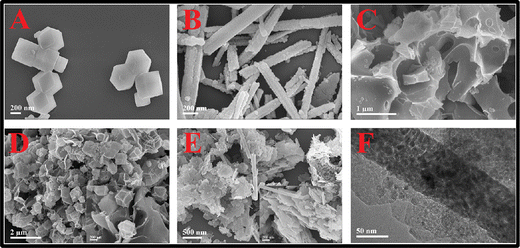 |
| Fig. 3 SEM micrographs of (A) ZIF-67, (B) FeCo2O4, (C) BC, (D) BC/Co3O4 and (E) BC/Co3O4/FeCo2O4 composite; and (F) TEM image of the BC/Co3O4/FeCo2O4 composite (reprinted with permission from ref. 47). | |
2.2 Sol–gel method
This method is very beneficial for the synthesis of composites, oxides, and hybrids of organics and inorganics. In the sol–gel method, firstly, the metal alkoxide solution undergoes hydrolysis with water or an alcoholic solution in the presence of acid or base, followed by polycondensation. Owing to the polycondensation, the water molecules are removed, and the liquid phase is transformed into the gel phase, which increases the viscosity of the solution. Thereafter, the condensation of water molecules takes place, and the gel phase changes into a powder phase. Additional heat is essential for obtaining a fine crystalline powder. The sol–gel method basically uses inorganic polymerization reactions. The superiority of this method is that it is an easy process for the creation of a superfine poriferous powder.49,50 Bagherinasab et al. reported the synthesis of BaFe12O9via a sol–gel method in which citric acid, Ba(NO3)2·6H2O, Fe (NO3)3·9H2O, and NH3 were utilized as the starting materials. The FE-SEM results showed the hexagonal morphology of the BaFe12O19 nanoparticles with a particle mean size of 76 nm.51 Deiminiat et al. succeeded in making an electrochemical imprinted sensor by combining functionalized multiwall carbon nanotubes and a thin molecularly imprinted film for the detection of dopamine. First, they functionalized multiwalled CNTs using nitric acid and carboxylic acid, and then deposited these functionalized multiwalled carbon nanotubes (f-MWCNTs) on glassy carbon electrodes. Later, they deposited the imprinted film on the assembled f-MWCNTs layer the using sol–gel method. The sol solution was synthesized by mixing 75 μL of PTEOS, 75 μL of TEOS, 700 μL of water, 1100 μL of ethanol and 10 μL of TFA. All these chemicals were added to tramadol in a vial and the solution was stirred at room temperature (RT) for 2 h to obtain a homogeneous sol. Thereafter, pyrrole solution (50 μL) and lithium perchlorate (5.0 mg) were added to the mixture, which was subsequently sonicated for 10 min. Then, the polypyrrole@sol–gel MIP/f-MWCNTs/GC electrode was fabricated by applying CV (between −0.8 V and +0.8 V (versus Ag/AgCl) for 10 cycles at a scan rate of 50 mV s−1) in the imprinted sol–gel solution, which displayed a dense and uniform morphology.52 Similarly, sol–gel imprinted polymer based electrochemical sensors for the recognition and detection of paracetamol were synthesized by Zhu et al.53 A sol–gel fabricated Mn2O3–TiO2 decorated graphene electrode was reported for the quick and selective ultra-sensitive sensing of dopamine.54 Luo and co-workers used the one-pot synthesis of a graphene oxide molecularly imprinted polymer sol–gel for the electrochemical sensing of paracetamol, where this sensor possessed a wide detection range, high selectivity, and low LOD along with good stability.55 Rouhani et al. fabricated an electrochemical sensor based on a modified imprinted sol–gel graphite electrode using gold nanoparticles, multiwalled CNTs, and Preyssler heteropolyacid.56
2.3 Co-precipitation method
This method is used widely for the preparation of high-purity, uniform, and multicomponent ceramic precipitates with an exact stoichiometry. An aqueous medium is required for this method. This method requires the mixing of two or more water soluble divalent or trivalent metal ions. These salts undergo a reaction that leads to the precipitation of one or more water soluble salts. This method has a number of characteristics, such as good stoichiometric control, uniform mixing, a low processing time, and the ability to use commercially existing chemicals.57Fig. 4 presents a schematic of the co-precipitation method to synthesize the nanomaterials. Singh et al. synthesized magnetite (Fe3O4) and hematite (α-Fe2O3) iron oxide nanoparticles via a facile co-precipitation method. The synthesized Fe3O4 material was annealed at 700 °C for 2 h to obtain α-Fe2O3 phase nanoparticles. The prepared nanoparticles (Fe3O4 and α-Fe2O3) were used for the modification of a glassy carbon electrode, which was further used for the electrochemical sensing of AP.58
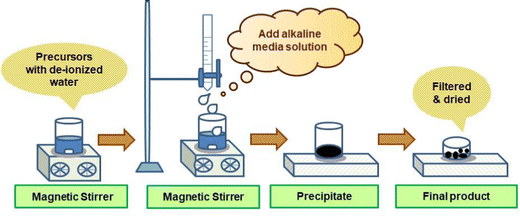 |
| Fig. 4 Schematic of co-precipitation method for the synthesis of nanomaterials. | |
Sivakumar et al. reported the synthesis of the activated carbon–ZnO (AC–ZnO) nanocomposite, where mango leaves were used as the activated carbon source. First, they prepared ZnO via a co-precipitation method using Zn(NO3)2, NaNO3, and NaOH as the precursor materials; then, a 1
:
2 ratio of activated carbon and ZnO powder was mixed in water (10 ml) to prepare the ZnO–AC composite. It was observed that each ZnO nanoflake microsphere was formed via interconnected ultrathin nanosheets (Fig. 5(a) and (b)), and the ZnO nanoflakes were successfully decorated on the activated carbon. The FESEM images of activated carbon at different magnifications are shown in Fig. 5(c) and (d). The ZnO–AC modified GCE was found to be suitable for the detection of acetaminophen within the range of 0.05–1380 μM with a corresponding sensitivity and LOD of about 8.33 μA μM−1 cm−2 and 0.83 μM, respectively. In this study, it was reported that AC provided a large surface area and a better electrochemical performance for sensor applications.59
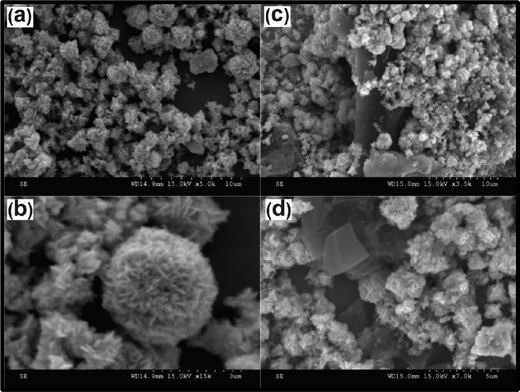 |
| Fig. 5 SEM micrographs at different magnifications for (a) and (b) ZnO nanoflake microspheres, and (c) and (d) AC–ZnO nanocomposite (reprinted with permission from ref. 59). | |
Sheikhshoaie and co-workers synthesized La3+/Co3O4 nanoflowers via a co-precipitation method and then used them to modify graphite screen printed electrodes for the sensitive detection of acetaminophen.60 Taei et al. reported an electrochemical sensor fabricated using an Fe2O3 (0.5)/SnO2 (0.5) nanocomposite for the concurrent detection of acetaminophen, epinephrine, and tryptophan.61 Mutharani et al. synthesized 3D stone-like copper tellurate (Cu3TeO6) via a wet chemical route and used it in an electrochemical sensor for the detection of ibuprofen.62 Zhang et al. prepared ZnO nanoflowers using the precipitation method, and then oxygen plasma treatment was applied for surface modification. They constructed an electrochemical sensor based on these ZnO nanoflowers for the detection of dopamine and diclofenac sodium.63
2.4 Green synthesis
Nowadays, there is an emerging shift for the formation of nanoparticles using green synthesis methods. The main reasons behind this emergence are safety issues, reaction complications, and the high cost of conventional methods. The green synthesis method involves plant products such as extracts and isolates. Green synthesis has a number of advantages over other methods, for example, its simplicity, effectiveness, strategy, rapid, and sustainability. In the green synthesis method, fairly homogenous nanoparticles are formed and there are no requirements for toxic chemicals, high pressure, and energy, which are the main benefits of this procedure. In some reactions, however, heating is required, which increases the production cost slightly. Zamarchi et al. fabricated a biosensor using silver nanoparticles for the detection of paracetamol. Silver nanoparticles were synthesized using silver nitrate as the precursor and pine nut extract as a stabilizing and reducing agent. The prepared Au NPs displayed a spherical morphology with an average size distribution of 91.0 ± 0.5 nm. The sensor showed a linear response towards acetaminophen from 4.98 to 33.8 μ ML−1, with a detection limit of 8.50 × 10−8 ML−1 and good reproducibility.64 Iranmanesh et al. synthesized CeO2 nanoparticles decorated with carbon nanotubes via green synthesis. They mixed CNTs (50 mg) and Ce(NO3)2·6H2O (25 mg) using a mortar and pestle, and transferred this mixture to a glass vial for irradiation using a microwave. The CNTs served as the microwave absorbing material and heating layer for decomposition of the facilitating Ce(NO3)2·6H2O. FE-SEM results revealed that the CeO2/CNT nanocomposite had been successfully prepared, and that the CNT surface was completely covered by CeO2 nanoparticles. These nanoparticles were utilized for the simultaneous determination of acetaminophen (AP), uric acid (UA), ascorbic acid (AA), and dopamine (DA) in real specimens.65 Kong et al. synthesized the rGO–TiN nanohybrid via green synthesis. They first synthesized TiO2 nanoparticles, and placed them in a horizontal alumina tube furnace before raising the temperature of the furnace from 0 to 1000 °C at a rate of about 3 °C min−1 under NH3 gas (150 mL min−1). After 6 h, they cooled the furnace to room temperature to obtain the TiN nanoparticles. Later they mixed these nanoparticles with an aqueous solution of graphene oxide and treated the resultant solution with glucose and Zn foil for 30 min at 75 °C under magnetic stirring to obtain the rGO–TiN hybrid nanostructures. They further reported, from TEM data, that the TiN nanoparticles had a cuboid shape with a size of ∼50 nm, which were densely distributed on the surface of the rGO with no free-standing TiN particles. They also found that the as-synthesized rGO–TiN nanohybrid showed an excellent electrocatalytic performance for the simultaneous detection of acetaminophen and 4-aminophenol within the range of 0.06–660 μM for acetaminophen and 0.05–520 μM for 4-aminophenol, along with low detection limits of 0.02 μM for AP and 0.013 μM for 4-aminophenol.66 Wang et al. reported the green synthesis of Pd/polyoxometalate/nitrogen-doped hollow carbon sphere tricomponent nanohybrids for the selective electrochemical detection of AP.67 Avinash et al. synthesized copper oxide nanoparticles via green synthesis using aloe vera latex as the fuel. They homogeneously mixed the desired amount of cupric nitrate and aloe vera latex, and kept the blend in a preheated muffle furnace at 400 ± 10 °C. The reaction mixture bubbled to form a transparent gel that underwent rapid combustion throughout its volume, leaving a white colored highly porous powder that was further calcined to obtain the CuO nanoparticles. TEM results displayed that the average size of the synthesized nanoparticles was 52 nm.68 Furthermore, the green synthesis of ZnO/Au nanoparticles,69 hematite/graphene nanocomposites,70 and nitrogen doped carbon dots71 were also reported.
2.5 Physical vapor deposition (PVD)
In this method, the material is deposited on a surface as a thin film or as nanoparticles. Thermal evaporation and sputtered deposition are examples of highly controlled vacuum techniques that cause the material to vaporize and then condense on a substrate. For the fabrication of thin films of various materials, physical vapor deposition techniques, such as pulsed vapor deposition, are commonly used. For pulsed laser deposition, however, laser ablation is used on a solid target, which results in the generation of a plasma of ablated species that is then deposited on a substrate to form a film. This technique is widely utilized to deposit metal nanoparticles and thin films on carbon nanotubes. It is a very easy method for the formation of thin metal films, but it has some drawbacks such as high cost and low volumes of material produced.72,73 Khoobi et al. synthesized iron oxide nanoparticles using a spray pyrolysis method, and then the prepared nanoparticles were impregnated in a carbon paste matrix to construct a modified sensor for the determination of acetaminophen.74
2.6 Sputtering techniques
This method involves the vaporization of a solid through sputtering with a beam of inert gas ions. In the past few years, it has been used for the preparation of nanoparticles using the magnetron sputtering of metal targets. Collimated beams of the nanoparticles are formed, and mass nanostructured films are deposited on the silicon substrates. The whole process is carried out at relatively low pressures (1 m Torr). Sputter deposition is executed in a vacuum chamber, in which molecules of the sputter gas enter and the working pressure is sustained. A high voltage is applied to the target (cathode), and free electrons are pushed in a spiral direction by a magnetic system, which then collide with the sputtering gas (argon) atoms, resulting in gas ionization. This continuous process generates a glow discharge (plasma) that can be used for ignition. The positively charged gas ions are attracted to impinge on the target. This occurs many times, with the ions reaching the target's surface with an energy above the surface binding energy, which enables atoms to be released from the target. Metal atoms and gas molecules continuously collide with each other in the vacuum chamber, causing atoms to scatter and form a diffuse cloud. This technique has many advantages such as lower impurities in the deposited materials, a lower cost in comparison with electron-beam lithography systems, and an unchanging composition of the sputtered material. Using this technique, alloy nanoparticles can be formed with simple control over the composition. In contrast to these benefits, it does have some drawbacks, however, where the nature of the sputtering gas (inert gasses) can affect the texture, surface morphology, composition, and optical properties of the nanocrystalline metal oxide thin films or nanoparticles.75,76 Soganci et al. prepared a single layer graphene film via a CVD method on copper foil that was then transferred to an FTO glass slide. After this, the film was decorated with copper nanoparticles via a sputtering technique. The formed sensor showed a sensitivity of 430.52 μA mM−1 cm−2 in the linear concentration range of 0.01–1.0 mM, with the detection limit of 7.2 μM.77
3. Types of electrochemical sensor
Generally, there are three types of electrochemical sensor: amperometric, potentiometric and conductometric. In amperometric sensors, the current resulting from the oxidation or reduction of an electroactive species is measured. This resulting current is produced due to the potential applied between a working and a reference electrode. By contrast, in potentiometric sensors, a local equilibrium is set-up on the interface of the sensor, where either the electrode or membrane potential is measured, and the concentration of the analyte is acquired from the potential difference between the working and reference electrodes. In conductometric sensors, the conductivity or resistivity is measured as a function of the analyte concentration.78–81
3.1 Potentiometric sensors
Since the early 1930s, potentiometric sensors have been developed for most universal practical applications. These sensors have three basic device types: ion-selective electrodes (ISEs), coated wire electrodes (CWEs), and field effect transistors (FETs). The ion selective electrode acts as an indicator electrode, which has the potential to measure selectively the activity of a specific ionic species. In the typical layout, such electrodes are commonly membrane-based devices that contain permselective ion-conducting materials, where the membrane segregates the specimen from the inside of the electrode. Within these, the first electrode is the working electrode, whose potential is decided by its environment, and the second electrode is the reference electrode, whose potential is determined by a solution that contains the analyte of interest. The potential of the reference electrode is constant and the potential difference value is connected with the concentration of the dissolved ion.82,83 Various approaches for fabricating a cathode that is particular to one species depend on the composition and nature of the membrane material. Investigations in this field have unlocked an entire arrangement of applications to an almost unlimited number of analytes, where the sole limitation is the choice of ionophore matrix of the membrane and the dopant. On the basis of the nature of the membrane, ISEs can be categorized into three classes: glass, liquid, or solid electrodes. It has been reported that there are more than two dozen ISEs that are commercially available from Corning, Orion, Beckman, Hitachi, Radiometer and many other manufacturers. They are broadly used for investigating organic ions and for cationic or anionic species from different effluents, as well as in the production and screening of drugs, utilizing selected response membrane electrodes.79
In the mid-1970s, coated-wire electrodes (CWEs) were first introduced by Freiser. In classical CWEs, a conductor is coated with a suitable ion-selective polymer membrane to make an electrode framework that is sensitive to the electrolyte concentration. The response of CWEs is nearly the same as for classical ISEs, with respect to the detectability and concentration range. Shamsipur et al. prepared a potentiometric sensor for the detection of diclofenac with a detection limit of 4.0 × 10−6 M in the concentration range from 1.0 × 10−5 to 1.0 × 10−2 M.84
3.2 Amperometric sensors
With reference to electroanalytical techniques, amperometric measurements are carried out by estimating the flow of current in the cell at a constant potential. Since the current is measured through the controlled variation of the potential, these measurements are known as voltammetry or voltammetric measurements. For both conditions, the transfer of electrons is the main operational characteristic of amperometric or voltammetric devices. The primary instrumentation needs controlled-potential equipment. The electrochemical cell contains two electrodes that are immersed in an appropriate electrolyte, although a more intricate and normal arrangement includes the use of a three-terminal cell that contains working, counter, and reference electrodes. The main reaction occurs at the working electrode. By contrast, the electrode that provides a constant potential in comparison with the working electrode is referred to as the reference electrode. Chemically inert conducting materials like graphite or platinum are utilized as the auxiliary (or counter) electrode. In controlled-potential experiments, a supporting electrolyte is needed to maintain a constant ionic strength to decrease the solution's resistance, and eliminate electromigration effects.79,85,86
The working electrode materials strongly affect the performance of amperometric sensors. Therefore, great efforts have been dedicated to the fabrication and maintenance of working electrodes. The classical electrochemical measurement of analytes started after the invention of dropping mercury electrodes by Heyrovský. In recent years, solid electrodes, fabricated using noble metals and different forms of carbon, have been found to be of great interest for the development of sensors. Effective advancements in electroanalytical chemistry have led to the development of various electrochemical sensors. For several years, mercury was a very attractive material for electrodes due to its renewable surface, extended cathodic potential range window, and high reproducibility. However, its applications were limited because of its toxicity and limited anodic potential. Alternatively, solid electrodes such as nickel, platinum, gold, carbon, and dimensionally stable anions have become very well-known as electrode materials because they are low cost and demonstrate a multifaceted potential window, chemical inertness, a low background current, and the capability for use in different sensing and detection applications. Currently, various nanomaterials are being developed for application in electrochemical sensors.87 Lima et al. fabricated an amperometric sensor based on double walled CNTs/GCE and used it for the sensing of dopamine and catechol. The sensitivity for dopamine and catechol was found to be 0.259 and 0.301 μA L μmol−1, respectively.84
3.3 Conductometric sensors
This type of sensor depends greatly on variation of the electrical conductivity of a film or bulk substance whose conductivity is influenced by the analyte present in that material. Conductometric methods are fundamentally non-specific. However, there are a few practical factors that enable conductometric methods to be unique, for example, their cost-effectiveness, simplicity (since no reference anodes are required), and insensitivity to light. Besides these factors, conductometric sensors can be miniaturized as planar interdigitated electrodes, and integrated easily through the use of thin film standard technology, which makes them inexpensive and easy to use for applications in a number of biosensors and gas sensors. Sadek and co-workers fabricated a conductometric sensor for the detection of H2 gas. They deposited doped and de-doped nanofibers onto conductometric sapphire transducers, and various concentrations of hydrogen (H2) gas at room temperature were used to determine the sensor characteristics. The sensitivity of the H2 sensor was measured to be 1.11 for doped and 1.07 for de-doped polyaniline nanofiber sensors upon exposure to 1% H2. The de-doped nanofibers exhibited better repeatability than the doped nanofibers.88
Ghosh and co-workers developed a low-cost conductometric glucose sensor, which can detect glucose concentrations as low as 10 nM.89 Sun and co-workers fabricated a conductometric sensor based on a tourmaline@ZnO core–shell structure for n-butanol gas detection. It was reported that the optimum sensitivity of the sensor was 120.8–100 ppm n-butanol at 320 °C in a 1%
tourmaline@ZnO sample, which was more than twice that of pure ZnO.90 Wang and co-workers fabricated a conductometric sensor to detect ammonia gas using titanium dioxide nanoparticle decorated black phosphorus (BP) nanosheets as the sensing layer. First, they prepared planar interdigital electrodes (IDEs) (thickness of Au/Ti layers: 200 nm/100 nm) on a SiO2/Si substrate using lithography and lift-off methods with an active area of 7 mm × 11 mm. Then they drop-cast aqueous BP (40 μL), TiO2 (40 μL), and BP–TiO2 (40 μL) solutions on different IDE devices, followed by 60 °C vacuum heating for 2 h and cooling to room temperature. The BP nanosheet electrodes decorated with titanium dioxide nanoparticles were found to be suitable to detect NH3 in the linear range of 0.5–30 ppm at RT.91
4. Some voltammetric techniques for electrochemical sensors
In analytical chemistry, voltammetric techniques are used widely for the quantitative determination of different dissolved organic and inorganic substances. In inorganic, physical, and biological chemistry, the use of voltammetric techniques is carried out for different purposes, such as electron transfer and reaction mechanisms, fundamental studies on oxidation and reduction processes, the kinetics of electron transfer processes, thermodynamic properties of solvated species, and adsorption processes on surfaces. Voltammetric techniques are also of interest for the determination of compounds in pharmaceuticals.
The various voltammetric techniques that are used are differentiated from each other by the material that is used as the working electrode and the potential function that is applied to the working electrode. Some voltammetric techniques are explained briefly in the following sections.
4.1 Linear sweep voltammetry (LSV)
In this analysis method, a linear potential is applied, which sweeps to the working electrode while the current flowing in the circuit is being simultaneously measured. A signal generator produces a voltage sweep from E to Ef, and a potentiostat applies this potential wave to the electrode under study. The direction of the scan can be positive or negative, and in principle the sweep rate can possess any constant value:
This technique is generally used in polarography under well-defined conditions; the limiting current derived from a redox process in a solution during LSV may be used to quantitatively determine the concentration of electroactive species in the solution.92 Vilian and co-workers investigated the electrochemical performance of dopamine and paracetamol using a sensor that was based on ZrO2 nanoparticles supported on graphene oxide. This prepared sensor showed two well-defined voltammetric potential peaks at 0.34 V and 0.53 V, for dopamine and paracetamol, respectively.93
4.2 Square wave voltammetry (SWV)
This voltammetric technique was invented by Ramaley and Krause, and further developed by Oster Youngs and co-workers.92 In this analysis method, remarkable versatility is found. It is a differential technique in which the potential, in the form of a symmetrical square wave of constant amplitude, is superimposed on a bare staircase potential.94 In this analysis, the graph is plotted between the difference in the current measured in forward (if) and reverse cycles (ir), versus the average potential of each waveform cycle. In this technique, the peak potential exists at the Em of the redox couple, because the current function is symmetrical around the potential. High sensitivity and excellent peak separation are the main benefits of this technique. Kang et al. detected acetaminophen in pharmaceutical preparation tablets using a graphene based sensor. They reported a recovery rate of 96.4–103.3% with a detection limit of 3.2 × 10−8 M.95
4.3 Differential pulse polarography/voltammetry (DPP/DPV)
This technique was propounded by Barker and Gardner. Using this technique, greater sensitivity, more efficient resolutions, and the differentiation of various species can be achieved. In this method, each potential pulse is constant and of a small amplitude (0.01 to 0.1), and the current is measured at two points from each pulse, one just before the application of the pulse and another at the end of the pulse. The difference between the current measurements at these points, for each pulse, is calculated and plotted against the base potential. This difference in current values reaches a maximum value near the redox potential, whereas a minimum (nearly zero) is found as the current becomes diffusion controlled.92,94 Zhang et al. prepared a sensor based on the 3D-rGO/GCE nanocomposite for the detection of an antibiotic drug. They found two reduction peaks at 170 and 633 mV with a detection limit of 0.15 μmol L−1, in the detection range from 1 to 113 μmol L−1.96 Sebastian et al. developed a sensor based on the GO/3D hierarchical ZnO nanocomposite for the detection of chloramphenicol. The electrochemical performance was observed via DPV and CV techniques. From the DPV technique, the LOD of the GO/ZnO modified GCE was found to be about 0.01 μM in the linear range of 0.2–124 μM with a sensitivity of around 7.27 μA μM−1 cm−2, and this sensor exhibited high stability, reproducibility, and repeatability.97
4.4 Anodic stripping voltammetry (ASV)
This is an electrolytic method in which mercury is kept at a negative potential to decrease the metal ions in a solution to form an amalgam with the electrode. After reducing and accumulating the analyte for a certain time, the potential on the electrode increases to re-oxidize the analyte, and in this way the current signal is generated. The current produced by anodic stripping depends on the particular type of mercury electrode, although it is directly proportional to the concentration of the analyte concentrated into the electrode.92 Using this method, Mohammed et al. determined traces of the drug timolol maleate using a Nafion/carboxylated-MWCNT nanocomposite GCE. The LOD was found to be 7.1 × 10−10 mol L−1 in the linear range from 1.0 × 10−9 to 2.0 × 10−5 mol L−1.98
4.5 Cyclic voltammetry (CV)
This method was first reported in 1938 and was described by Randles. It is the most commonly used technique for obtaining qualitative information on electrochemical reactions. The capacity of cyclic voltammetry results from its ability to rapidly offer considerable information on the thermodynamics of redox processes, couples’ chemical reactions, and the kinetics of heterogeneous electron-transfer events. It was the first experimental approach used in electroanalytical investigations, because it provides the rapid determination of redox potentials of electroactive species. CV provides both quantitative as well as qualitative information about electrochemical processes in working electrode active materials. In this method, we measure the potential between working electrode and reference electrode. On the other hand, the current is measured between counter electorde and the reference electrode. The operating stability of the electrolyte limits the potential capacity. The graph is plotted between a time-dependent current, which is obtained by scanning the potential range versus the scanned potential (E). If IdV is the integrated area under the CV curve, Vs is the potential scan rate and V is the measured potential range, then the specific capacitance Cs is given by: | 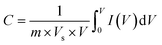 | (1) |
|  | (2) |
where C is the evaluated capacitance from eqn (1) and m is the active mass of the material.92,94 For instance, Chethana et al. investigated the oxidative behavior of diclofenac using this technique. They prepared the electrode by mixing carbon paste with tyrosine, which greatly increased the sensitivity of the CPE. The prepared sensor exhibited a satisfactory LOD of 3.28 μM and a sensitivity of 0.1905 μA μM−1.99
5. Nanomaterials based electrochemical sensing platforms for some analgesic and antipyretic drugs
5.1 Metal oxide nanomaterials
Metal oxide nanoparticles have received much consideration due to their special properties and various potential applications.100 Metal oxide nanoparticles with different morphologies have been made through versatile synthesis techniques. These metal oxide nanoparticles exhibit several types of photochemical, electrochemical, electronic, and electrical properties because of their shape, size, stability, and high surface area. Metal oxide nanoparticles exhibit remarkable qualities, particularly for noble metal nanoparticle modified electrodes, which usually show good electrocatalytic activity in the compounds with a slow redox process at unmodified electrodes.101,102 Metal oxide nanoparticles such as TiO2 nanoparticles, ZrO2 nanoparticles, Fe3O4 nanoparticles, etc., have been successfully used for the development of electrochemical sensors because of their catalytic ability, faster electron transfer kinetics and morphology.
Ozcan et al. constructed a high performance acetaminophen sensor based on zinc (Zn)/zinc oxide (ZnO) decorated reduced graphene oxide surfaces. They synthesized zinc (Zn)/zinc oxide (ZnO)/reduced graphene oxide nanohybrids via facile chemical precipitation method. Using XRD, TEM, XPS, and TGA it was confirmed that the Zn/ZnO nanoparticles were immobilized on the rGO surface with an average particle size of around 25.1 ± 6.6 nm. The response of the sensor to detect AP was found to be in the linear range of 0.05–2 mM, with a high sensitivity of 166.5 ± 6 μA mM−1 cm−2.103 Kenarkob and Pourghobadi electrochemically synthesized glassy carbon electrodes modified with ZnO/Au nanoparticles for acetaminophen sensing. The characterization results displayed that the Au nanoparticles were well anchored onto the ZnO nanospheres. The LOD was found to be 9 nM using the SWV technique in the AP concentration range of 0.05–20 μM.69
Wang et al. prepared Co/Co3O4 nanoparticles, coupled with hollow non-porous carbon polyhedron nanoparticles using pyrolysis and subsequent oxidation techniques to develop electrochemical sensors for acetaminophen detection. It was reported that pore architectures of the hollow carbon polyhedra were found to be favorable for interface features. The mesopore size and micropore size distributions of the Co/Co3O4@HNCP were found to have a pore diameter from 3 to 7 nm, and from 0.6 to 1.6 nm, respectively. The mesopore size distribution was beneficial for the mass transport and adsorption of molecules, while the micropore size distribution features possible discriminating ability of the constructed Co/Co3O4@HNCP sensor for the electrochemical sensing of AP. The constructed Co/Co3O4@HNCP sensor showed an ultrahigh sensitivity (157 μA μM−1) and a very low LOD (0.0083 μM) (Fig. 6(a)–(d)) for acetaminophen sensing in the concentration range of 0.025–2.5 μM and 2.5–50 μM, respectively.67 Sheikhshoaie and co-workers have synthesized La3+/Co3O4 nanoflowers via a co-precipitation method and used them to modify graphite screen printed electrodes for acetaminophen detection. The La3+/Co3O4 modified graphite screen printed electrode was found to be electrocatalytically active toward the detection of acetaminophen with a wide linear concentration range from 0.5 μM to 250.0 μM, and a detection limit of 0.09 μM.60 Annadurai et al. prepared nickel oxide nanoparticles using the hydrothermal route to modify a GCE for the determination of acetaminophen. CV, DPV, and amperometry were employed to investigate the electrochemical behavior of the NiO modified glassy carbon electrodes (3 mm diameter). The size of the NiO nanoparticles obtained was between 15 and 20 nm. It was found from the electrochemical studies that the sensor exhibited a linear detection range from 7.5 to 3000 mM with a high sensitivity of 91.0 μA cm−2 mM−1, and a low detection limit of 0.23 mM. The repeatability and dynamic stability of the constructed sensor is shown in Fig. 6(e) and (f), respectively.42 The electrodeposition preparation technique was adopted by Liu et al. to synthesize nickel and copper oxide nanoparticles. These prepared oxides were further used to modify graphene electrodes for the detection of dopamine, acetaminophen, and tryptophan. The modified electrode displayed linear response ranges of 0.5–20 μM, 4–400 μM, and 0.3–40 μM, and detection limits of 0.17 μM, 1.33 μM, and 0.1 μM, for detecting dopamine, acetaminophen, and tryptophan, respectively.104 Manikandan and Dharuman prepared un-doped α-Fe2O3, platinum doped Fe2O3, (dPtFe2O3), Pt decorated Fe2O3 (sPtFe2O3) and doped and decorated Fe2O3 (sdPtFe2O3) nanoparticles via a co-precipitation method in the presence of poly(ethylene glycol) and modified the surface of a glassy carbon electrode. These modified electrodes were utilized for the simultaneous detection of melatonin, dopamine, and acetaminophen. The experimental results concluded that the sdPtFe2O3 NPs had a higher catalytic activity than the other modified electrodes with dPtFe2O3, sPtFe2O3 as well as un-doped α-Fe2O3.105
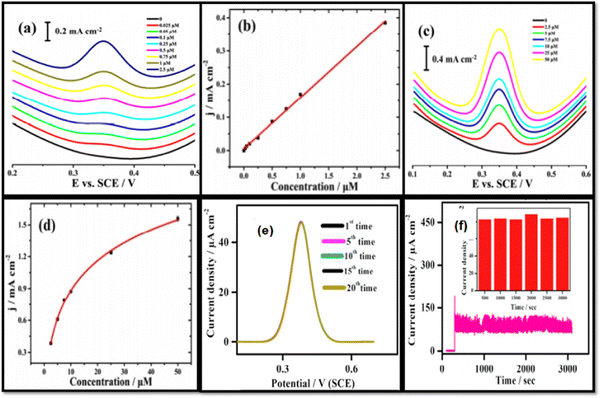 |
| Fig. 6 DPV curves for the Co/Co3O4@HNCP sensor for the sensing of AP in concentration windows of (a) 0.025–2.5 μM and (c) 2.5–50 μM. (b) Linear calibration curve and (d) logarithmic calibration curve (reprinted with permission from ref. 67). (e) Repeatability test using DPV, and (f) amperometric stability study for the NiO/GCE with 0.25 mM AP addition, where the inset shows the flow chart plot of amperometric current density vs. time (reprinted with permission from ref. 42). | |
Cao and co-workers synthesized CeBiOx nanofibers via a simple two-step procedure, which includes electrospinning and calcination with Ce
:
Bi ratios of 0.25
:
0.75, 0.5
:
0.5, and 0.75
:
0.25. SEM studies showed uniform, long, and continuous Ce0.75Bi0.25Ox NFs with an average diameter of ∼200 nm. They prepared CeBiOx nanofiber modified screen printed carbon electrodes for the detection of acetaminophen. From DPV studies, it was reported that the Ce0.75Bi0.25Ox NF modified SPE showed a linear detection range for AP between 2.5 μM and 130 μM, with a high sensitivity of 360 μA mM−1 cm−2 and a low detection limit of 0.2 μM.106 Khoobi et al. constructed a modified sensor using iron oxide nanoparticles by impregnating them in a carbon paste matrix for the detection of diclofenac. The developed sensor showed a very low detection limit of 2.45 nM in the linear range of 0.01–100.0 μM; it also exhibited long-term stability, good sensitivity, and repeatability.74 Mutharani et al. developed an electrochemical sensor based on (Cu3TeO6) for detection of the anti-inflammatory agent ibuprofen in fluids of the human body. The constructed sensor showed a detection limit of 0.017 μM and linear ranges of 0.02–5 μM and 9–246 μM, respectively. This designed sensor also demonstrated high sensitivity, good selectivity, and long term stability.62 Diouf et al. fabricated an electrochemical sensor by self-assembling chitosan capped with Au NPs on a screen-printed carbon electrode, for the detection of aspirin in tablets and human physiological fluids. The designed sensor demonstrated good selectivity, sensitivity, and reproducibility with satisfactory analytical parameters (R2 = 0.97) as well as a low LOD of about 0.03 pg mL−1 estimated from DPV.107
5.2 Carbon materials
Carbon, one of the most versatile elements found on Earth, has attracted a lot of attention due to its special types of hybridization state (sp, sp2 and sp3), which make it capable of forming a wide range of allotropes from diamond (the hardest material) to graphite (the softest material).108,109 Recently, various types of carbon based nanomaterial, such as single or multiwalled carbon nanotubes (SWCNTs or MWCNTs, respectively),110 carbon nanostructures, graphene,111 graphene oxide,112 and reduced graphene oxide,113 have been synthesized, which have gained attention due to their physical, optical, magnetic, and super electronic properties; these make carbon suitable for wide applications in sensing devices,114 energy storage,115 and drug delivery.116
Various studies have confirmed the use of carbon-based materials for the detection of acetaminophen. Cernat and co-workers reported, in their review article, that different configurations of carbon, such as modified/unmodified carbon nanotubes and graphene, could become a good candidate for electrochemical sensors and biosensors for the detection of acetaminophen.117 Gopal and co-workers fabricated eco-friendly and bio-waste-based hydroxyapatite/rGO hybrid modified carbon paste electrodes for the simultaneous detection of dopamine, acetaminophen, and ascorbic acid. They used eggshell bio-waste-based hydroxyapatite materials for the sensing applications. The electrochemical performance results showed a good linear range for the detection of acetaminophen from 20 to 160 μM.118 Pham and co-workers prepared platinum decorated rGO modified glassy carbon electrodes via an environmentally friendly electrodeposition technique for the detection of acetaminophen. Morphology characterization techniques revealed the cauliflower-like structure of the Pt particles. The prepared electrochemical sensor was able to detect acetaminophen in a linear concentration range from 0.01 to 350 μM, with a detection limit of 2.2 nM.119
Alam and co-workers coated a glassy carbon electrode using a drop cast method with multi-walled carbon nanotube–β-cyclodextrin composites for the low level detection of acetaminophen in water. It was reported that the sensor responded to acetaminophen in a linear range of 50 nM–300 μM with a detection limit of 11.5 nM, and showed good reproducibility, high stability, and exclusive selectivity. They also mentioned that this improvement was because of the electron transfer capability and high conductivity of the MWCNTs, which also had a high surface-to-volume ratio, and that the higher surface area of the sensor was due to the porous structure of the CD.110 Berto et al. designed and tested an electro-activated glassy-carbon electrode for acetaminophen detection in surface water. The sensor showed a linear range of 13.3–33 μg L−1 for acetaminophen, and was able to detect acetaminophen concentrations higher than 4.4 μg L−1 in untreated samples.120
Liang and co-workers developed a sensor based on glassy carbon electrodes modified with nitrogen-rich porous carbon for acetaminophen detection. They synthesized nitrogen rich porous carbon nanotubes via assisted carbonization of the zeolitic imidazolate framework ZIF-8 using polyvinylpyrrolidone. They found from TEM studies that the average size of the ZIF-8 polyhedra was 70 nm. The pore size of P-NC was found to be distributed in the 4–4.5 and 30–50 nm range, which confirmed the efficient preparation of a meso–microporous hierarchical structure. According to them, the highly porous structure of the prepared electrodes provided an interweaving network that facilitated more active sites and helped the better transportation of reactants and products, which contributed to the better electrochemical performance. The sensor showed good linearity for acetaminophen in the range of 3–110 μM, with a minimum LOD of ∼0.5 μM (S/N ≈ 3), and the sensor was efficiently applied for the detection of acetaminophen in urine samples. Fig. 7(a) displays the DPV voltammograms, and Fig. 7(b) shows the calibration plot of the synthesized sample.121
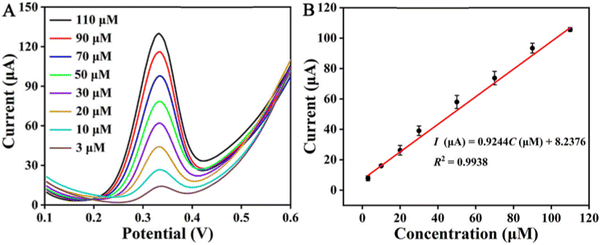 |
| Fig. 7 (A) DPV curves of AP at different concentrations on the P-NC/GCE in 0.1 M PBS (pH 7.0), and (B) calibration plot of peak current as a function of the AP concentration (reprinted with permission from ref. 121). | |
Amiri et al. reported carbon nanoparticle modified carbon paste electrodes for the detection of paracetamol, phenylephrine, and dextromethorphan. The paste design consisted of a hydrophobic binder, hydrophobic graphite as the conducting component, and a nanoparticulate thin film with a hydrophilic surface to provide sensitivity and selectivity. The sensor was found to be suitable for the detection of acetaminophen in the linear range of 1 × 10−7 M to 1.0 × 10−3 M, with a detection limit of 1.5 × 10−8 M.9
Tsierkezos studied the effect of the incorporation of nitrogen on the electrocatalytic activity of MWCNTs. They synthesized vertically aligned MWCNTs on an oxidized porous silicon wafer using a catalytic CVD technique with acetonitrile as the carbon source material and ferrocene as the catalyst. The solution of ferrocene in acetonitrile (1% w/w) was introduced into a furnace at 900 °C through a syringe with a flow rate of 0.2 ml min−1 to fabricated the nitrogen-doped multiwalled carbon nanotubes. The modified carbon nanotubes were utilized to develop the electrochemical sensor for acetaminophen sensing. It was noted that the sensor detection ability was enhanced by the nitrogen doping in the CNTs. The sensor based on pristine MWCNTs recorded a very low sensitivity (0.6010 A M−1 cm−2) and detection limit (0.950 μM), but the nitrogen doped MWCNT sensor's detection limit and sensitivity increased significantly to 0.485 μM and 0.8406 A M−1 cm−2, respectively.122 Barsan and co-workers developed and characterized acetaminophen sensors in two different architectures. In the first configuration, they electropolymerized PMG onto a graphite–epoxy composite electrode (CE) and then coated these electrodes with fCNTs (fCNT/PMG/CE); in the second architecture they coated the CE with fCNTs and then electropolymerized PMG onto these electrodes (PMG/fCNT/CE). It was observed that on the fCNT/CE, the polymer was better formed compared with the bare CE because of the higher surface area of the fCNTs. The sensors were used to detect pyridoxine and acetaminophen, and it was observed that the fCNT-PMG-CE possessed a higher sensitivity than the PMG-fCNT-CE.123 Li and co-workers described the fabrication of layer-by-layer (LBL) carboxylic acid functionalized multiwalled CNTs on glassy carbon electrodes to develop an electrochemical sensor for paracetamol (acetaminophen). The covalent LBL assembly was confirmed using SEM. It was reported that the modified electrode with six layers exhibited a good sensitivity of 2.293 μA M−1 cm−2 in the linear range of 1–200 μM with a detection limit 0.092 μM.93 Sarhangzadeh et al. constructed a sensor for the simultaneous detection of diclofenac and indomethacin using MWCNTs and ionic liquid modified carbon ceramic electrodes. Using the DPV technique, the prepared electrode showed linear calibration curves in the concentration range of 0.05–50 μmol L−1 for diclofenac and 1–50 μmol L−1 for indomethacin. The developed sensor showed a limit of detection of 18 nM for diclofenac and 260 nM for indomethacin.124 Roushani et al. fabricated an ultrasensitive sensor using gold nanoparticles that were electrochemically deposited on a glassy carbon electrode surface for the detection of ibuprofen in spiked human serum. In this study, it was observed that a layer of Au nanoparticles can improve the electrochemical performance as well as the electron transfer due to the layer's large surface area. The designed sensor demonstrated good reproducibility and long-term stability. The detection limit was found to be 0.5 pM in the concentration range of 0.005–7 nmol−1.125 Suresh et al. simultaneously detected paracetamol and ibuprofen in different tablets using glassy carbon electrodes with stripping SWV and DPV. The constructed sensor showed good repeatability and recorded a detection limit (LOD) of 0.96
μmol L−1 in a linear concentration range between 1.45 and 3.87
μmol L−1. It was reported that this type of sensor can be successfully used for both drugs (AP and IB) in commercial tablets. These obtained data were found to be in agreement with the data of many manufacturing companies.126
5.3 Nanocomposites
Nanocomposites have the properties of their components plus a new characteristic due to the synergistic effect. Because of their new features, nanocomposites are widely employed in electrochemical sensors to detect different drugs. Various investigations have been done on nanocomposite based electrochemical sensors for the detection of different drugs. Hao et al. synthesized copper nanowires and used them to prepare a Cu nanowire/graphene oxide nanocomposite (Cu-NW/GO). Later they modified glassy carbon electrodes using this Cu-NW/GO nanocomposite for the simultaneous detection of acetaminophen, dopamine, and ascorbic acid. They reported that the sensor presented a wide linear range of 1–60 μM, 1–100 μM, and 1–100 μM with a detection limit of 50, 410, and 40 nM, for ascorbic acid, dopamine and acetaminophen, respectively.127 Hasanpour et al. prepared a semiconductor composite CuO/CuFe2O4 with a p–n junction. The prepared nanocomposite was to make carbon paste electrodes for the detection of acetaminophen and codeine in biological fluids. The electrode surface area of CuO/CuFe2O4/CPE was observed to be 0.85 cm2, which was 5.21 times greater than the surface area of the unmodified carbon paste electrode. It was reported that the CuO/CuFe2O4/CPE electrode was found to be suitable for acetaminophen detection with a very low detection limit of 0.007 μmol L−1 in the linear range of 0.01–1.5 μmol L−1.128 Lin et al. synthesized a graphene/ZrO2 nanocomposite to modify screen printed carbon electrodes (SPCEs), and it also studied their sensing properties for acetaminophen detection. The graphene/ZrO2 modified screen printed carbon electrodes showed an acetaminophen detection limit of 75.5 nM, for the linear range of 10–100 μM.129 Tamilalagan et al. fabricated the (Ni/Zn)O@rGO p–n heterojunction semiconductor nanocomposite, which was used for acetaminophen detection using the DPV technique. The DPV response curve showed a linear relationship between the AP concentration and the anodic current. This excellent response behavior of the synthesized nanocomposite was observed with a detection limit of 2.2 nM over a wider range from 0.009 to 413 μM. Moreover, the (Ni/Zn)O@rGO/GCE exhibited a high sensitivity of 19.1 μA μM−1 cm−2. This might occur because of the larger surface area of the prepared nanocomposite, where nano-sized spherical particles were found to be decorated on the rGO sheets. It was also noticed that the (Ni/Zn)O@rGO modified GCE demonstrated very good selectivity towards acetaminophen with good repeatability and reproducibility.130 Nikpanje et al. developed an electrochemical sensor based on a carbon paste electrode (CPE), modified with ZnO–Zn2SnO4–SnO2 and graphene (ZnO–Zn2SnO4–SnO2/Gr/CPE), for the detection of acetaminophen, ascorbic acid, and caffeine. It was shown that the modified electrodes exhibited a high electrical conductivity, which made them a good candidate for electrochemical applications. The amperometric response of the electrodes confirmed the detection limits to be 0.00364, 0.00385, and 0.00628 μM for acetaminophen, caffeine, and ascorbic acid in the linear range of 0.008–12 μM, 0.01–14 μM, and 0.013–16 μM, respectively. In addition, the ZnO–Zn2SnO4–SnO2/Gr/CPE based sensor showed superb selectivity, repeatability, stability, and reproducibility for the determination of acetaminophen, ascorbic acid, and caffeine.131 Iranmanesh et al. modified a glassy carbon electrode with the CeO2–CNT nanocomposite and used it for the simultaneous detection of ascorbic acid (AA), dopamine (DA), uric acid (UA), and acetaminophen (AP). The detection limits for AA, DA, UA and AP were found to be 3.1 nM, 2.6 nM, 2.4 nM, and 4.4 nM in the linear range of 0.01–900.0 μM, 0.01–700.0 μM, 0.01–900.0 μM, and 0.01–900.0 μM, respectively.65
Afkhami and co-workers developed a novel electrochemical sensor based on a carbon paste electrode modified with the NiFe2O4/graphene nanocomposite for the effective and simultaneous detection of acetaminophen and tramadol. The morphology and electronic composition of the prepared NiFe2O4/graphene nanocomposite was confirmed using SEM, XRD, and FT-IR spectroscopy. The limit of detection for acetaminophen and tramadol was confirmed to be 0.0036 and 0.0030 μmol L−1, respectively. It was found that the sensitivity of the sensor was enhanced by the combination of graphene and NiFe2O4 in the nanocomposite. The fabricated sensor possessed high sensitivity and good stability for clinical assays of tramadol and acetaminophen.132 Demir and co-workers designed an electrochemical sensor based on a screen printed electrode modified with a molybdenum disulphide–titanium dioxide/reduced graphene oxide (MoS2–TiO2/rGO) nanocomposite for paracetamol detection. The MoS2–TiO2/rGO/SPE sensor was examined for the effect of the rGO
:
MoS2–TiO2 ratio, and the amount of MoS2–TiO2/rGO composite on the screen-printed electrode for the sensitive and selective detection of paracetamol. It was reported that the sensor showed a high electrocatalytic performance for the oxidation of acetaminophen with a low detection limit of 0.046 μM and a wide linear response in the range of 0.1–125 μM. The sensor was also used for the detection of acetaminophen in urine and drug samples with acceptable recovery values.133 Anuar et al. fabricated modified glassy carbon electrodes with a platinum nitrogen-doped graphene (Pt/NGr) nanocomposite for the sensitive determination of acetaminophen. From TEM images obtained they reported that the Pt nanoparticles were evenly distributed on the NGr sheets. It was also reported that the synergy between the nitrogen-doped graphene and the platinum nanoparticles enhanced the interfacial electron transfer process and showed a higher catalytic performance towards the electrochemical oxidation of acetaminophen. The sensor was found to be suitable for the detection of acetaminophen in a linear range of 0.05–90 μmol L−1 with a detection limit of 0.008 μmol L−1. FESEM images for this sensor are shown in Fig. 8(a) and (b), while Fig. 8(c) and (d) represent the electrochemical results of the as-prepared sensor.134
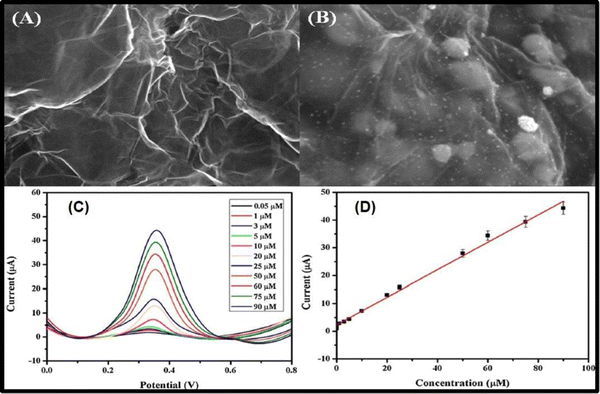 |
| Fig. 8 FESEM images of (A) NGr, and (B) NGr with Pt NPs deposited on it; (C) square-wave voltammograms with different AP concentrations at the Pt/NGr/GCE in 0.1 mol L−1 phosphate buffer solution at pH 7.0 and (D) plot of peak current against concentration of AP (reprinted with permission from ref. 134). | |
Shaikshavali et al. fabricated an electrochemical sensor based on MWCNTs decorated with a CuO–Au composite for the sensitive determination of acetaminophen and 4-aminophenol. It was reported that the electrochemical activity of the CuO–Au/MWCNTs/GCE was higher than the bare GCE, the CuO–Au/GCE and the MWCNTs/GCE. A good linear response of 0.2–6.0 μM and 0.5–1.6 μM with a detection limit of 0.016 μM and 0.105 μM was recorded for acetaminophen and aminophenol, respectively.135 Huang and co-workers reported that modified glassy carbon electrodes with layered MoS2–graphene composites could be used for acetaminophen detection in the linear range of 0.1–100 μM with a detection limit of 2.0 × 10−8 M. The superior electrochemical performance resulted because of the robust composite structure and the synergistic effects between the layered MoS2 and graphene.136 Kimuam et al. prepared a nanocomposite of platinum nanoflowers and reduced graphene oxide (PtNFs/rGO), which was used for the determination of diclofenac in urine samples. Using the DPV technique, a linear range of 0.1–100 μM was observed, with a detection limit of 40 nM. The recovery rate was found to be in the range of 85–100%; they proposed that this system might be used in various applications.137 Goyal et al. used an SWCNT modified pyrolytic graphite electrode for the determination of diclofenac. At pH 7.2, diclofenac oxidized at 439 mV and 854 mV. It was observed that the modified electrode demonstrated an excellent catalytic activity compared with the bare electrode. The calibration curves were found to be linear in the concentration ranges of 1 × 10−9 to 500 × 10−9 M and 25 × 10−9 to 1500 × 10−9 M for peaks I and II, respectively. In this investigation, they used the SWV technique to determine diclofenac in biological and pharmaceutical samples.138 Nasiri et al. constructed a sensor using a graphene oxide/CNT nanocomposite and gold nanoparticles for the determination of diclofenac molecules. They used an electrochemical deposition method to deposit the AuNPs on the surface of MWCNT–GO nanocomposite films. The developed sensor showed good results which may be attributed to the large surface area of the prepared nanocomposite and the fast electron transfer rate of the AuNPs. The detection limit was found to be 0.09 μmol L−1 in the linear range of 0.4–1000 μmol L−1.139
Charithra et al. fabricated poly asparagine modified CNT and graphene mixed paste electrode for the detection of acetaminophen. The prepared electrode showed a very low detection limit of 4.10 × 10−8 M in the linear range of 20–100 μM.140 Chen et al. attached CuO, Cu2O, and CuS, respectively, on g-C3N4 to prepare composites, and used these composite electrodes for acetaminophen detection. Fig. 9(a) shows the detection of acetaminophen using these synthesized nanocomposites. It was found that the Cu2O/g-C3N4, CuO/g-C3N4, and CuS/g-C3N4 sensors have wide linear ranges of 5–250 μM with a LOD of 0.47 μM (for Cu2O/g-C3N4), 5–300 μM with a LOD of 0.32 μM (for CuO/g-C3N4), and 5–500 μM with a LOD of 0.26 μM (for CuS/g-C3N4), as shown in Fig. 9(b)–(d), respectively.36 Shi et al. prepared a nanocomposite containing zinc tetrahydroxyphthalocyanine and reduced graphene oxide (rGO–ZnPc–OH) as an electrode material for acetaminophen sensing in human urine samples and drug formulations. It was observed that the formed nanocomposite provided an effective electroactive surface area. A synergistic enrichment was also seen because of the adsorption of π–π stacking and hydrogen bonding of hydroxyl groups.141 Yigit et al. fabricated a graphene–Nafion composite film on a GCE; they used it for the simultaneous detection of acetaminophen, aspirin and caffeine in commercial tablets. The CV and ASV techniques were used to determine the electrochemical behavior of all the mentioned drugs. The oxidation peaks of the designed electrochemical sensor were observed at 0.64, 1.04 and 1.44 V, and good linear current responses were also demonstrated, with detection limits of 1.2 × 10−9 M, 6.5 × 10−8 M and 3.8 × 10−8 M for acetaminophen, aspirin and caffeine, respectively.142 Roushani et al. fabricated a low-cost electrochemical aptasensor for the detection of ibuprofen. They formed a nanocomposite with nitrogen doped GQDs and gold nanoparticles which have a unique matrix for covalently attaching the aptamer molecules. The modified GCE with prepared nanocomposite provided higher surface area and electrical conductivity. In this study, riboflavin was first used for the electrochemical detection of ibuprofen. The detection limit was found to be 33.33 aM. The obtained results revealed that this type of strategy can be implicated in the design of biosensors and electrochemical sensors for the detection of different targets.143 The literature survey mentioned above is summarized in Table 1.
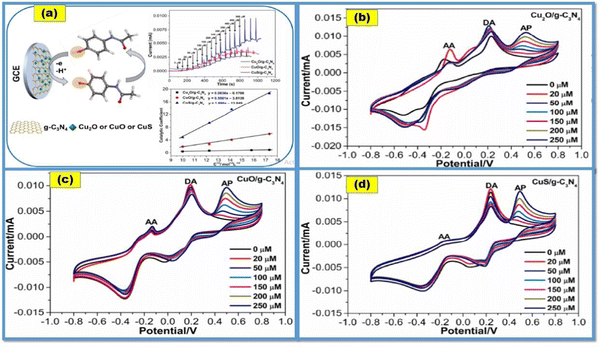 |
| Fig. 9 (a) Detection of AP using CuX/g-C3N4 nanocomposite-based electrochemical sensors (CuX = Cu2O, CuO and CuS); and (b)–(d) CV curves for paracetamol (AP) on different CuX-C3N4 modified electrodes in the presence of dopamine (DA) and ascorbic acid (AA) (reprinted with permission from ref. 36). | |
Table 1 Comparison data for nanomaterial based electrochemical sensors for the detection of drugs
Material |
Synthesis method |
Morphology |
Detection limit |
Linear range |
Drug |
Ref. |
MWCNTs |
Layer by layer |
— |
0.5 μM (5 × 10−7 mol L−1) |
25–400 μM |
AP |
144
|
f-MWCNTs modified glassy carbon electrodes (GCEs) |
— |
Densely packed |
0.6 μM (0.6 μmol L−1) |
3–300 μM (3–300 μmol L−1) |
AP |
145
|
SWCNT–graphene nanosheet hybrid film |
Modified Hummers' method |
Uniformly dispersed |
38 × 10−3 μM (38 nM) |
0.05–64.5 μM |
AP |
146
|
Iron oxide NPs |
|
|
2.45 × 10−3 μM (2.45 nM) |
0.01–100.0 μM |
DCF |
74
|
Cu3TeO6 |
Wet chemical route |
Stone like |
0.017 μM |
0.02–5 μM |
IB |
62
|
Nafion/TiO2–graphene nanocomposite |
— |
— |
0.21 μM (2.1 × 10−7 M) |
1–100 μM |
AP |
147
|
Fe3O4@Au–S–Fc/GS |
Co-precipitation |
|
0.05 μM |
|
AP |
148
|
MWNTs |
Molecular imprinting and sol–gel |
3D network |
0.04 μM (4.0 × 10−8 mol L−1) |
8.0 × 10−2 to 5.0 × 1011 μM |
AP |
53
|
(8.0 × 10−8 to 5.0 × 105 mol L−1) |
ZnO |
|
Nanoflowers |
|
0.1–300 μM |
DA |
63
|
GO/MIPs |
Modified Hummers' method |
Curved and layer like structure |
20 × 10−3 μM (20 nM) |
0.1–80 μM |
AP |
55
|
Fe2O3(0.5)/SnO2(0.5) |
Solid phase reaction |
— |
0.2 μM (0.2 μmol L−1) |
4.5–876.0 μM (4.5–876.0 μmol L−1) |
AP |
61
|
MoS2-Gr/GCE |
Solution-phase method |
3D sphere-like |
0.02 μM |
0.1–100 μM |
AP |
136
|
rGO–tin nanohybrid |
Green synthesis |
Wrinkled and flake like |
0.02 μM |
0.06–660 μM |
AP |
66
|
Graphene–Nafion composite film |
|
|
6.5 × 10−8 |
|
ASP |
142
|
Graphene/SnO2 nanocomposite |
Hydrothermal method |
Irregular |
1 μM |
|
AP |
43
|
ERG/GCE |
— |
— |
0.0021/1.2 μM |
0.005–4/5–800 μM |
AP |
111
|
Activated carbon–ZnO composite |
Co-precipitation |
Nanoflakes |
0.02 μM (0.02 μ ML−1) |
0.05–1380 μM (0.05–1380 μmol L−1) |
AP |
59
|
Cd(OH)2–rGO |
Co-precipitation |
Nanorod-like |
0.08 μM |
0.1–102 μM |
AP |
149
|
CuO–CuFe2O4 |
Co-precipitation |
Spherical |
0.007 μM (0.007 μmol L−1) |
0.01–1.5 μM (0.01–1.5 μmol L−1) |
AP |
128
|
γ-Fe2O3 |
Co-precipitation |
— |
75 μM (0.075 mM) |
31–1000 μM (3.1 × 10−5 M to 1.0 × 10−3 M) |
AP |
150
|
P-RGO |
Hydrothermal method |
— |
0.36 μM |
1.5–120 μM |
AP |
44
|
Graphene/ZrO2 |
Green synthesis |
Sheets |
75.5 × 10−3 μM (75.5 nM) |
10–100 μM |
AP |
129
|
Chitosan–Au NPs |
Chemical route |
Dispersed |
0.03 pg mL−1 |
— |
ASP |
107
|
Pd/POMs/NHCSs |
Green synthesis |
Nanospheres |
3 × 10−3 μM (3 nM) |
0.63 μM to 0.083 mM |
AP |
151
|
La3+/Co3O4 |
Co-precipitation |
Nanoflower |
0.09 μM |
0.5–250.0 μM |
AP |
60
|
Sl-CPE |
— |
— |
21 × 10−3 μM (21 nm) |
1–160 μM |
AP |
152
|
Ag NPs/GCE |
Green synthesis |
— |
8.50 × 10−2 μM (8.50 × 10−8 mol L−1) |
— |
AP |
64
|
NiO/GCE |
Hydrothermal method |
Asymmetric |
0.23 μM |
7.5–3000 μM |
AP |
42
|
CeO2–CNT |
Green synthesis |
|
4.4 × 10−3 μM (4.4 nM) |
0.01–900.0 μM |
AP |
65
|
rGO/AuNPs/MWCNTs |
Chemical route |
Tubular/flaky |
42 × 10−3 μM (42 nM) |
0.12–12 μM |
AP |
153
|
Cu2O–CuO/rGO/CPE |
Hummers’ method/chemical reduction |
— |
0.003 μM |
0.008–13 μM |
AP |
154
|
SrP/g-CN NSs |
Thermal polymerization technique |
Nanosheets |
2 × 10−3 μM (2.0 nM) |
0.01–370 μM |
AP |
155
|
Ni/C-400/GCE |
Direct calcination method |
Spherical |
4.04 × 10−2 μM |
0.20–53.75 μM |
AP |
156
|
GCE |
— |
— |
0.96 μM |
1.45–3.87 μmol L−1 |
IB |
126
|
PASMCNTMGPE |
— |
Thick layer |
0.04 μM |
20–100 μM |
AP |
140
|
BC/Co3O4/FeCo2O4 |
Calcination treatment with hydrothermal |
Nanosheets |
0.02886 μM |
0.1–220 μM |
AP |
47
|
rGO–ZnPc–OH nanocomposite |
Hummers' method/chemical route |
Gauzy crinkled and folded nanosheets |
10−2 μM (10 nM) |
100–800 μM |
AP |
141
|
(WP6–Pd–COF) nanocomposite |
Chemical route |
— |
3 × 104 μM (0.03 M) |
0.1–7.5 μM |
AP |
157
|
6. Challenges and future prospects
Over the past few years, there has been a significant increase in the need for precise and affordable methods for the detection and quality control of pharmaceutical compounds. As a result, there has been a lot of interest in and the development of specialized nanomaterial-based electrochemical sensors. For full utilization of the capabilities of advanced electrochemical sensors, a thorough understanding of the physicochemical and electronic interactions that take place at the interfaces between nanomaterials and relevant analytes is required. In this review, the most recent developments in the creation of electrochemical sensors based on nanomaterials for the detection of important pharmaceutical drugs such as acetaminophen, ibuprofen, aminophenol, diclofenac, dopamine, and others are covered in-depth. Noteworthy progress has been made in combining carbon-based nanomaterials with metal nanoparticles, quantum dots, organic functional groups, and conductive polymers to produce powerful synergistic effects that will make it easier to catalyze reactions with target analytes. Although the sensitivity and LOD are often improved with these methods, other problems, such as fouling and the non-specific adsorption of other species, can occur that make it more challenging to commercialize the proposed electrochemical sensors. In fact, few researchers have questioned whether adding carbon-based nanomaterials like graphene, GO, and CNTs has a synergistic effect on the electrochemical detection of analytes. It was not always confirmed that carbon nanomaterials or the modified materials themselves would produce a signal that was comparable. Moreover, the improved performance observed after using carbon nanomaterials may simply be the result of an increase in the active surface area. For tailoring the design of sensing platforms, a thorough understanding of the electrochemical reactions at electrode/electrolyte interfaces remains one of the foremost challenges. Despite the fact that most proposed electrochemical sensors have been tested with real samples, there remains a significant gap between laboratory tests and the commercialization of these sensing devices. To develop and commercialize electrochemical sensors and biosensors for the efficient detection of pharmaceutical drugs, the industry, as well as multidisciplinary research groups, must collaborate closely. Electrochemical sensors and biosensors must be carefully analyzed with regard to their costs and stability in addition to their sensitivity and selectivity. For the analysis of pharmaceutical compounds, chromatographic techniques such as HPLC, GC–MS, and LC–MS/MS as well as colorimetric methods are currently widely employed in hospitals and laboratories. However, these techniques are often constrained in terms of their portability. Electrochemical sensors, on the other hand, have advantages that allow them to surpass the limitations of those more traditional methods. Using liquid chromatography or mass spectrometry in conjunction with electrochemical sensing for effective drug detection is one of the newest trends. Over the past few decades, remarkable work has been carried out in the advancement of sophisticated electrochemical sensors and biosensors for the sensing of pharmaceutical compounds. An encouraging trend for further improvement in the sensitivity and a reduction in detection limits for target analytes through synergistic effects is via the use of nanocomposites made of carbon and other nanomaterials like metal nanoparticles, polymers, and functionalized nanostructures.
7. Conclusions
This review summarizes recent advances in the fabrication of electrochemical sensors used for analgesic and antipyretic drug detection. We have discussed some commonly used drugs such as acetaminophen, ibuprofen, aspirin, and diclofenac. We deliberately discussed synthesis methods for nanomaterials and their utilization in electrochemical sensors for the detection of drugs. Generally, the special properties of metals and carbon-based nanomaterials have contributed considerably to the advancement of electrochemical sensors. Both the novel and adjusted metal-based probes often show an improved analytical performance over traditional non-nanostructured electrochemical frameworks. Electroanalytical techniques that utilize sensing and biosensing devices, including carbon and metal oxide-based nanostructure modified electrodes, are promising for real-life analytical detection applications. Specifically, diamonds and CNTs have been used as electrode materials for electrochemical sensing. However, a few challenges remain, for instance, the scalability and reproducibility of current “nano” gadgets, and the sensing frameworks are particularly influenced by the properties of the nanostructures that are utilized. However, greater suitable assessments of the few performance properties, including their application for detecting analytes in real world samples, are essential before potential commercialization.
Abbreviations
AP | Acetaminophen |
IB | Ibuprofen |
DCF | Diclofenac |
ASP | Aspirin |
NSAID | Non-steroidal anti-inflammatory drug |
NPs | Nanoparticles |
CV | Cyclic voltammetry |
ASV | Anodic stripping voltammetry |
LSV | Linear sweep voltammetry |
SWV | Square wave voltammetry |
DPV | Differential pulse voltammetry |
AA | Ascorbic acid |
DA | Dopamine |
CNTs | Carbon nanotubes |
SWCNTs | Single walled carbon nanotubes |
MWCNTs | Multiwalled carbon nanotubes |
RT | Room temperature |
LOD | Limit of detection |
SEM | Scanning electron microscope |
FESEM | Field emission electron microscopy |
TEM | Transmission electron microscopy |
XRD | X-ray diffraction |
FTIR | Fourier transform infrared radiation |
CVD | Chemical vapor deposition |
GO | Graphene oxide |
rGO | reduced graphene oxide |
GCE | Glassy carbon electrode |
Ag | Silver |
Au | Gold |
GQDs | Graphene quantum dots |
UA | Uric acid |
NiO | Nickel oxide |
ZnO | Zinc oxide |
CA | Chronoamperometry |
Author contributions
Manika Chaudhary: role/writing – original draft, data curation, validation; Ashwani Kumar: review editing and software; Arti Devi: role/writing – original draft, investigation; Beer Pal Singh: supervision, resources, conceptualization; Bansi Dhar Malhotra: supervision, writing – review and editing; Kushagr Singhal: review, editing, Sangeeta Shukla: resources, project administration; Carmen A Vega-Olivencia: conceptualization, Srikanth Ponnada: formal analysis and review editing; Rakesh K. Sharma: supervision and review editing, Shrestha Tyagi: software; Rahul Singhal: writing – review and editing, conceptualization, visualization, methodology, supervision.
Conflicts of interest
The authors declare that they have no known competing financial interests or personal relationships.
Acknowledgements
One of us (BDM) Acknowledges the Science and Engineering Board (DST-SERB, Govt. of India) for the award of Distinguished Fellowship (SB/DF/011/2019).
References
-
H. H. Brownstein, Drugs and Society, The Handbook of Drugs and Society, 2015. pp.1–13 Search PubMed.
- E. Jane-Llopis and I. Matytsina, Mental health and alcohol, drugs and tobacco: a review of the comorbidity between mental disorders and the use of alcohol, tobacco and illicit drugs, Drug Alcohol Rev., 2006, 25(6), 515–536, DOI:10.1080/09595230600944461.
- J. van Amsterdam, A. Opperhuizen, M. Koeter and W. van den Brink, Ranking the harm of alcohol, tobacco and illicit drugs for the individual and the population, Eur. Addict. Res., 2010, 16(4), 202–207, DOI:10.1159/000317249.
-
J. Peppin, K. Dineen, A. Ruggles and J. Coleman, Prescription Drug Diversion and Pain: History, Policy, and Treatment, Oxford University Press, 2018 Search PubMed.
-
S. Agnieszka, Global Pharmaceutical Industry: Characteristics and Trends, in Global Supply Chains in the Pharmaceutical Industry, ed. N. Hamed and S. Agnieszka, IGI Global, Hershey, PA, USA, 2019, pp.57–85 Search PubMed.
- B. Lunenfeld and P. Stratton, The clinical consequences of an aging world and preventive strategies, Best Pract. Res. Clin. Obstet. Gynaecol., 2013, 27(5), 643–659, DOI:10.1016/j.bpobgyn.2013.02.005.
- A. Kaushik and E. Mostafavi, To manage long COVID by selective SARS-CoV-2 infection biosensing, Innovation, 2022, 3(5), 100303, DOI:10.1016/j.xinn.2022.100303.
- M. Keyvanfard, R. Shakeri, H. Karimi-Maleh and K. Alizad, Highly selective and sensitive voltammetric sensor based on modified multiwall carbon nanotube paste electrode for simultaneous determination of ascorbic acid, acetaminophen and tryptophan, Mater. Sci. Eng. C, 2013, 33(2), 811–816, DOI:10.1016/j.msec.2012.11.005.
- M. Amiri, F. Rezapour and A. Bezaatpour, Hydrophilic carbon nanoparticulates at the surface of carbon paste electrode improve determination of paracetamol, phenylephrine and dextromethorphan, J. Electroanal. Chem., 2014, 735, 10–18, DOI:10.1016/j.jelechem.2014.10.006.
- J. Li, J. Liu, G. Tan, J. Jiang, S. Peng, M. Deng, D. Qian, Y. Feng and Y. Liu, High-sensitivity paracetamol sensor based on Pd/graphene oxide nanocomposite as an enhanced electrochemical sensing platform, Biosens. Bioelectron., 2014, 54, 468–475, DOI:10.1016/j.bios.2013.11.001.
- M. T. Olaleye and B. T. J. Rocha, Acetaminophen-induced liver damage in mice: Effects of some medicinal plants on the oxidative defense system, Exp. Toxicol. Pathol., 2008, 59(5), 319–327, DOI:10.1016/j.etp.2007.10.003.
- X. Wu, Y. Wu, H. Dong, J. Zhao, C. Wang, S. Zhou, J. Lu, Y. Yan and H. Li, Accelerating the design of molecularly imprinted nanocomposite membranes modified by Au@ polyaniline for selective enrichment and separation of ibuprofen, Appl. Surf. Sci., 2018, 428, 555–565, DOI:10.1016/j.apsusc.2017.09.104.
- C. Parlak and Ö. Alver, Adsorption of ibuprofen on silicon decorated fullerenes and single walled carbon nanotubes: A comparative DFT study, J. Mol. Struct., 2019, 1184, 110–113, DOI:10.1016/j.molstruc.2019.02.023.
- Ş. Dinç-Zor and Ö. Aksu Dönmez, Box-Behnken Design-Desirability Function Approach in Optimization of HPLC Method for Simultaneous Determination of Ibuprofen Along with Additives in Syrup Formulation, J. AOAC Int., 2021, 104(1), 78–83, DOI:10.1093/jaoacint/qsaa096.
- E.-S. Mahmoud, A. Omar, A. M. Bayoumy and M. Ibrahim, Chitosan ibuprofen interaction: modeling approach, Sens. Lett., 2018, 16(5), 347–355, DOI:10.1166/sl.2018.3956.
- C. Cao, R. Jin, H. Wei, Z. Liu, S. Ni, G.-J. Liu, H. A. Young, X. Chen and G. Liu, Adaptive in vivo device for theranostics of inflammation: Real-time monitoring of interferon-γ and aspirin, Acta Biomater., 2020, 101, 372–383, DOI:10.1016/j.actbio.2019.10.021.
- X.-J. Han, X.-F. Ji, Q. Zhang, J.-W. Sun, P.-X. Sun, W.-J. Pan, J. Wang and C. Yang, Giant “molecular capacitor” arrays - portable sensors to determine ionizable compounds, J. Electroanal. Chem., 2020, 865, 114108, DOI:10.1016/j.jelechem.2020.114108.
- F. M. Abdel-Haleem and E. M. Zahran, Miniaturization overcomes macro sample analysis limitations: Salicylate-selective polystyrene nanoparticle-modified optical sensor, Talanta, 2019, 196, 436–441, DOI:10.1016/j.talanta.2018.12.073.
- R. Altman, B. Bosch, K. Brune, P. Patrignani and C. Young, Advances in NSAID development: evolution of diclofenac products using pharmaceutical technology, Drugs, 2015, 75(8), 859–877, DOI:10.1007/s40265-015-0392-z.
- L. Qian, S. Durairaj, S. Prins and A. Chen, Nanomaterial-based electrochemical sensors and biosensors for the detection of pharmaceutical compounds, Biosens. Bioelectron., 2021, 175, 112836, DOI:10.1016/j.bios.2020.112836.
- P. Daneshgar, P. Norouzi, M. R. Ganjali, R. Dinarvand and A. A. Moosavi-Movahedi, Determination of diclofenac on a dysprosium nanowire- modified carbon paste electrode accomplished in a flow injection system by advanced filtering, Sensors, 2009, 9(10), 7903–7918, DOI:10.3390/s91007903.
- G. Dowling, P. Gallo, S. Fabbrocino, L. Serpe and L. Regan, Determination of ibuprofen, ketoprofen, diclofenac and phenylbutazone in bovine milk by gas chromatography-tandem mass spectrometry, Food Addit. Contam., Part A: Chem., Anal., Control, Exposure Risk Assess., 2008, 25(12), 1497–1508, DOI:10.1080/02652030802383160.
- G. Burgot, F. Auffret and J. L. Burgot, Determination of acetaminophen by thermometric titrimetry, Anal. Chim. Acta, 1997, 343(1), 125–128, DOI:10.1016/S0003-2670(96)00613-7.
- Y. Ni, C. Liu and S. Kokot, Simultaneous kinetic spectrophotometric determination of acetaminophen and phenobarbital by artificial neural networks and partial least squares, Anal. Chim. Acta, 2000, 419, 185–196, DOI:10.1016/S0003-2670(00)00978-8.
- D. Easwaramoorthy, Y.-C. Yu and H.-J. Huang, Chemiluminescence detection of paracetamol by a luminol-permanganate based reaction, Anal. Chim. Acta, 2001, 439(1), 95–100, DOI:10.1016/S0003-2670(01)00968-0.
- C. Nebot, S. W. Gibb and K. G. Boyd, Quantification of human pharmaceuticals in water samples by high performance liquid chromatography–tandem mass spectrometry, Anal. Chim. Acta, 2007, 598(1), 87–94, DOI:10.1016/j.aca.2007.07.029.
- A. B. Moreira, H. P. M. Oliveira, T. D. Z. Atvars, I. L. T. Dias, G. O. Neto, E. A. G. Zagatto and L. T. Kubota, Direct determination of paracetamol in powdered pharmaceutical samples by fluorescence spectroscopy, Anal. Chim. Acta, 2005, 539(1), 257–261, DOI:10.1016/j.aca.2005.03.012.
- R. N. Goyal, V. K. Gupta and S. Chatterjee, Voltammetric biosensors for the determination of paracetamol at carbon nanotube modified pyrolytic graphite electrode, Sens. Actuators, B, 2010, 149(1), 252–258, DOI:10.1016/j.snb.2010.05.019.
- P. R. Dalmasso, M. L. Pedano and G. A. Rivas, Electrochemical determination of ascorbic acid and paracetamol in pharmaceutical formulations using a glassy carbon electrode modified with multi-wall carbon nanotubes dispersed in polyhistidine, Sens. Actuators, B, 2012, 173, 732–736, DOI:10.1016/j.snb.2012.07.087.
- R. T. Kachoosangi, G. G. Wildgoose and R. G. Compton, Sensitive adsorptive stripping voltammetric determination of paracetamol at multiwalled carbon nanotube modified basal plane pyrolytic graphite electrode, Anal. Chim. Acta, 2008, 618(1), 54–60, DOI:10.1016/j.aca.2008.04.053.
- S. I. Kaya, S. Kurbanoglu and S. A. Ozkan, Nanomaterials-Based Nanosensors for the Simultaneous Electrochemical Determination of Biologically Important Compounds: Ascorbic Acid, Uric Acid, and Dopamine, Crit. Rev. Anal. Chem., 2019, 49(2), 101–125, DOI:10.1080/10408347.2018.1489217.
- V. Chaudhary, N. Ashraf, M. Khalid, R. Walvekar, Y. Yang, A. Kaushik and Y. K. Mishra, Emergence of MXene–Polymer Hybrid Nanocomposites as High-Performance Next-Generation Chemiresistors for Efficient Air Quality Monitoring, Adv. Funct. Mater., 2022, 32(33), 2112913, DOI:10.1002/adfm.202112913.
- P. Manickam, S. A. Mariappan, S. M. Murugesan, S. Hansda, A. Kaushik, R. Shinde and S. P. Thipperudraswamy, Artificial Intelligence (AI) and Internet of Medical Things (IoMT) Assisted Biomedical Systems for Intelligent Healthcare, Biosensors, 2022, 12(8), 562, DOI:10.3390/bios12080562.
- V. Chaudhary, A. Kaushik, H. Furukawa and A. Khosla, Review—Towards 5th Generation AI and IoT Driven Sustainable Intelligent Sensors Based on 2D MXenes and Borophene, ECS Sensors Plus, 2022, 1(1), 013601, DOI:10.1149/2754-2726/ac5ac6.
- H. Montaseri and P. B. C. Forbes, Analytical techniques for the determination of acetaminophen: A review, TrAC, Trends Anal. Chem., 2018, 108, 122–134, DOI:10.1016/j.trac.2018.08.023.
- Y. Chen, Y. Zhu, Y. Zhao, J. Wang and M. Li, Insight into CuX (CuO, Cu2O, and CuS) for enhanced performance of CuX/g-C3N4 nanocomposites-based acetaminophen electrochemical sensors, Microchem. J., 2021, 163, 105884, DOI:10.1016/j.microc.2020.105884.
- J. Li, J. Liu, G. Tan, J. Jiang, S. Peng, M. Deng, D. Qian, Y. Feng and Y. Liu, High-sensitivity paracetamol sensor based on Pd/graphene oxide nanocomposite as an enhanced electrochemical sensing platform, Biosens. Bioelectron., 2014, 54, 468–475, DOI:10.1016/j.bios.2013.11.001.
- A. S. Adekunle, B. O. Agboola, J. Pillay and K. I. Ozoemena, Electrocatalytic detection of dopamine at single-walled carbon nanotubes–iron(III) oxide nanoparticles platform, Sens. Actuators, B, 2010, 148(1), 93–102, DOI:10.1016/j.snb.2010.03.088.
- G. Ozcelikay, S. Kurbanoglu, A. Yarman, F. W. Scheller and S. A. Ozkan, Au–Pt nanoparticles based molecularly imprinted nanosensor for electrochemical detection of the lipopeptide antibiotic drug Daptomycin, Sens. Actuators, B, 2020, 320, 128285, DOI:10.1016/j.snb.2020.128285.
- Y. X. Gan, A. H. Jayatissa, Z. Yu, X. Chen and M. Li, Hydrothermal Synthesis of Nanomaterials, J. Nanomater., 2020, 2020, 8917013, DOI:10.1155/2020/8917013.
-
F. A. Khan, Synthesis of nanomaterials: methods & technology, Applications of Nanomaterials in Human Health, Springer, 2020, pp. 15–21 Search PubMed.
- K. Annadurai, V. Sudha, G. Murugadoss and R. Thangamuthu, Electrochemical sensor based on hydrothermally prepared nickel oxide for the determination of 4-acetaminophen in paracetamol tablets and human blood serum samples, J. Alloys Compd., 2021, 852, 156911, DOI:10.1016/j.jallcom.2020.156911.
- R. Nurzulaikha, H. N. Lim, I. Harrison, S. S. Lim, A. Pandikumar, N. M. Huang, S. P. Lim, G. S. H. Thien, N. Yusoff and I. Ibrahim, Graphene/SnO2 nanocomposite-modified electrode for electrochemical detection of dopamine, Sens. Bio-Sens. Res., 2015, 5, 42–49, DOI:10.1016/j.sbsr.2015.06.002.
- X. Zhang, K. P. Wang, L. N. Zhang, Y. C. Zhang and L. Shen, Phosphorus-doped graphene-based electrochemical sensor for sensitive detection of acetaminophen, Anal. Chim. Acta, 2018, 1036, 26–32, DOI:10.1016/j.aca.2018.06.079.
- Z. Xu, H. Teng, J. Song, F. Gao, L. Ma, G. Xu and X. Luo, A nanocomposite consisting of MnO2 nanoflowers and the conducting polymer PEDOT for highly sensitive amperometric detection of paracetamol, Microchim. Acta, 2019, 186(8), 499, DOI:10.1007/s00604-019-3614-3.
- S. Ponnada, D. B. Gorle, M. S. Kiai, S. Rajagopal, R. K. Sharma and A. Nowduri, A facile, cost-effective, rapid, single-step synthesis of Ag–Cu decorated ZnO nanoflower-like composites (NFLCs) for electrochemical sensing of dopamine, Mater. Adv., 2021, 2(18), 5986–5996, 10.1039/d1ma00319d.
- Z. Lu, J. Zhong, Y. Zhang, M. Sun, P. Zou, H. Du, X. Wang, H. Rao and Y. Wang, MOF-derived Co3O4/FeCo2O4 incorporated porous biomass carbon: Simultaneous electrochemical determination of dopamine, acetaminophen and xanthine, J. Alloys Compd., 2021, 858, 157701, DOI:10.1016/j.jallcom.2020.157701.
- E. D. Razmi, H. Beitollahi, M. T. Mahani and M. Anjomshoa, TiO2/Fe3O4/Multiwalled Carbon Nanotubes Nanocomposite as Sensing Platform for Simultaneous Determination of Morphine and Diclofenac at a Carbon Paste Electrode, Russ. J. Electrochem., 2018, 54(12), 1132–1140, DOI:10.1134/S1023193518140057.
- S. P. Sajjadi, Sol–gel process and its application in Nanotechnology, J. Polym. Eng. Technol., 2005, 13, 38–41 Search PubMed.
- S. Sakka, Sol–Gel Process and Applications, Handb. Adv. Ceram., 2013, 883–910, DOI:10.1016/b978-0-12-385469-8.00048-4.
- Z. Bagherinasab, H. Beitollahi, M. Yousefi, M. Bagherzadeh and M. Hekmati, Rapid sol gel synthesis of BaFe12O19 nanoparticles: An excellent catalytic application in the electrochemical detection of tramadol in the presence of acetaminophen, Microchem. J., 2020, 156, 104803, DOI:10.1016/j.microc.2020.104803.
- B. Deiminiat, G. H. Rounaghi and M. H. Arbab-Zavar, Development of a new electrochemical imprinted sensor based on poly-pyrrole, sol–gel and multiwall carbon nanotubes for determination of tramadol, Sens. Actuators, B, 2017, 238, 651–659, DOI:10.1016/j.snb.2016.07.110.
- A. Zhu, G. Xu, L. Li, L. Yang, H. Zhou and X. Kan, Sol–Gel Imprinted Polymers Based Electrochemical Sensor for Paracetamol Recognition and Detection, Anal. Lett., 2013, 46(7), 1132–1144, DOI:10.1080/00032719.2012.753607.
- A. Anancia Grace, K. P. Divya, V. Dharuman and J. H. Hahn, Single step sol-gel synthesized Mn2O3–TiO2 decorated graphene for the rapid and selective ultra sensitive electrochemical sensing of dopamine, Electrochim. Acta, 2019, 302, 291–300, DOI:10.1016/j.electacta.2019.02.053.
- J. Luo, J. Cong, R. Fang, X. Fei and X. Liu, One-pot synthesis of a graphene oxide coated with an imprinted sol–gel for use in electrochemical sensing of paracetamol, Microchim. Acta, 2014, 181(11–12), 1257–1266, DOI:10.1007/s00604-014-1237-2.
- M. Rouhani and A. Soleymanpour, Molecularly imprinted sol-gel electrochemical sensor for sildenafil based on a pencil graphite electrode modified by Preyssler heteropolyacid/gold nanoparticles/MWCNT nanocomposite, Mikrochim. Acta, 2020, 187(9), 512, DOI:10.1007/s00604-020-04482-6.
- K. Petcharoen and A. Sirivat, Synthesis and characterization of magnetite nanoparticles via the chemical co-precipitation method, Mater. Sci. Eng., B, 2012, 177(5), 421–427, DOI:10.1016/j.mseb.2012.01.003.
- B. P. Singh, A. Kumar, A. P. Duarte, S. J. Rojas, M. Crespo-Medina, H. I. Areizaga-Martinez, C. A. Vega-Olivencia and M. S. Tomar, Synthesis, characterization, and electrochemical response of iron oxide nanoparticles for sensing acetaminophen, Mater. Res. Express, 2016, 3(10), 106105, DOI:10.1088/2053-1591/3/10/106105.
- M. Sivakumar, Activated Carbon -ZnO Nanocomposite for Electrochemical Sensing of Acetaminophen, Int. J. Electrochem. Sci., 2016, 8363–8373, DOI:10.20964/2016.10.51.
- I. Sheikhshoaie, F. Garakani Nejad and H. Beitollai, An electrochemical acetaminophen sensor based on La3+/Co3O4 nanoflowers modified graphite screen printed electrode architecture, Int. J. Nano Dimens., 2019, 10(2), 154–162 CAS.
- M. Taei, M. Shavakhi, H. Hadadzadeh, M. Movahedi, M. Rahimi and S. Habibollahi, Simultaneous determination of epinephrine, acetaminophen, and tryptophan using Fe2O3(0.5)/SnO2(0.5) nanocomposite sensor, J. Appl. Electrochem., 2014, 45(2), 185–195, DOI:10.1007/s10800-014-0756-1.
- B. Mutharani, R. Rajakumaran, S.-M. Chen, P. Ranganathan, T.-W. Chen, D. A. Al Farraj, M. Ajmal Ali and F. M. A. Al-Hemaid, Facile synthesis of 3D stone-like copper tellurate (Cu3TeO6) as a new platform for anti-inflammatory drug ibuprofen sensor in human blood serum and urine samples, Microchem. J., 2020, 159, 105378, DOI:10.1016/j.microc.2020.105378.
- C. Zhang, Z. Cao, G. Zhang, Y. Yan, X. Yang, J. Chang, Y. Song, Y. Jia, P. Pan, W. Mi, Z. Yang, J. Zhao and J. Wei, An electrochemical sensor based on plasma-treated zinc oxide nanoflowers for the simultaneous detection of dopamine and diclofenac sodium, Microchem. J., 2020, 158, 105237, DOI:10.1016/j.microc.2020.105237.
- F. Zamarchi and I. C. Vieira, Determination of paracetamol using a sensor based on green synthesis of silver nanoparticles in plant extract, J. Pharm. Biomed. Anal., 2021, 196, 113912, DOI:10.1016/j.jpba.2021.113912.
- T. Iranmanesh, M. M. Foroughi, S. Jahani and M. Shahidi Zandi, and H. Hassani Nadiki, Green and facile microwave solvent-free synthesis of CeO2 nanoparticle-decorated CNTs as a quadruplet electrochemical platform for ultrasensitive and simultaneous detection of ascorbic acid, dopamine, uric acid and acetaminophen, Talanta, 2020, 207, 120318, DOI:10.1016/j.talanta.2019.120318.
- F.-Y. Kong, S.-X. Gu, J.-Y. Wang, H.-L. Fang and W. Wang, Facile green synthesis of graphene–titanium nitride hybrid nanostructure for the simultaneous determination of acetaminophen and 4-aminophenol, Sens. Actuators, B, 2015, 213, 397–403, DOI:10.1016/j.snb.2015.02.120.
- K. Wang, C. Wu, F. Wang, N. Jing and G. Jiang, Co/Co3O4 Nanoparticles Coupled with Hollow Nanoporous Carbon Polyhedrons for the Enhanced Electrochemical Sensing of Acetaminophen, ACS Sustainable Chem. Eng., 2019, 7(22), 18582–18592, DOI:10.1021/acssuschemeng.9b04813.
- B. Avinash, C. R. Ravikumar, M. R. A. Kumar, H. P. Nagaswarupa, M. S. Santosh, A. S. Bhatt and D. Kuznetsov, Nano CuO: Electrochemical sensor for the determination of paracetamol and D-glucose, J. Phys. Chem. Solids, 2019, 134, 193–200, DOI:10.1016/j.jpcs.2019.06.012.
- M. Kenarkob and Z. Pourghobadi, Electrochemical sensor for acetaminophen based on a glassy carbon electrode modified with ZnO/Au nanoparticles on functionalized multi-walled carbon nano-tubes, Microchem. J., 2019, 146, 1019–1025, DOI:10.1016/j.microc.2019.02.038.
- V. Haridas, R. N. K. Z. Yaakob, S. Sugunan and B. N. Narayanan, Selective electrochemical determination of paracetamol using hematite/graphene nanocomposite modified electrode prepared in a green chemical route, Mater. Chem. Phys., 2021, 263, 124379, DOI:10.1016/j.matchemphys.2021.124379.
- L. Fu, A. Wang, G. Lai, C.-T. Lin, J. Yu, A. Yu, Z. Liu, K. Xie and W. Su, A glassy carbon electrode modified with N-doped carbon dots for improved detection of hydrogen peroxide and paracetamol, Microchim. Acta, 2018, 185(2), 87, DOI:10.1007/s00604-017-2646-9.
- P. A. Pandey, G. R. Bell, J. P. Rourke, A. M. Sanchez, M. D. Elkin, B. J. Hickey and N. R. Wilson, Physical Vapor Deposition of Metal Nanoparticles on Chemically Modified Graphene: Observations on Metal–Graphene Interactions, Small, 2011, 7(22), 3202–3210, DOI:10.1002/smll.201101430.
- J.-S. Park, W.-H. Chung, H.-S. Kim and Y.-B. Kim, Rapid fabrication of chemical-solution-deposited La0.6Sr0.4CoO3−δ thin films via flashlight sintering, J. Alloys Compd., 2017, 696, 102–108, DOI:10.1016/j.jallcom.2016.11.074.
- A. Khoobi, N. Soltani and M. Aghaei, Computational design and multivariate statistical analysis for electrochemical sensing platform of iron oxide nanoparticles in sensitive detection of anti-inflammatory drug diclofenac in biological fluids, J. Alloys Compd., 2020, 831, 154715, DOI:10.1016/j.jallcom.2020.154715.
- M. T. Swihart, Vapor-phase synthesis of nanoparticles, Curr. Opin. Colloid Interface Sci., 2003, 8(1), 127–133, DOI:10.1016/S1359-0294(03)00007-4.
-
E. Vanecht, Gold Nanoparticles in Ionic Liquids Prepared by Sputter Deposition. 2012.
- T. Soganci, R. Ayranci, E. Harputlu, K. Ocakoglu, M. Acet, M. Farle, C. G. Unlu and M. Ak, An effective non-enzymatic biosensor platform based on copper nanoparticles decorated by sputtering on CVD graphene, Sens. Actuators, B, 2018, 273, 1501–1507, DOI:10.1016/j.snb.2018.07.064.
-
D. Woermann and J. Janata, Principles of Chemical Sensors, Plenum Press, New York and London, 1989. 317 Seiten, Preis in Europa: US $47.40. 1990, Wiley Online Library Search PubMed.
-
J. Wang, Electroanalytical techniques in clinical chemistry and laboratory medicine, John Wiley & Sons, 1988 Search PubMed.
- J. Janata, Chemical sensors, Anal. Chem., 1992, 64(12), 196–219, DOI:10.1021/ac00036a012.
- C. A. Widrig, M. D. Porter, M. D. Ryan, T. G. Strein and A. G. Ewing, Dynamic electrochemistry: methodology and application, Anal. Chem., 1990, 62(12), 1–20, DOI:10.1021/ac00211a001.
- L. D. Bowers and P. W. Carr, Applications of immobilized enzymes in analytical chemistry, Anal. Chem., 1976, 48(7), 544A, DOI:10.1021/ac60371a033.
- H. H. Weetall, Immobilized enzymes. Analytical applications, Anal. Chem., 1974, 46(7), 602A–615A, DOI:10.1021/ac60343a035.
- A. P. Lima, A. C. Catto, E. Longo, E. Nossol, E. M. Richter and R. A. A. Munoz, Investigation on acid functionalization of double-walled carbon nanotubes of different lengths on the development of amperometric sensors, Electrochim. Acta, 2019, 299, 762–771, DOI:10.1016/j.electacta.2019.01.042.
-
J. Wang, Analytical Electrochemistry, VCH, Publishers. Inc., New York, 1994 Search PubMed.
- J. J. Lagowski, Ion-Selective Electrode Methodology, Vols. I and II (Covington, Arthur K., ed.), J. Chem. Educ., 1981, 58(1), A30, DOI:10.1021/ed058pA30.1.
- N. R. Stradiotto, H. Yamanaka and M. V. B. Zanoni, Electrochemical sensors: A powerful tool in analytical chemistry, SciELO Brasil, 2003, 159–173 CAS.
- A. Z. Sadek, W. Wlodarski, K. Kalantar-Zadeh, C. Baker and R. B. Kaner, Doped and dedoped polyaniline nanofiber based conductometric hydrogen gas sensors. Sensors and Actuators A: Physical, Sens. Actuators, A, 2007, 139(1–2), 53–57, DOI:10.1016/j.sna.2006.11.033.
- P. Ghosh, S. Biswas and A. Kushagra, Development of conductometric glucose sensor in nanomolar (nM) range from phantom blood serum, Mater. Today: Proc., 2021 DOI:10.1016/j.matpr.2021.05.627.
- L. Sun, Y. Guo, Y. Hu, S. Pan and Z. Jiao, Conductometric n-butanol gas sensor based on Tourmaline@ZnO hierarchical micro-nanostructures, Sens. Actuators, B, 2021, 337, 129793, DOI:10.1016/j.snb.2021.129793.
- Y. Wang, Y. Zhou, Y. Wang, R. Zhang, J. Li, X. Li and Z. Zang, Conductometric room temperature ammonia sensors based on titanium dioxide nanoparticles decorated thin black phosphorus nanosheets, Sens. Actuators, B, 2021, 349, 130770, DOI:10.1016/j.snb.2021.130770.
-
A. J. Bard, L. R. Faulkner and H. S. White, Electrochemical methods: fundamentals and applications, John Wiley & Sons, 2022 Search PubMed.
- A. T. Ezhil Vilian, M. Rajkumar and S. M. Chen,
In situ electrochemical synthesis of highly loaded zirconium nanoparticles decorated reduced graphene oxide for the selective determination of dopamine and paracetamol in presence of ascorbic acid, Colloids Surf., B, 2014, 115, 295–301, DOI:10.1016/j.colsurfb.2013.12.014.
- J. Wang, D. B. Luo, P. A. Farias and J. S. Mahmoud, Adsorptive stripping voltammetry of riboflavin and other flavin analogs at the static mercury drop electrode, Anal. Chem., 1985, 57(1), 158–162, DOI:10.1021/ac00279a039.
- X. Kang, J. Wang, H. Wu, J. Liu, I. A. Aksay and Y. Lin, A graphene-based electrochemical sensor for sensitive detection of paracetamol, Talanta, 2010, 81(3), 754–759, DOI:10.1016/j.talanta.2010.01.009.
- X. Zhang, Y. C. Zhang and J. W. Zhang, A highly selective electrochemical sensor for chloramphenicol based on three-dimensional reduced graphene oxide architectures, Talanta, 2016, 161, 567–573, DOI:10.1016/j.talanta.2016.09.013.
- N. Sebastian, W.-C. Yu and D. Balram, Electrochemical detection of an antibiotic drug chloramphenicol based on a graphene oxide/hierarchical zinc oxide nanocomposite, Inorg. Chem. Front., 2019, 6(1), 82–93, 10.1039/c8qi01000e.
- G. I. Mohammed, N. H. Khraibah, A. S. Bashammakh and M. S. El-Shahawi, Electrochemical sensor for trace determination of timolol maleate drug in real samples and drug residues using Nafion/carboxylated-MWCNTs nanocomposite modified glassy carbon electrode, Microchem. J., 2018, 143, 474–483, DOI:10.1016/j.microc.2018.08.011.
- B. K. Chethana, S. Basavanna and Y. Arthoba Naik, Voltammetric Determination of Diclofenac Sodium Using Tyrosine-Modified Carbon Paste Electrode, Ind. Eng. Chem. Res., 2012, 51(31), 10287–10295, DOI:10.1021/ie202921e.
- X. C. Song, X. Wang, Y. F. Zheng, R. Ma and H. Y. Yin, A hydrogen peroxide electrochemical sensor based on Ag nanoparticles grown on ITO substrate, J. Nanopart. Res., 2011, 13(10), 5449, DOI:10.1007/s11051-011-0532-7.
- C. Burda, X. Chen, R. Narayanan and M. A. El-Sayed, Chemistry and Properties of Nanocrystals of Different Shapes, Chem. Rev., 2005, 105(4), 1025–1102, DOI:10.1021/cr030063a.
- Z. Linting, L. Ruiyi, L. Zaijun, X. Qianfang, F. Yinjun and L. Junkang, An immunosensor for ultrasensitive detection of aflatoxin B1 with an enhanced electrochemical performance based on graphene/conducting polymer/gold nanoparticles/the ionic liquid composite film on modified gold electrode with electrodeposition, Sens. Actuators, B, 2012, 174, 359–365, DOI:10.1016/j.snb.2012.06.051.
- M. Ozcan, A. Basak and A. Uzunoglu, Construction of High-Performance Amperometric Acetaminophen Sensors Using Zn/ZnO-Decorated Reduced Graphene Oxide Surfaces, ECS J. Solid State Sci. Technol., 2020, 9(9), 093003, DOI:10.1149/2162-8777/ab951b.
- B. Liu, X. Ouyang, Y. Ding, L. Luo, D. Xu and Y. Ning, Electrochemical preparation of nickel and copper oxides-decorated graphene composite for simultaneous determination of dopamine, acetaminophen and tryptophan, Talanta, 2016, 146, 114–121, DOI:10.1016/j.talanta.2015.08.034.
- P. N. Manikandan and V. Dharuman, Electrochemical Simultaneous Sensing of Melatonin, Dopamine and Acetaminophen at Platinum Doped and Decorated Alpha Iron Oxide, Electroanalysis, 2017, 29(6), 1524–1531, DOI:10.1002/elan.201700054.
- F. Cao, Q. Dong, C. Li, J. Chen, X. Ma, Y. Huang, D. Song, C. Ji and Y. Lei, Electrochemical sensor for detecting pain reliever/fever reducer drug acetaminophen based on electrospun CeBiO nanofibers modified screen-printed electrode, Sens. Actuators, B, 2018, 256, 143–150, DOI:10.1016/j.snb.2017.09.204.
- A. Diouf, M. Moufid, D. Bouyahya, L. Österlund, N. El Bari and B. Bouchikhi, An electrochemical sensor based on chitosan capped with gold nanoparticles combined with a voltammetric electronic tongue for quantitative aspirin detection in human physiological fluids and tablets, Mater. Sci. Eng. C, 2020, 110, 110665, DOI:10.1016/j.msec.2020.110665.
- W. Yang, K. R. Ratinac, S. P. Ringer, P. Thordarson, J. J. Gooding and F. Braet, Carbon Nanomaterials in Biosensors: Should You Use Nanotubes or Graphene?, Angew. Chem., Int. Ed., 2010, 49(12), 2114–2138, DOI:10.1002/anie.200903463.
- S. A. R. Alavi-Tabari, M. A. Khalilzadeh and H. Karimi-Maleh, Simultaneous determination of doxorubicin and dasatinib as two breast anticancer drugs uses an amplified sensor with ionic liquid and ZnO nanoparticle, J. Electroanal. Chem., 2018, 811, 84–88, DOI:10.1016/j.jelechem.2018.01.034.
- A. U. Alam, Y. Qin, M. M. R. Howlader, N.-X. Hu and M. J. Deen, Electrochemical sensing of acetaminophen using multi-walled carbon nanotube and β-cyclodextrin, Sens. Actuators, B, 2018, 254, 896–909, DOI:10.1016/j.snb.2017.07.127.
- B.-R. Adhikari, M. Govindhan and A. Chen, Sensitive Detection of Acetaminophen with Graphene-Based Electrochemical Sensor, Electrochim.
Acta, 2015, 162, 198–204, DOI:10.1016/j.electacta.2014.10.028.
- N. Dou, S. Zhang and J. Qu, Simultaneous detection of acetaminophen and 4-aminophenol with an electrochemical sensor based on silver–palladium bimetal nanoparticles and reduced graphene oxide, RSC Adv., 2019, 9(54), 31440–31446, 10.1039/c9ra05987c.
- C. Wu, J. Li, X. Liu, H. Zhang, R. Li, G. Wang, Z. Wang, Q. Li and E. Shangguan, Simultaneous voltammetric determination of epinephrine and acetaminophen using a highly sensitive CoAl-OOH/reduced graphene oxide sensor in pharmaceutical samples and biological fluids, Mater. Sci. Eng., C, 2021, 119, 111557, DOI:10.1016/j.msec.2020.111557.
- Z. Qian, X. Shan, L. Chai, J. Ma, J. Chen and H. Feng, Si-Doped Carbon Quantum Dots: A Facile and General Preparation Strategy, Bioimaging Application, and Multifunctional Sensor, ACS Appl. Mater. Interfaces, 2014, 6(9), 6797–6805, DOI:10.1021/am500403n.
- X. Li, M. Rui, J. Song, Z. Shen and H. Zeng, Carbon and Graphene Quantum Dots for Optoelectronic and Energy Devices: A Review, Adv. Funct. Mater., 2015, 25(31), 4929–4947, DOI:10.1002/adfm.201501250.
- V. Kumar, G. Toffoli and F. Rizzolio, Fluorescent Carbon Nanoparticles in Medicine for Cancer Therapy, ACS Med. Chem. Lett., 2013, 4(11), 1012–1013, DOI:10.1021/ml400394a.
- A. Cernat, M. Tertis, R. Sandulescu, F. Bedioui, A. Cristea and C. Cristea, Electrochemical sensors based on carbon nanomaterials for acetaminophen detection: A review, Anal. Chim. Acta, 2015, 886, 16–28, DOI:10.1016/j.aca.2015.05.044.
- T. V. Gopal, T. M. Reddy, P. Shaikshavali and G. Venkataprasad, Eco-friendly and bio-waste based hydroxyapatite/reduced graphene oxide hybrid material for synergic electrocatalytic detection of dopamine and study of its simultaneous performance with acetaminophen and uric acid, Surf. Interfaces, 2021, 24, 101145, DOI:10.1016/j.surfin.2021.101145.
- T. S. H. Pham, P. J. Mahon, G. Lai, L. Fu, C. T. Lin and A. Yu, Cauliflower-like Platinum Particles Decorated Reduced Graphene Oxide for Sensitive Determination of Acetaminophen, Electroanalysis, 2019, 31(9), 1758–1768, DOI:10.1002/elan.201900138.
- S. Berto, L. Carena, F. Valmacco, C. Barolo, E. Conca, D. Vione, R. Buscaino, M. Fiorito, C. Bussi, O. Abollino and M. Malandrino, Application of an electro-activated glassy-carbon electrode to the determination of acetaminophen (paracetamol) in surface waters, Electrochim. Acta, 2018, 284, 279–286, DOI:10.1016/j.electacta.2018.07.145.
- W. Liang, L. Liu, Y. Li, H. Ren, T. Zhu, Y. Xu and B.-C. Ye, Nitrogen-rich porous carbon modified electrochemical sensor for the detection of acetaminophen, J. Electroanal. Chem., 2019, 855, 113496, DOI:10.1016/j.jelechem.2019.113496.
- N. G. Tsierkezos, S. H. Othman and U. Ritter, Nitrogen-doped multi-walled carbon nanotubes for paracetamol sensing, Ionics, 2013, 19(12), 1897–1905, DOI:10.1007/s11581-013-0930-1.
- M. M. Barsan, C. T. Toledo and C. M. A. Brett, New electrode architectures based on poly(methylene green) and functionalized carbon nanotubes: Characterization and application to detection of acetaminophen and pyridoxine, J. Electroanal. Chem., 2015, 736, 8–15, DOI:10.1016/j.jelechem.2014.10.026.
- K. Sarhangzadeh, A. A. Khatami, M. Jabbari and S. Bahari, Simultaneous determination of diclofenac and indomethacin using a sensitive electrochemical sensor based on multiwalled carbon nanotube and ionic liquid nanocomposite, J. Appl. Electrochem., 2013, 43(12), 1217–1224, DOI:10.1007/s10800-013-0609-3.
- M. Roushani, Z. Rahmati, S. Farokhi, S. J. Hoseini and R. H. Fath, The development of an electrochemical nanoaptasensor to sensing chloramphenicol using a nanocomposite consisting of graphene oxide functionalized with (3-Aminopropyl)
triethoxysilane and silver nanoparticles, Mater. Sci. Eng. C, 2020, 108, 110388, DOI:10.1016/j.msec.2019.110388.
- E. Suresh, K. Sundaram, B. Kavitha, S. M. Rayappan and N. S. Kumar, Simultaneous Electrochemical Determination of Paracetamol and Ibuprofen at The Glassy Carbon Electrode, J. Adv. Chem. Sci., 2016, 369–372 Search PubMed.
- W. Hao, Y. Zhang, J. Fan, H. Liu, Q. Shi, W. Liu, Q. Peng and G. Zang, Copper Nanowires Modified with Graphene Oxide Nanosheets for Simultaneous Voltammetric Determination of Ascorbic Acid, Dopamine and Acetaminophen, Molecules, 2019, 24(12), 2320, DOI:10.3390/molecules24122320.
- F. Hasanpour, M. Taei and S. Tahmasebi, Ultra-sensitive electrochemical sensing of acetaminophen and codeine in biological fluids using CuO/CuFe2O4 nanoparticles as a novel electrocatalyst, J. Food Drug Anal., 2018, 26(2), 879–886, DOI:10.1016/j.jfda.2017.10.001.
- L. P. Lin, P. S. Khiew, W. S. Chiu and M. T. T. Tan, A Disposable Electrochemical Sensing Platform for Acetaminophen Based on Graphene/ZrO2 Nanocomposite Produced via a Facile, Green Synthesis Method, IEEE Sens. J., 2018, 18(19), 7907–7916, DOI:10.1109/jsen.2018.2864326.
- E. Tamilalagan, S. Vetri Selvi, S.-M. Chen, M. Akilarasan, S. Maheshwaran, T.-W. Chen, A. M. Al-Mohaimeed, W. A. Al-onazi, M. Soliman Elshikh and X. Liu, Fabrication of p–n Junction (Ni/Zn)O and Reduced Graphene Oxide (rGO) Nanocomposites for the Electrocatalysis of Analgesic Drug (Acetaminophen) Detection in Pharmaceutical and Biological Samples, J. Electrochem. Soc., 2021, 168(3), 036501, DOI:10.1149/1945-7111/abe6eb.
- E. Nikpanje, M. Bahmaei and A. M. Sharif, Determination of Ascorbic Acid, Acetaminophen, and Caffeine in Urine, Blood Serum by Electrochemical Sensor Based on ZnO–Zn2SnO4–SnO2 Nanocomposite and Graphene, J. Electrochem. Sci. Technol., 2021, 12(2), 173–187, DOI:10.33961/jecst.2020.00724.
- A. Afkhami, H. Khoshsafar, H. Bagheri and T. Madrakian, Preparation of NiFe(2)O(4)/graphene nanocomposite and its application as a modifier for the fabrication of an electrochemical sensor for the simultaneous determination of tramadol and acetaminophen, Anal. Chim. Acta, 2014, 831, 50–59, DOI:10.1016/j.aca.2014.04.061.
- N. Demir, K. Atacan, M. Ozmen and S. Z. Bas, Design of a new electrochemical sensing system based on MoS2–TiO2/reduced graphene oxide nanocomposite for the detection of paracetamol, New J. Chem., 2020, 44(27), 11759–11767, 10.1039/d0nj02298e.
- N. S. Anuar, W. J. Basirun, M. Ladan, M. Shalauddin and M. S. Mehmood, Fabrication of platinum nitrogen-doped graphene nanocomposite modified electrode for the electrochemical detection of acetaminophen, Sens. Actuators, B, 2018, 266, 375–383, DOI:10.1016/j.snb.2018.03.138.
- P. Shaikshavali, T. Madhusudana Reddy, V. N. Palakollu, R. Karpoormath, Y. Subba Rao, G. Venkataprasad, T. V. Gopal and P. Gopal, Multi walled carbon nanotubes supported CuO–Au hybrid nanocomposite for the effective application towards the electrochemical determination of Acetaminophen and 4-Aminophenol, Synth. Met., 2019, 252, 29–39, DOI:10.1016/j.synthmet.2019.04.009.
- K.-J. Huang, L. Wang, J. Li and Y.-M. Liu, Electrochemical sensing based on layered MoS2–graphene composites, Sens. Actuators, B, 2013, 178, 671–677, DOI:10.1016/j.snb.2013.01.028.
- K. Kimuam, N. Rodthongkum, N. Ngamrojanavanich, O. Chailapakul and N. Ruecha, Single step preparation of platinum nanoflowers/reduced graphene oxide electrode as a novel platform for diclofenac sensor, Microchem. J., 2020, 155, 104744, DOI:10.1016/j.microc.2020.104744.
- R. N. Goyal, S. Chatterjee and A. R. S. Rana, The effect of modifying an edge-plane pyrolytic graphite electrode with single-wall carbon nanotubes on its use for sensing diclofenac, Carbon, 2010, 48(14), 4136–4144, DOI:10.1016/j.carbon.2010.07.024.
- F. Nasiri, G. H. Rounaghi, N. Ashraf and B. Deiminiat, A new electrochemical sensing
platform for quantitative determination of diclofenac based on gold nanoparticles decorated multiwalled carbon nanotubes/graphene oxide nanocomposite film, Int. J. Environ. Anal. Chem., 2019, 101(2), 153–166, DOI:10.1080/03067319.2019.1661396.
- M. M. Charithra and J. G. Manjunatha, Electroanalytical determination of acetaminophen using polymerized carbon nanocomposite based sensor, Chem. Data Collect., 2021, 33, 100718, DOI:10.1016/j.cdc.2021.100718.
- Y.-m Shi, X. Zhang, L. Mei, D.-e Han, K. Hu, L.-Q. Chao, X.-m Li and M.-s Miao, Sensitive acetaminophen electrochemical sensor with amplified signal strategy via non-covalent functionalization of soluble tetrahydroxyphthalocyanine and graphene, Microchem. J., 2021, 160, 105609, DOI:10.1016/j.microc.2020.105609.
- A. Yiğit, Y. Yardım, M. Çelebi, A. Levent and Z. Şentürk, Graphene/Nafion composite film modified glassy carbon electrode for simultaneous determination of paracetamol, aspirin and caffeine in pharmaceutical formulations, Talanta, 2016, 158, 21–29, DOI:10.1016/j.talanta.2016.05.046.
- M. Roushani and F. Shahdost-fard, Applicability of AuNPs@N-GQDs nanocomposite in the modeling of the amplified electrochemical Ibuprofen aptasensing assay by monitoring of riboflavin, Bioelectrochemistry, 2019, 126, 38–47, DOI:10.1016/j.bioelechem.2018.11.005.
- R. Manjunatha, D. H. Nagaraju, G. S. Suresh, J. S. Melo, S. F. D'Souza and T. V. Venkatesha, Electrochemical detection of acetaminophen on the functionalized MWCNTs modified electrode using layer-by-layer technique, Electrochim. Acta, 2011, 56(19), 6619–6627, DOI:10.1016/j.electacta.2011.05.018.
- Z. A. Alothman, N. Bukhari, S. M. Wabaidur and S. Haider, Simultaneous electrochemical determination of dopamine and acetaminophen using multiwall carbon nanotubes modified glassy carbon electrode, Sens. Actuators, B, 2010, 146(1), 314–320, DOI:10.1016/j.snb.2010.02.024.
- X. Chen, J. Zhu, Q. Xi and W. Yang, A high performance electrochemical sensor for acetaminophen based on single-walled carbon nanotube–graphene nanosheet hybrid films, Sens. Actuators, B, 2012, 161(1), 648–654, DOI:10.1016/j.snb.2011.10.085.
- Y. Fan, J. H. Liu, H. T. Lu and Q. Zhang, Electrochemical behavior and voltammetric determination of paracetamol on Nafion/TiO2-graphene modified glassy carbon electrode, Colloids Surf., B, 2011, 85(2), 289–292, DOI:10.1016/j.colsurfb.2011.02.041.
- M. Liu, Q. Chen, C. Lai, Y. Zhang, J. Deng, H. Li and S. Yao, A double signal amplification platform for ultrasensitive and simultaneous detection of ascorbic acid, dopamine, uric acid and acetaminophen based on a nanocomposite of ferrocene thiolate stabilized Fe(3)O(4)@Au nanoparticles with graphene sheet, Biosens. Bioelectron., 2013, 48, 75–81, DOI:10.1016/j.bios.2013.03.070.
- M. Sakthivel, M. Sivakumar, S.-M. Chen, Y.-S. Hou, V. Veeramani, R. Madhu and N. Miyamoto, A Facile Synthesis of Cd(OH)2-rGO Nanocomposites for the Practical Electrochemical Detection of Acetaminophen, Electroanalysis, 2017, 29(1), 280–286, DOI:10.1002/elan.201600351.
-
H. Yakowitz, Methods of quantitative X-ray analysis used in electron probe microanalysis and scanning electron microscopy, in Practical Scanning Electron Microscopy, 1975, Springer, pp.327–372 Search PubMed.
- L. Wang, T. Meng, J. Sun, S. Wu, M. Zhang, H. Wang and Y. Zhang, Development of Pd/Polyoxometalate/nitrogen-doping hollow carbon spheres tricomponent nanohybrids: A selective electrochemical sensor for acetaminophen, Anal. Chim. Acta, 2019, 1047, 28–35, DOI:10.1016/j.aca.2018.09.042.
- N. P. Shetti, S. J. Malode, D. S. Nayak, K. R. Reddy, C. V. Reddy and K. Ravindranadh, Silica gel-modified electrode as an electrochemical sensor for the detection of acetaminophen, Microchem. J., 2019, 150, 104206, DOI:10.1016/j.microc.2019.104206.
- N. Dou and J. Qu, Rapid synthesis of a hybrid of rGO/AuNPs/MWCNTs for sensitive sensing of 4-aminophenol and acetaminophen simultaneously, Anal. Bioanal. Chem., 2021, 413(3), 813–820, DOI:10.1007/s00216-020-02856-6.
- H. Farzad, M. Bahmaei and M. Davallo, Electrochemical Determination of Propranolol, Acetaminophen and Folic Acid in Urine, and Human Plasma Using Cu2O–CuO/rGO/CPE, Russ. J. Electrochem., 2021, 57(4), 357–374, DOI:10.1134/s1023193521040054.
- T. W. Tseng, T. W. Chen, S. M. Chen, T. Kokulnathan, F. Ahmed, P. M. Z. Hasan, A. L. Bilgrami and S. Kumar, Construction of strontium phosphate/graphitic-carbon nitride: A flexible and disposable strip for acetaminophen detection, J. Hazard. Mater., 2021, 410, 124542, DOI:10.1016/j.jhazmat.2020.124542.
- L. Guo, L. Hao, Y. Zhang, X. Yang, Q. Wang, Z. Wang and C. Wang, Metal–organic framework precursors derived Ni-doping porous carbon spheres for sensitive electrochemical detection of acetaminophen, Talanta, 2021, 228, 122228, DOI:10.1016/j.talanta.2021.122228.
- X. Tan, T. Mu, S. Wang, J. Li, J. Huang, H. Huang, Y. Pu and G. Zhao, Simultaneous determination of Acetaminophen and dopamine based on a water-soluble pillar[6]arene and ultrafine Pd nanoparticle-modified covalent organic framework nanocomposite, Analyst, 2021, 146(1), 262–269, 10.1039/d0an01717e.
|
This journal is © The Royal Society of Chemistry 2023 |
Click here to see how this site uses Cookies. View our privacy policy here.