DOI:
10.1039/D2MA01016J
(Paper)
Mater. Adv., 2023,
4, 910-916
Preparation and photocatalytic activity of ZnGa2O4-β-Ga2O3 thin films†
Received
4th November 2022
, Accepted 9th December 2022
First published on 4th January 2023
Abstract
ZnGa2O4 and ZnGa2O4-β-Ga2O3 thin films were prepared via aerosol-assisted chemical vapor deposition (AACVD) using various ratios of the Zn and Ga precursors, resulting in the formation of amorphous ZnGa2O4 and Ga2O3. The formation of crystalline zinc gallate and heterostructure zinc gallate thin films was achieved by annealing the resulting films at high temperatures under air. The ZnGa2O4-β-Ga2O3 thin films showed enhanced photocatalytic activity compared with ZnGa2O4. The photocatalytic enhancement of the ZnGa2O4-β-Ga2O3 is explained by the formation of type-II band alignment at the interfaces between ZnGa2O4 and Ga2O3, resulting in enhanced photoinduced charge separation in the material.
1. Introduction
Oxide semiconductors have attracted much interest as an effective material for photocatalysts because of their high photocatalytic activity and mechanical and chemical durability.1–3 Semiconductor-based photocatalysts have been applied for various applications, such as for self-cleaning, organic/inorganic pollutant decomposition, photoelectrochemical water splitting and antimicrobial coatings. Since the first study on p-block metal oxides of MIn2O4, M2SnO4 and M2Sb2O7 (M = Ca, Sr) was reported by Inoue et al.,4–7 semiconductors with a d10 electronic configuration have been widely studied and show promise as photocatalysts.8–12 Among the p-block metal oxide photocatalysts, zinc gallate (ZnGa2O4) is also a promising photocatalyst and has been applied to water splitting,13–15 organic pollutant degradation16 and CO2 reduction17,18 applications under UV irradiation. However, ZnGa2O4 has had limited practical success due to its wide band gap energy (4.1–4.5 eV) and high recombination rate of the electrons and holes,19 resulting in low photocatalytic performance. Therefore, broadening the light absorption and preventing the rapid recombination of the photogenerated electron–hole pairs of ZnGa2O4 photocatalysts, has become an area of focus.
Doping ZnGa2O4 with foreign ions is one approach to broaden the light absorption and improve the photocatalytic performance. Doping ZnGa2O4 with cations has been investigated showing improved visible light absorption.20,21 Although the band gap was successfully reduced, some metal-doped ZnGa2O4 showed low photocatalytic efficiency due to rapid electron–hole recombination.22 The formation of heterojunction structures is an attractive strategy to improve the photocatalytic properties of ZnGa2O4 by increasing the charge separation in photocatalytic processes. Heterojunction structures, such as ZnGa2O4/N-rGO14 and ZnO/ZnGa2O4,23 have been developed for improving the photocatalytic performance of ZnGa2O4.
Many methods have been used to synthesize ZnGa2O4 particles or thin films including hydrothermal,24 solid-state,25 sol–gel,26 chemical vapor deposition,27 and RF magnetron sputtering.28 Among the large number of synthesis methods to produce ZnGa2O4 materials, the synthesis of thin films is widely convenient for practical applications compared with powders, due to the problems in the separation and recovery of powders. Aerosol-assisted chemical vapor deposition (AACVD) is a promising technique for thin film preparation because it is a simple, scalable, and cost-efficient technique that allows good control over physical and chemical properties.29,30 In AACVD, precursors with low vapor pressure are dissolved in an appropriate solvent and the solution is aerosolized and transported into the CVD chamber using a carrier gas. The chemical composition of the starting material can play a crucial role in influencing the chemical and physical properties of the deposited films.
In this study, the formation of crystalline zinc gallate and heterostructure zinc gallate (ZnGa2O4-β-Ga2O3) thin films was studied. A deeper understanding of the effect of the heterostructure formation on band alignment, charge transfer and photocatalytic activity is required to explain the phenomena at the interfaces between ZnGa2O4 and Ga2O3.
2. Experimental section
2.1. Preparation of ZnGa2O4 and ZnGa2O4-β-Ga2O3 thin films
Zinc acetylacetonate [(Zn(C5H7O2)2)], 99.9% and gallium acetylacetonate [(Ga(C5H7O2)3], 99.9%) from Sigma Aldrich were used as the zinc and gallium source materials, respectively. In a round bottom flask, solutions for AACVD were prepared by dissolving 0.5 mmol of zinc acetylacetonate and different amounts of gallium acetylacetonate (0.5, 0.7 and 1.0 mmol) in 40 mL methanol. Using a piezoelectric ultrasonic humidifier, a precursor mist was created and delivered to the reaction chamber with a flow rate of 1.0 L min−1 nitrogen gas. The deposition was carried out in a reactor at 400 °C. The obtained films were then annealed at 700 °C in air for 5 h, yielding clear thin films.
2.2. Analytical methods
Film morphology and thickness were studied using top- and side-view scanning electron microscopy (SEM) in a JEOL6301 instrument (10 kV). X-ray diffraction (XRD) analysis was carried out using a Bruker-Axs D8 (GADDS) diffractometer. The instrument operated with a monochromated Cu X-ray source with Cu Kα1 (λ = 1.54056 Å) and Cu Kα2 radiation (λ = 1.54439 Å) emitted with an intensity ratio of 2
:
1 and a 2D area X-ray detector with a resolution of 0.01°. Films were analyzed with a glancing incident angle (θ) of 1°. The diffraction patterns obtained were confirmed using database standards. UV/Vis spectroscopy was performed using a double monochromated PerkinElmer Lambda 950 UV/Vis/NIR spectrophotometer in the 300–800 nm range. X-Ray photoelectron spectroscopy (XPS) was performed using a Thermo K alpha spectrometer with monochromated Al Kα radiation, a dual beam charge compensation system and constant pass energy of 50 eV. Survey scans were collected in the range of 0–1200 eV. High-resolution XPS spectra were used for the principal peaks of Zn (2p) and Ga (2p), and deconvoluted using CasaXPS software with the calibration of C1s at 284.5 eV. The surface roughness of the films was characterized by atomic force microscopy (AFM) on a Keysight 5600LS scanning probe microscope taken at a scale of 5 μm × 5 μm.
2.3. Photocatalytic tests
The intrinsic photocatalytic properties of the films were investigated through the photodegradation of octadecanoic (stearic acid), which was used here as a model organic pollutant.31 Stearic acid is very stable under UV irradiation in the absence of a photocatalyst. In these experiments, the films were dip coated with a thin layer of stearic acid (0.05 M solution in chloroform) and then monitored under UVC irradiation over a period of 32 h using a PerkinElmer RX-I Fourier transform infrared (FTIR) spectrometer. Plots of integrated areas of characteristic C–H infrared bands at 2923 and 2853 cm−1 were produced and the photodegradation rates were estimated from linear regression of the initial 30–50% of the curves. A conversion factor from the literature (1 cm−1 = 9.7 × 1015 molecules of stearic acid)32 allowed for the estimation of the number of molecules of stearic acid degraded upon irradiation time. The light source used was a UVC (λ = 254 nm) Vilber-Lourmat BLB lamp (2 × 8 W, I = 1.0 mW cm−2). The irradiance of the lamp was measured using a UVX radiometer (UVP).
3. Results and discussion
Amorphous thin films were grown on quartz substrates using aerosol-assisted chemical vapor deposition (AACVD) at 400 °C, as detailed in the experimental section. On the heated substrates, the precursors react and deposit on the substrates. The unreacted precursors and waste products are carried away to the exhaust, as shown in Fig. S1 (ESI†). A series of zinc gallate thin films were deposited using different molar ratios of Zn/Ga in the precursor mixture, resulting in the Zn/Ga mole ratios of 0.45, 0.26 and 0.17 in the films, as determined by elemental analysis (EDS), henceforth referred to as ZG-[Zn/Ga mole ratio], namely ZG-0.45, ZG-0.26 and ZG-0.17, respectively. X-ray diffraction (XRD) of the as-deposited films from AACVD showed no patterns and therefore they were presumed to be amorphous (Fig. S2, ESI†).
The amorphous phase was analyzed by XPS, where the energy separation between the Zn 2p3/2 and Ga 2p3/2 peaks was studied. The energy separation between Zn 2p3/2 and Ga 2p3/2 peaks (ΔE) has been used as a tool to distinguish whether the obtained product is a complete ZnGa2O4 or a composite of ZnO and Ga2O3, where the formation of ZnGa2O4 provides lower ΔE compared with a mixture of ZnO and Ga2O3.33,34 In this work, ΔE of a mixture of commercial ZnO and Ga2O3 was studied providing a value of 96.6 eV. It was found that ΔE of the commercial mixture is higher than ΔE of the as-deposited amorphous thin films giving a value of 96.2 eV (Table S1, ESI†). This indicates the formation of zinc gallate under AACVD. The amorphous thin films were annealed under air at 700 °C, resulting in the formation of crystalline zinc gallate, which was confirmed by XRD as shown in Fig. 1. For those samples with low Ga content (ZG-0.45) only the pattern of cubic-phase ZnGa2O4 (JCPDS no. 86-0415)35 was found. Relatively high Ga contents (ZG-0.26 and ZG-0.17), showed additional reflection peaks that were consistent with β-Ga2O3 (JCPDS no. 43-1012).22,36
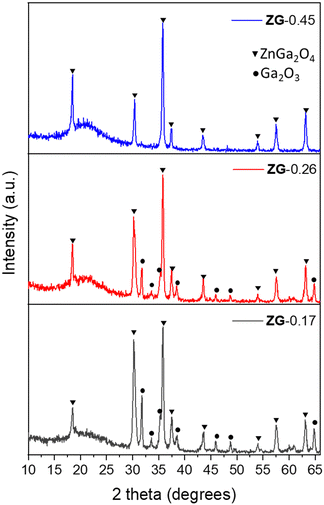 |
| Fig. 1 XRD patterns of thin films annealed under air on quartz substrates at 700 °C of ZG-0.45, ZG-0.26 and ZG-0.17, respectively. Triangle symbols correspond to cubic-phase ZnGa2O4 (JCPDS no. 86-0415) and circle symbols correspond to β-Ga2O3 (JCPDS no. 43-1012). | |
The morphology and cross-section SEM images of the samples are shown in Fig. 2(a)–(c). SEM analysis showed similar surface morphologies in all thin films, with the thickness of ZG-0.45, ZG-0.26 and ZG-0.17 being 230, 280 and 340 nm, respectively. The surface topography of the annealed films (ZG-0.45, ZG-0.26 and ZG-0.17) was investigated by AFM, taken with a scale of 5 μm × 5 μm, as shown in Fig. 2.
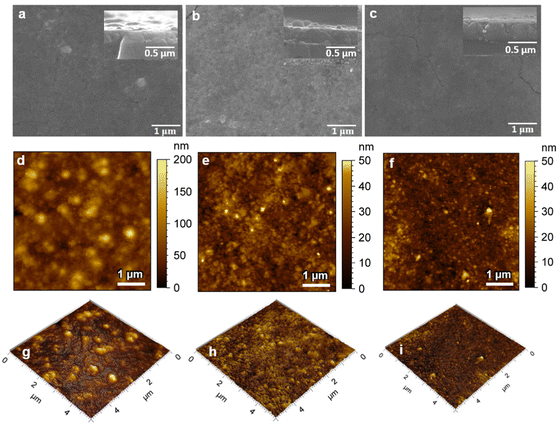 |
| Fig. 2 Scanning electron microscopy (SEM) of (a) ZG-0.45, (b) ZG-0.26 and (c) ZG-0.17. Atomic Force Microscopy (AFM) images showing the surface morphology (top) and 3D images (bottom) of (d), (g) ZG-0.45, (e), (h) ZG-0.26 and (f), (i) ZG-0.17 thin films on quartz substrates prepared by the AACVD method at different mole ratios of Zn and Ga in the precursor mixture. The surface topography of the annealed films investigated by AFM, taken with a scale of 5 μm × 5 μm. | |
Inspection of these images shows that the surface area and surface roughness of the thin films was not significantly affected by the ratio of Zn and Ga in the films. The surface roughness (rms roughness) of the annealed films (ZG-0.45, ZG-0.26 and ZG-0.17) was 63, 51 and 73, respectively, with surface areas of 26.7, 25.6 and 25.7 μm2, respectively, as depicted in Table 1.
Table 1 Structural, optical, and functional parameters of ZnGa2O4 and ZnGa2O4-β-Ga2O3 heterojunction films prepared with different ratios of zinc acetylacetonate and gallium acetylacetonate in the precursor mixture
Sample |
Zn/Ga atomic ratio |
ZnGa2O4 : Ga2O3 |
E
g (eV) |
Film thickness (nm) |
Surface area (μm2) |
RMS roughness (nm) |
Surface area (μm2) |
ξ × 10−4 (molecules photon−1) |
In precursor solution |
In as-deposited thin films |
ZG-0.45 |
1.0 |
0.45 |
9 : 1 |
5.2 |
230 |
26.69 |
63 |
26.69 |
0.50 |
ZG-0.26 |
0.7 |
0.26 |
1 : 1 |
5.1 |
280 |
25.59 |
51 |
25.59 |
1.64 |
ZG-0.17 |
0.5 |
0.17 |
1 : 2 |
4.9 |
340 |
25.67 |
74 |
25.67 |
0.80 |
High-resolution XPS was further employed to investigate the composition and surface electron state of the thin films. Fig. 3 shows the XPS spectra revealing (a) Ga 2p and (b) Zn 2p of the films ZG-0.45, ZG-0.26 and ZG-0.17, respectively. Binding energy (BE) calibration was carried out using the C1s peak located at 284.5 eV. The XPS spectrum of the ZG-0.45 reveals a symmetric peak for the Ga 2p orbital, with a BE of 1118.5 eV, while the spectra of ZG-0.26 and ZG-0.17 can be deconvoluted into two peaks, with binding energy values of Ga 2p3/2 at 1118.4 and 1119.8 eV, respectively, which are associated with Ga3+ species in Ga2O3 and ZnGa2O4, respectively.37
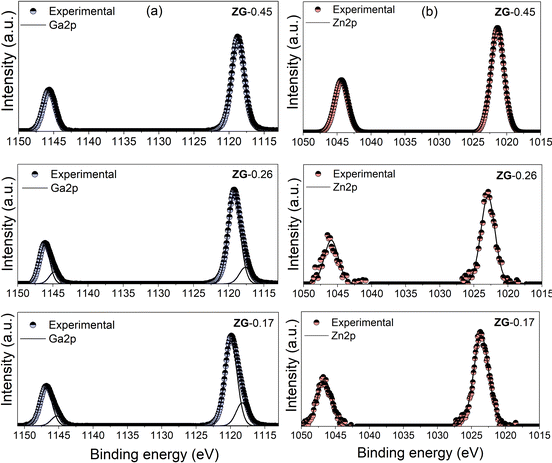 |
| Fig. 3 High-resolution XPS of (a) Ga 2p and (b) Zn 2p spectra of ZG-0.45, ZG-0.26 and ZG-0.17 deposited by AACVD on quartz. | |
The XPS spectrum of the Zn 2p1/2 peaks in the films was ∼1022 eV, which could be related to the formation of ZnGa2O4.38 To investigate the vertical distribution of ZnGa2O4 and Ga2O3 in the films, the Zn/Ga ratio was calculated using XPS depth profiling shown in Fig. S3 (ESI†). The Zn/Ga atomic ratio of thin films with etching shows that there is a homogenous mix of ZnGa2O4 and Ga2O3 in the thin films (ZG-0.45, ZG-0.26 and ZG-0.17).
The light absorption properties of the materials were studied by using UV-vis spectroscopy. The absorption edges of ZG-0.45, ZG-0.26 and ZG-0.17 were located at around 250 nm. The band gap energy of the materials was determined from the Tauc plot for indirect band gap absorption, as shown in Fig. 4(a). The samples (ZG-0.45, ZG-0.26 and ZG-0.17) had Eg values of 5.2, 5.1 and 4.9 eV, respectively.
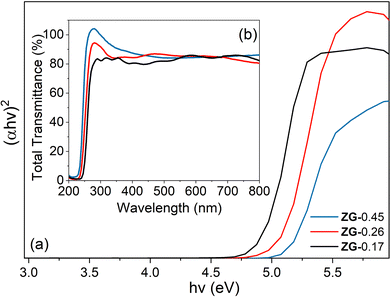 |
| Fig. 4 (a) Tauc plot allowing an estimation of the band gap energies and the inset figure (b) shows the UV-Vis total transmittance of the annealed samples (ZG-0.45, ZG-0.26 and ZG-0.17) deposited by the AACVD method at different mole ratios between Zn/Ga in the precursor mixture of 1, 0.7 and 0.5, respectively. | |
The photocatalytic activity of the thin films was evaluated by testing the degradation rate of stearic acid, a model organic pollutant under UVC irradiation (BLB lamp, 1.0 mW cm−1), as shown in Fig. 5 and Table 1. In the absence of catalyst, the detected concentration of stearic acid was stable under illumination, while the presence of catalyst (ZG-0.45, ZG-0.26 and ZG-0.17) leads to obvious degradation of stearic acid. The degradation of steric acid under irradiation results from the redox reactions of steric acid by photogenerated electrons and holes on the photocatalytic surface, providing the production of non-toxic CO2 and H2O.39,40 The corresponding degradation curves are plotted against irradiation time in Fig. 5(a). The trend of degradation curves was as expected with the ZnGa2O4-β-Ga2O3 heterostructure thin film (ZG-0.26 and ZG-0.17) being a more effective photocatalyst than ZnGa2O4 (ZG-0.45). In the case of ZG-0.26, with the ratio between ZnGa2O4 and Ga2O3 being 1
:
1, this provided higher photocatalytic performance, compared with the other thin films. As mentioned above, the surface area of the thin films was similar, and the photocatalytic efficiency was independent of the thickness of the thin films (Table 1). The apparent enhancement could then be related to the optimal ratio between ZnGa2O4 and Ga2O3 in the thin film.
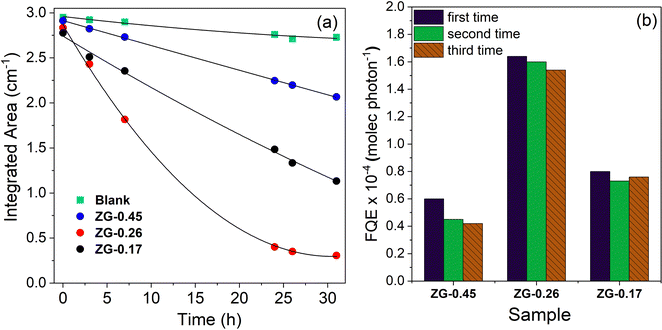 |
| Fig. 5 (a) Integrated area of the IR spectra obtained from the initial rates of photodegradation of stearic acid upon irradiation time under UV illumination (UVC, λ = 254 nm, I = 1.0 mW cm−2), with thin films (ZG-0.45, ZG-0.26 and ZG-0.17) acting as photocatalysts in the photodegradation. (b) Corresponding formal quantum efficiencies (ξ), given as molecules degraded per incident photon (units, molecules photon−1), upon cycling experiments. A blank reference corresponds to the quartz substrate without the photocatalytic coating. | |
The fitting of the initial degradation steps (zero-order kinetics) allowed for the estimation of the formal quantum efficiencies (ξ, units molecules photon−1), given as the number of acid molecules degraded per incident photon (Fig. 5(b)). The cycle experiments resulted in a slight drop of the initial ξ values of the thin films. The drop of photocatalytic performance in the cycling experiments might be due to carbon contamination during the photodegradation of organic pollutants.
The higher photocatalytic performance of heterostructures (ZG-0.26 and ZG-0.17) might result from the formation of a heterojunction, which is an interface between the two regions of dissimilar semiconductors. In order to classify the type of band alignment in the interface, the valence band potential and band gap energy of ZnGa2O4 and β-Ga2O3 were considered. The band structures of ZnGa2O4 and β-Ga2O3 were analyzed using XPS showing that the valence band potential of β-Ga2O3 was 1.5 eV more positive than that of ZnGa2O4.22 ZnGa2O4 has a wide bandgap energy of ∼4.1–4.5 eV being similar to the bandgap energy of β-Ga2O3 (4.8 eV). Therefore, the band alignment of the ZnGa2O4-β-Ga2O3 heterojunction could be a type-II band alignment, which can enhance charge separation, as shown in Scheme 1.
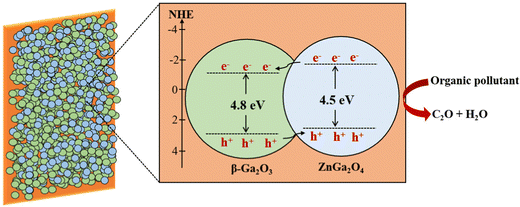 |
| Scheme 1 A schematic description of charge transfers across the ZnGa2O4-β-Ga2O3 heterojunction. | |
The photogenerated electrons tend to migrate from the conduction band (CB) of ZnGa2O4 to that of β-Ga2O3, while the generated holes transfer from β-Ga2O3 to ZnGa2O4. Consequently, the recombination process in this material is decreased, benefiting the enhancement of the photocatalytic performance. The higher photocatalytic performance of ZG-0.26 than ZG-0.17 might result from achieving an ideal ratio between ZnGa2O4 and β-Ga2O3 (1
:
1) in the ZnGa2O4-β-Ga2O3 heterojunction, resulting in the increase of the heterojunction interface in the material.
4. Conclusion
In this research, the ability to tune the composition of ZnGa2O4 and β-Ga2O3 thin films by varying the ratios of Zn and Ga precursors in the starting mixture under aerosol-assisted chemical vapor deposition (AACVD) has been demonstrated. The optimal ratio between the Zn and Ga precursor was found to be 0.7, which showed promising photocatalytic performance of the final product (ZG-0.26). The ZnGa2O4-β-Ga2O3 heterojunction possesses type-II band alignment, resulting in enhanced photocatalytic properties of the material. This result is a step forward toward the fabrication of an optimized photocatalytic material and calls for the implementation of synthesis strategies of zinc gallate.
Conflicts of interest
There are no conflicts to declare.
Acknowledgements
This work is supported by The Development and Promotion of Science and Technology Talents Project for a fellowship of P. Promdet. I. P. P. and C. J. C. thank EPSRC for grant EP/L0177709/1. The authors would like to thank Prof. Andreas Kafizas and Dr Raul Quesada-Cabrera for useful discussions on photocatalysis. The authors would also like to thank Prof. Robert Palgrave and Dr Sanjayan Sathasivam for XPS and Jian Guo for AFM.
References
- J. J. Rueda-Marquez, I. Levchuk, P. Fernández Ibañez and M. Sillanpää, A Critical Review on Application of Photocatalysis for Toxicity Reduction of Real Wastewaters, J. Cleaner Prod., 2020, 258, DOI:10.1016/j.jclepro.2020.120694.
- S. Dong, J. Feng, M. Fan, Y. Pi, L. Hu, X. Han, M. Liu, J. Sun and J. Sun, Recent Developments in Heterogeneous Photocatalytic Water Treatment Using Visible Light-Responsive Photocatalysts: A Review, RSC Adv., 2015, 5(19), 14610–14630, 10.1039/c4ra13734e.
- S. Banerjee, D. D. Dionysiou and S. C. Pillai, Self-Cleaning Applications of TiO2 by Photo-Induced Hydrophilicity and Photocatalysis, Appl. Catal., B, 2015, 176–177, 396–428, DOI:10.1016/j.apcatb.2015.03.058.
- K. Ikarashi, J. Sato, H. Kobayashi, N. Saito, H. Nishiyama and Y. Inoue, Photocatalysis for Water Decomposition by RuO2-Dispersed ZnGa2O4 with d10 Configuration, J. Phys. Chem. B, 2002, 106(35), 9048–9053, DOI:10.1021/jp020539e.
- J. Sato, N. Saito, H. Nishiyama and Y. Inoue, New Photocatalyst Group for Water Decomposition of RuO2-Loaded p-Block Metal (In, Sn, and Sb) Oxides with d10 Configuration, J. Phys. Chem. B, 2001, 105(26), 6061–6063, DOI:10.1021/jp010794j.
- J. Sato, N. Saito, H. Nishiyama and Y. Inoue, Photocatalytic Activity for Water Decomposition of RuO2-Loaded SrIn2O4 with d10 Configuration, Chem. Lett., 2001, 868–869, DOI:10.1246/cl.2001.868.
- J. Sato, N. Saito, H. Nishiyama and Y. Inoue, Photocatalytic Water Decomposition by RuO2-Loaded Antimonates, M2Sb2O7 (M = Ca, Sr), CaSb2O6 and NaSbO3, with d10 Cofiguration, J. Photochem. Photobiol., A, 2002, 148(1–3), 85–89, DOI:10.1016/S1010-6030(02)00076-X.
- K. Maeda and K. Domen, Solid Solution of GaN and ZnO as a Stable Photocatalyst for Overall Water Splitting under Visible Light, Chem. Mater., 2010, 22(3), 612–623, DOI:10.1021/cm901917a.
- K. R. Reyes-Gil, E. A. Reyes-García and D. Raftery, Nitrogen-Doped In2O3 Thin Film Electrodes for Photocatalytic Water Splitting, J. Phys. Chem. C, 2007, 111(39), 14579–14588, DOI:10.1021/jp072831y.
- Q. Liu, Y. Zhou, J. Kou, X. Chen, Z. Tian, J. Gao, S. Yan and Z. Zou, High-Yield Synthesis of Ultralong and Ultrathin Zn2GeO4 Nanoribbons toward Improved Photocatalytic Reduction of CO2 into Renewable Hydrocarbon Fuel, J. Am. Chem. Soc., 2010, 132(41), 14385–14387, DOI:10.1021/ja1068596.
- Y. Hou, X. Wang, L. Wu, Z. Ding and X. Fu, Efficient Decomposition of Benzene over a β-Ga2O3 Photocatalyst under Ambient Conditions, Environ. Sci. Technol., 2006, 40(18), 5799–5803, DOI:10.1021/es061004s.
- K. Maeda, T. Takata, M. Hara, N. Saito, Y. Inoue, H. Kobayashi and K. Domen, GaN: ZnO Solid Solution as a Photocatalyst for Visible-Light-Driven Overall Water Splitting, J. Am. Chem. Soc., 2005, 127, 8286–8287, DOI:10.1021/ja0518777.
- M. Zhong, Y. Li, I. Yamada and J.-J. Delaunay, ZnO–ZnGa2O4 Core–Shell Nanowire Array for Stable Photoelectrochemical Water Splitting, Nanoscale, 2012, 4(5), 1509–1514, 10.1039/C2NR11451H.
- X. P. Bai, X. Zhao and W. L. Fan, Preparation and Enhanced Photocatalytic Hydrogen-Evolution Activity of ZnGa2O4/N-RGO Heterostructures, RSC Adv., 2017, 7(84), 53145–53156, 10.1039/c7ra09981a.
- C. Zeng, T. Hu, N. Hou, S. Liu, W. Gao, R. Cong and T. Yang, Photocatalytic Pure Water Splitting Activities for ZnGa2O4 synthesized by Various Methods, Mater. Res. Bull., 2015, 61, 481–485, DOI:10.1016/j.materresbull.2014.10.041.
- W. Zhang, J. Zhang, Z. Chen and T. Wang, Photocatalytic Degradation of Methylene Blue by ZnGa2O4 thin Films, Catal. Commun., 2009, 10(13), 1781–1785, DOI:10.1016/j.catcom.2009.06.004.
- Q. Liu, D. Wu, Y. Zhou, H. Su, R. Wang, C. Zhang, S. Yan, M. Xiao and Z. Zou, Single-Crystalline, Ultrathin ZnGa2O4 Nanosheet Scaffolds to Promote Photocatalytic Activity in CO2 Reduction into Methane, ACS Appl. Mater. Interfaces, 2014, 6(4), 2356–2361, DOI:10.1021/am404572g.
- Y. Zhang, P. Li, L. Q. Tang, Y. Q. Li, Y. Zhou, J. M. Liu and Z. G. Zou, Robust, Double-Shelled ZnGa2O4 hollow Spheres for Photocatalytic Reduction of CO2 to Methane, Dalton Trans., 2017, 46(32), 10564–10568, 10.1039/c6dt04668a.
- P. Li, X. Zhao, H. Sun, L. Wang, B. Song, B. Gao and W. Fan, Theoretical Studies on the Form and Effect of N-Doping in an ZnGa2O4 Photocatalyst, RSC Adv., 2016, 6(78), 74483–74492, 10.1039/c6ra09655g.
- W. Zhang, J. Zhang, X. Lan, Z. Chen and T. Wang, Photocatalytic Performance of ZnGa2O4 for Degradation of Methylene Blue and Its Improvement by Doping with Cd, Catal. Commun., 2010, 11(14), 1104–1108, DOI:10.1016/j.catcom.2010.05.021.
- X. Xu, Y. Xie, S. Ni, A. K. Azad and T. Cao, Photocatalytic H2 Production from Spinels ZnGa2−xCrxO4 (0 ≤ x ≤ 2) Solid Solutions, J. Solid State Chem., 2015, 230, 95–101, DOI:10.1016/j.jssc.2015.05.029.
- X. Wang, S. Shen, S. Jin, J. Yang, M. Li, X. Wang, H. Han and C. Li, Effects of Zn2+ and Pb2+ Dopants on the Activity of Ga2O3-Based Photocatalysts for Water Splitting, Phys. Chem. Chem. Phys., 2013, 15(44), 19380–19386, 10.1039/c3cp53333f.
- M. Baojun, L. Keying, S. Weiguang and L. Wanyi, One-Pot Synthesis of ZnO/ZnGa2O4 Heterojunction with X/XY Structure for Improved Photocatalytic Activity, Appl. Surf. Sci., 2014, 317, 682–687, DOI:10.1016/j.apsusc.2014.08.089.
- M. Sun, D. Li, W. Zhang, Z. Chen, H. Huang, W. Li, Y. He and X. Fu, Rapid Microwave Hydrothermal Synthesis of ZnGa2O4 with High Photocatalytic Activity toward Aromatic Compounds in Air and Dyes in Liquid Water, J. Solid State Chem., 2012, 190, 135–142, DOI:10.1016/j.jssc.2012.02.027.
- M. M. Can, G. Hassnain Jaffari, S. Aksoy, S. I. Shah and T. Firat, Synthesis and Characterization of ZnGa2O4 Particles Prepared by Solid State Reaction, J. Alloys Compd., 2013, 549, 303–307, DOI:10.1016/j.jallcom.2012.08.137.
- T. Sei, Y. Nomura and T. Tsuchiya, Preparation of ZnGa2O4 Thin Film by Sol–Gel Process and Effect of Reduction on Its Electric Conductivity, J. Non-Cryst. Solids, 1997, 218, 135–138, DOI:10.1016/S0022-3093(97)00163-4.
- C. E. Knapp, J. A. Manzi, A. Kafizas, I. P. Parkin and C. J. Carmalt, Aerosol-Assisted Chemical Vapour Deposition of Transparent Zinc Gallate Films, ChemPlusChem, 2014, 79(7), 1024–1029, DOI:10.1002/cplu.201402037.
- R. Reshmi, K. M. Krishna, R. Manoj and M. K. Jayaraj, Pulsed Laser Deposition of ZnGa2O4 Phosphor Films, Surf. Coat. Technol., 2005, 198(1–3 SPEC. ISS.), 345–349, DOI:10.1016/j.surfcoat.2004.10.072.
- C. Sotelo-Vazquez, N. Noor, A. Kafizas, R. Quesada-Cabrera, D. O. Scanlon, A. Taylor, J. R. Durrant and I. P. Parkin, Multifunctional P-Doped TiO2 Films: A New Approach to Self-Cleaning, Transparent Conducting Oxide Materials, Chem. Mater., 2015, 27(9), 3234–3242, DOI:10.1021/cm504734a.
- A. M. Alotaibi, S. Sathasivam, B. A. D. Williamson, A. Kafizas, C. Sotelo-Vazquez, A. Taylor, D. O. Scanlon and I. P. Parkin, Chemical Vapor Deposition of Photocatalytically Active Pure Brookite TiO2 Thin Films, Chem. Mater., 2018, 30(4), 1353–1361 CrossRef CAS.
- M. J. Powell, R. Quesada-Cabrera, W. L. Travis and I. P. Parkin, High-Throughput Synthesis of Core-Shell and Multi-Shelled Materials by Fluidised Bed Chemical Vapour Deposition. Case Study: Double-Shell Rutile-Anatase Particles, J. Mater. Chem. A, 2015, 3(33), 17241–17247, 10.1039/c5ta03526k.
- R. Quesada-Cabrera, C. Sotelo-Vazquez, J. C. Bear, J. A. Darr and I. P. Parkin, Photocatalytic Evidence of the Rutile-to-Anatase Electron Transfer in Titania, Adv. Mater. Interfaces, 2014, 1(6), 1–7, DOI:10.1002/admi.201400069.
- Y. Yuan, W. Du and X. Qian, ZnXGa2O3+x (0 ≤ x ≤ 1) Solid Solution Nanocrystals: Tunable Composition and Optical Properties, J. Mater. Chem., 2012, 22(2), 653–659, 10.1039/c1jm13091a.
- L. Zou, X. Xiang, M. Wei, F. Li and D. G. Evans, Single-Crystalline ZnGa2O4 Spinel Phosphor via a Single-Source Inorganic Precursor Route, Inorg. Chem., 2008, 47(4), 1361–1369, DOI:10.1021/ic7012528.
- T. A. Safeera, R. Khanal, J. E. Medvedeva, A. I. Martinez, G. Vinitha and E. I. Anila, Low Temperature Synthesis and Characterization of Zinc Gallate Quantum Dots for Optoelectronic Applications, J. Alloys Compd., 2018, 740(May), 567–573, DOI:10.1016/j.jallcom.2018.01.035.
- J. Zhang, Z. Liu, C. Lin and J. Lin, A Simple Method to Synthesize β-Ga2O3 Nanorods and Their Photoluminescence Properties, J. Cryst. Growth, 2005, 280(1–2), 99–106, DOI:10.1016/j.jcrysgro.2005.02.060.
- Y. B. Seung, W. S. Hee, W. N. Chan and J. Park, Synthesis of Blue-Light-Emitting ZnGa2O4 Nanowires Using Chemical Vapor Deposition, Chem. Commun., 2004,(16), 1834–1835, 10.1039/b405592f.
- E. Chikoidze, C. Sartel, I. Madaci, H. Mohamed, C. Vilar, B. Ballesteros, F. Belarre, E. del Corro, P. Vales-Castro and G. Sauthier,
et al., P-Type Ultrawide-Band-Gap Spinel ZnGa2O4: New Perspectives for Energy Electronics, Cryst. Growth Des., 2020, 20(4), 2535–2546, DOI:10.1021/acs.cgd.9b01669.
- J. Krýsa, G. Waldner, H. Měšt’ánková, J. Jirkovský and G. Grabner, Photocatalytic Degradation of Model Organic Pollutants on an Immobilized Particulate TiO2 Layer. Roles of Adsorption Processes and Mechanistic Complexity, Appl. Catal., B, 2006, 64(3–4), 290–301, DOI:10.1016/j.apcatb.2005.11.007.
- M. I. Litter, Heterogeneous Photocatalysis: Transition Metal Ions in Photocatalytic Systems, Appl. Catal., B, 1999, 23(2–3), 89–114, DOI:10.1016/S0926-3373(99)00069-7.
|
This journal is © The Royal Society of Chemistry 2023 |
Click here to see how this site uses Cookies. View our privacy policy here.