DOI:
10.1039/D3MA00002H
(Paper)
Mater. Adv., 2023,
4, 1687-1693
Luminescent protein–rare earth fluoride nanoflowers†
Received
1st January 2023
, Accepted 28th February 2023
First published on 1st March 2023
Abstract
Protein–inorganic hybrid nanomaterials are a new type of functional materials that combine the advantages of inorganic and organic polymers and nanomaterials. We herein report a simple one-pot method for synthesizing organic–inorganic hybrid nanoflowers under mild biomineralization conditions using proteins as an organic component and lanthanide fluorides as an inorganic component. The as-prepared protein–rare earth fluoride nanoflowers exhibit high performance in maintaining enzyme stability and catalytic activity due to their high surface area and graded nanostructures, and have finely tunable luminescence properties of lanthanide ions. Various proteins such as trypsin, BSA, collagen and FtsZ have all displayed excellent capability to produce Na5Yb9F32 nanoflowers, demonstrating that protein-templated biomineralization provides a universal and robust strategy for the synthesis of protein–rare earth fluoride hybrid nanoflowers. The novel luminescent protein–rare earth fluoride nanoflowers may have promising applications in biosensing, biocatalysis and medical diagnostics and treatment.
Introduction
Nanomaterials with a higher surface area to volume ratio have been known to have superior advantages for improving the surface reaction efficiency compared to bulk materials. Nanoflowers as a newly developed class of nanoparticles with flower-like morphology have extraordinary features such as excellent catalytic ability, strong adsorption capacity, and efficient loading capability for multiple applications in catalysis, biosensors, and medicine, and have attracted the attention of scientists worldwide.1–4 However, the synthesis of nanoflowers is an arduous task requiring harsh conditions in their making, including toxic organic reagents, high pressures, and high temperatures. Therefore, controlling the morphological characteristics of nanoflowers to tailor their structure is an intricate task, and applying these particles to the field of biochemistry is even more daunting.
Biomineralization is a remarkable strategy by which organisms phenomenally utilize the composition of organic and inorganic matrixes to produce functional materials with hierarchically ordered structures,5–7 sparking inspiration for the design of novel materials. When using proteins as templates to synthesize Cu3(PO4)2·3H2O organic–inorganic hybrid nanoflowers, which possess a high surface-to-volume ratio, the immobilised proteases in the hybrid exhibit higher catalytic efficacy and better thermal stability compared to free proteases.8 Recently, copper–protein,9–12 calcium–protein,13,14 manganese–protein hybrid nanoflowers,15 copper–DNA hybrid nanoflowers,16 and capsular hybrid nanoflowers17,18 have been reported and have been shown to have many unexpected applications in catalysis,19–21 biosensors,22–25 and drug delivery.26–29
Rare earth nanoparticles (NPs) received intense interest due to their promising applications in biosensing,30 photovoltaic devices,31 near-infrared bioimaging32 and photodynamic therapy.33 In particular, rare earth fluoride NPs have attracted much attention, compared to their oxide counterparts. The fluoride host materials showed distinguished strengths such as low vibrational energies, low phonon energies and high chemical stability, endowing them as a research hotspot in the field of biomedicine. Rare earth fluoride nanomaterials have been used for various imaging studies by doping. For example, upconverting alkaline rare-earth fluorides doped with Yb3+ and Er3+ ions,34 fluorescence-enhanced Yb3+/Tm3+-codoped fluoride35 and Nd3+/Yb3+-codoped luminescent fluoride nanoparticles allowed high contrast time-gated in vivo NIR II window imaging.36
A variety of methods have been explored to create rare earth fluorides including chemical vapor deposition,37 sol–gel processes,38 microemulsion methods,39 nonhydrolytic routes,40 and hydrothermal processes,41 while each approach suffered from its own drawbacks. For instance, the most used hydrothermal synthesis had low yield and required high-temperature conditions. The toxic organometallic precursors and organic solvents also raised serious concerns of potential environmental pollution. The development of innovative environmentally friendly methods for the synthesis of rare earth fluoride NPs with well-defined shapes and good dispersibility remains highly challenging, while the production of rare earth fluoride nanoflowers has not been reported yet.
Herein we have developed a novel protein-templated biomineralized strategy for the synthesis of monodisperse rare earth fluoride nanoflowers in an aqueous buffer at room temperature. This is a mild one-pot process for synthesizing organic–inorganic hybrid nanoflowers under biomineralization conditions. With this facile and safe synthesis method, it is possible to fabricate nanoflowers simply by adding proteins to rare earth fluoride solution and it does not require any toxic elements or extreme harsh conditions. The abundant presence of amide and carboxyl groups of proteins provides sites for the growth of rare earth fluoride crystals. The organic substance involved in the synthesis is subjected to less manipulation compared with other conventional methods to maintain the activity of the immobilized enzyme. Therefore, the immobilized proteases have been demonstrated to be far more stable and reusable than their free counterparts, and their catalytic activity became remarkably increased. The prepared protein–Na5Yb9F32 nanoflowers display unique luminescence properties. The robust protein–rare earth fluoride nanoflowers may have promising applications in various fields such as biocatalysis, luminescent matrix materials, and medical diagnosis.
Experimental section
Materials
YbN3O9·5H2O, NaF, Eu(NO3)3·6H2O and Tb(NO3)3·5H2O were purchased from Aladdin. Trypsin was provided by Sangon Biotech. Bovine serum albumin (BSA) and Folin–Ciocalteu's phenol reagent were obtained from Solarbio. Casein was provided by Yuanye Bio-Technology. Urea, anhydrous sodium carbonate, and trichloroacetic acid (TCA) were obtained from Guangfu Fine Chemical. Collagen and FtsZ were expressed and purified according to the previously reported method.42–44 All solvents were of reagent grade or HPLC grade.
Synthesis of protein–Na5Yb9F32 hybrid nanoflowers
Trypsin–Na5Yb9F32 hybrid nanoflowers were prepared from a mixture of 685 μL of trypsin solution (1.5 mg mL−1) and 100 μL of Yb(NO3)3 solution (0.5 M). The reaction solution was stirred for 60 minutes to obtain a homogeneous mixture, and 300 μL of NaF solution (0.5 M) was then added and stirred for 10 minutes. The mixture was incubated for 12–36 hours at 25 °C. After centrifugation, the precipitates were washed three times using ethanol and air dried at room temperature. Other types of protein–Na5Yb9F32 hybrid nanoflowers were obtained following similar protocols with different concentrations of proteins (6.0 mg mL−1 BSA; 6.0 mg mL−1 collagen; and 1.5 mg mL−1 Ftsz).
Characterization of trypsin–Na5Yb9F32 hybrid nanoflowers
The X-ray diffraction (XRD) images of the synthesised hybrid nanomaterials were obtained using a Rigaku D/ma-2500 diffractometer with Cu Kα radiation (40 kV, 200 mA) at a scanning rate of 0.02° s−1 in the 2θ range from 10° to 90°. X-ray photoelectron spectroscopy (XPS) patterns were recorded using a Kaltos Axis UltraDLD X-ray photoelectron spectroscope (Kratos Analytical, Manchester, UK) with a monochrome X-ray source using Alkα (1486.6 eV) radiation. The binding energies were measured and corrected by referencing the C 1s line at 284.5 eV. Fourier-transform infrared (FT-IR) spectra were measured using a Nicolet NEXUS 670 infrared spectrometer. Thermogravimetric analysis (TGA) was carried out on a TGA/NETZSCH STA449F3 instrument under a nitrogen atmosphere with a heating rate of 10 °C min−1 from 25 °C to 800 °C. Scanning Electron Microscopy (SEM) experiments were performed on a Hitachi S-4800 field emission scanning electron microscope (Hitachi Limited, Japan). TEM (transmission electron microscopy), HRTEM (high-resolution transmission electron microscopy), SAED (selected area electron diffraction), and electron diffraction (EDX) were performed at 200 kV using a JEM-2100 transmission electron microscope (JEOL, Japan). The specific surface area and pore size of the nanoflowers were analysed by the Brunauer–Emmett–Teller (BET) and Barrett–Joyner–Halenda (BJH) methods using a physisorption analyzer (Micromeritics ASAP2020 porosimeter, USA).
Photoluminescence spectroscopy
Using a Xe lamp as the excitation source, photoluminescence emission spectra were acquired on a FLS920 fluorescence spectrophotometer (Edinburgh Instruments, England). The emission spectra were recorded for the protein–Na5Yb9F32:Tb3+ (or Eu3+) aggregates at λex = 369 nm and λex = 394 nm, respectively.
The enzymatic activity of trypsin–Na5Yb9F32 hybrid nanoflowers and free trypsin
The Folin assay was used to determine the enzymatic activity of trypsin–Na5Yb9F32 hybrid nanoflowers. Casein was used as a substrate. Casein solution (10 g L−1) was prepared in PBS buffer (50 mM, pH 7.4). Trypsin–Na5Yb9F32 (10 mg) was added to casein (1 mL, 10 g L−1) and incubated at 30 °C for 30 minutes. The supernatant was collected after centrifugation for 5 minutes (9000 rpm). 2 mL of TCA solution (0.4 M) was added to the supernatant for 5 minutes. After centrifugation, the supernatant was harvested. 5 mL of Na2CO3 solution (0.4 M) and 1 mL of Ciocalteu's phenol reagent were mixed with 1 mL of the supernatant. To complete the color development reaction, the mixture solution was incubated at 40 °C for 20 minutes. The absorbance at 763 nm was monitored to evaluate the casein hydrolysis performance. The activity of free trypsin was assessed by the same procedure using the corresponding amount of trypsin in the hybrid nanoflowers.
The enzymatic profiles of trypsin–Na5Yb9F32 and free trypsin under denatured conditions were investigated in the presence of different amounts of urea or ACN. In order to investigate the thermal stability of trypsin–Na5Yb9F32 and free trypsin, their enzymatic activities were simultaneously monitored at 55 °C at different time intervals for 10 h using the Folin assay. Meanwhile, the enzymatic activities of trypsin–Na5Yb9F32 and free trypsin were measured at various temperatures (40–55 °C). The relative activities of trypsin–Na5Yb9F32 and free trypsin at different temperatures were normalised to the value at 40 °C.
The reusability of trypsin–Na5Yb9F32 hybrid nanoflowers
The reusability of trypsin–Na5Yb9F32 was investigated by recombinant collagen (V-CL) digestion assay as previously described.45 Briefly, recombinant collagen V-CL (5 mg mL−1, 50 mM glycine buffer, pH 8.6) was incubated with 2 mg of trypsin–Na5Yb9F32 at 25 °C for ∼12 hours. The supernatant was obtained by centrifugation and stored at −20 °C. The precipitates were washed three times with a glycine buffer and then reused for another cycle. The VCL cleavage experiments were carried out eight times following the same procedure. All the collected supernatants were used for SDS-PAGE analysis.
Results and discussion
Synthesis and characterization of trypsin–Na5Yb9F32 hybrid nanoflowers
Trypsin–Na5Yb9F32 hybrid nanomaterials were produced by incubating a mixture of trypsin (0.95 mg mL−1), Yb(NO3)3 (46 mM) and NaF (138 mM) at 25 °C for 12 hours. The crystallinity of the nanomaterials was characterised by the XRD (X-ray diffraction) technique, and their diffraction profiles were consistent with Na5Yb9F32 (JCPDS No. 27-1426) (Fig. 1a). The elemental composition of the nanomaterials was determined by XPS (X-ray photoelectron spectroscopy), and it indicated the presence of C, N, O, Na, Yb and F elements, which corresponded to proteins and Na5Yb9F32, respectively (Fig. 1b). These results demonstrated that trypsin provides a good bio template to create pure Na5Yb9F32 rare earth fluoride crystals under mild incubation conditions.
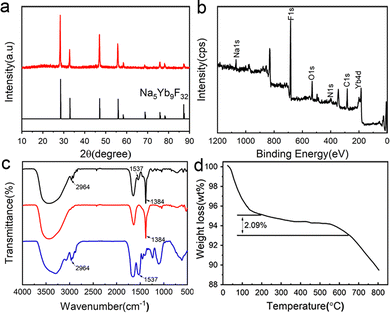 |
| Fig. 1 XRD (a), XPS (b), FT-IR (c), and TGA (d) characterization of the prepared trypsin–Na5Yb9F32 hybrid nanomaterials. The XRD pattern of the standard Na5Yb9F32 crystal (black) is shown below the plot of the hybrid nanomaterials (red) (a). FT-IR spectra of trypsin–Na5Yb9F32 hybrid nanomaterial (black), Na5Yb9F32 (red), and trypsin (blue) (c). | |
The FT-IR spectra of hybrid nanomaterials displayed a strong IR band at 1384 cm−1, which corresponded to the characteristic absorption of the Yb–F bond of Na5Yb9F32 (Fig. 1c). The observed vibration peaks at 2964 cm−1 and 1537 cm−1 were ascribed to the C–H and N–H bonds, respectively, suggesting the presence of trypsin within the synthesised Na5Yb9F32 crystal (Fig. 1c). The content of trypsin in the hybrid nanomaterials was further estimated by thermogravimetric analysis (TGA) (Fig. 1d). The TGA curve showed three stages of weight loss: loss of water below 200 °C, thermal decomposition of protein between 200 and 650 °C, and decomposition of Na5Yb9F32 above 650 °C. It indicated that approximately 2.09% of trypsin was encapsulated in the hybrid nanomaterials (Fig. 1d). All these results indicated that the mild incubation of the mixture of trypsin, Yb(NO3)3 and NaF led to the production of hybrid trypsin–Na5Yb9F32 nanomaterials using trypsin as the bio template.
Field emission scanning electron microscopy (FESEM) and transmission electron microscopy (TEM) techniques were performed to determine the morphology and detailed structure of the hybrid trypsin–Na5Yb9F32 nanomaterials. SEM images showed that the nanomaterials exhibited a carnation-like nanoflower structure with a diameter of approximately 3 μm (Fig. 2a). The magnified SEM image of the hybrid nanoflowers indicated that they have a layered structure with a high surface area to volume ratio, which was assembled by individual nanoparticle units (Fig. 2b). The TEM image further showed that the Na5Yb9F32 crystals were assembled via an oriented attachment of primary nanoparticles (Fig. 2c). The HRTEM image showed the characteristic (111) lattice spacing (0.31 nm) of the rare earth fluoride Na5Yb9F32 crystals (Fig. 2d). The pattern of selected-area electron diffraction (SAED) indicated that the assembly of primary Na5Yb9F32 nanoparticles was quite oriented (Fig. 2d).
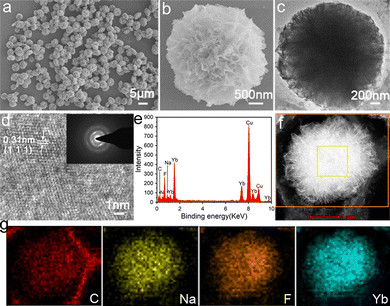 |
| Fig. 2 Electron microscopy characterization of the morphology of trypsin–Na5Yb9F32 hybrid nanomaterials. FESEM images of trypsin–Na5Yb9F32 nanoflowers prepared by the incubation of the mixture of trypsin (0.95 mg mL−1), Yb(NO3)3 (46 mM) and NaF (138 mM) at 25 °C for 48 h (a and b). TEM image (c), HRTEM image, SAED pattern (inset) (d), EDX spectrum (e), and HAADF-STEM image (f) of the trypsin–Na5Yb9F32 nanoflowers. (g) EDS mapping images of C, Na, F, and Yb for the square region of the Na5Yb9F32 particles in (f). | |
The presence of C, N, Na, Yb and F was evident in the energy dispersive X-ray (EDX) analysis of hybrid nanomaterials (Fig. 2e). The high angle annular dark field scanning TEM (HAADF-STEM) image indicated that the primary Na5Yb9F32 nanoparticles gradually aggregated to form hierarchical flower-like mesocrystals (Fig. 2f). The corresponding element mapping results of energy dispersive X-ray spectroscopy (EDS) further showed that the C, Na, F and Yb elements were evenly distributed on the hybrid nanoflowers (Fig. 2g). In addition, the Brunauer–Emmett–Teller (BET) surface area and average pore size of the Na5Yb9F32 mesocrystals were calculated using the Barrett–Joyner–Halenda (BJH) model to be 86.8 m2 g−1 and 231.0 Å, respectively (Fig. S1, ESI†). It is demonstrated that the Na5Yb9F32 mesocrystals displayed a porous structure with a high surface-to-volume ratio. All the results indicated that the trypsin-templated biomineralization process resulted in Na5Yb9F32 mesocrystals with elegant flower-like morphology.
Time-dependent evolution of trypsin–Na5Yb9F32 hybrid nanoflowers
The time-dependent evolution of trypsin–Na5Yb9F32 hybrid nanoflowers was monitored by SEM and XRD to investigate the underlying mechanism of biomineralization of Na5Yb9F32 crystals using trypsin as the template (Fig. 3 and Fig. S2, ESI†). A mixture of trypsin (0.95 mg mL−1), Yb(NO3)3 (46 mM) and NaF (138 mM) was incubated at 25 °C. SEM images as a function of time suggested the following formation mechanism of trypsin–Na5Yb9F32 hybrid nanoflowers (Fig. 3a). At the early growth step, Yb(III) ions formed a complex with trypsin probably through the interaction of charged amino acids, leading to the production of primary crystals (Fig. 3b and c). At the secondary growth step, the primary Na5Yb9F32 crystals self-assembled to form nanoflowers with small petals, while trypsin probably covered the surface and stabilized the primary crystals (Fig. 3d and e). At the final anisotropic growth step, the nanoparticles continued the oriented-assembly to build hierarchical flower-like mesocrystals with branched petals (Fig. 3f and g). In this time-dependent biomineralization process for the production of hybrid nanoflowers, trypsin played a key role by guiding the nucleation of Na5Yb9F32 to form a well-ordered scaffold and gluing the petals together (Fig. 3a). Meanwhile, the nanomaterials obtained at different times of incubation (5 min, 30 min, 3 h, 5 h, 12 h and 36 h) showed the same XRD pattern assigned to pure Na5Yb9F32 crystals (JCPDS No. 27-1426) (Fig. S2, ESI†). This indicated that the mixture formed primary Na5Yb9F32 crystals at the initial stage and kept the same crystal phase during the growth of trypsin–Na5Yb9F32 hybrid nanoflowers.
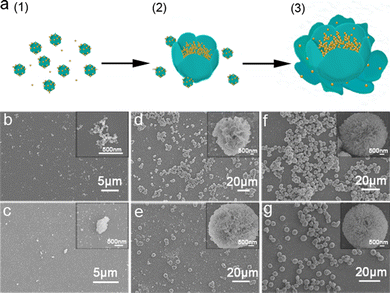 |
| Fig. 3 (a) Schematic representation of the formation mechanism of trypsin–Na5Yb9F32 hybrid nanoflowers. FESEM images of trypsin–Na5Yb9F32 hybrid nanomaterials prepared at different incubation times: 5 min (b), 30 min (c), 3 h (d), 5 h (e), 12 h (f) and 36 h (g). | |
In order to examine the critical role of trypsin in the generation of hybrid nanoflowers, the morphologies of trypsin–Na5Yb9F32 hybrid nanomaterials were measured after treatments with calcination and glutaraldehyde/EDTA (Fig. S3, ESI†). Calcination of the as-prepared hybrid nanomaterials at 350 °C for 6 h resulted in the disruption of flower-like structures, suggesting that trypsin was a key factor to hold the hierarchical structure (Fig. S3a, ESI†). When the hybrid nanomaterials were first cross-linked by glutaraldehyde and then treated with ethylenediaminetetraacetic acid (EDTA) to remove the Yb3+ ions, the exquisite nanoflower morphology was also destroyed, suggesting that the Yb3+ ions were also critical in the formation of nanoflowers (Fig. S3b, ESI†). These results demonstrated that both trypsin and Yb3+ ions were evenly distributed in the hybrid nanomaterials, and they collectively regulated the morphology of hybrid nanoflowers.
Universality of protein–Na5Yb9F32 nanoflowers
In order to evaluate the general applicability of the production of Na5Yb9F32 crystals using proteins as the template, different types of proteins were used for the biomineralization process and the morphology of the as-prepared hybrid nanomaterials was characterised by SEM (Fig. 4). In the absence of proteins, the synthesised nanomaterials formed small nanospheres with a diameter of approximately 350 nm (Fig. 4a). Collagen and FtsZ were prepared by recombinant methods as previously described.42–44 When BSA, collagen or FtsZ was added, the generated nanomaterials displayed well-ordered flower-like nanostructures. In summary, different types of proteins could be used as bio templates to create hybrid protein–Na5Yb9F32 hybrid nanoflowers.
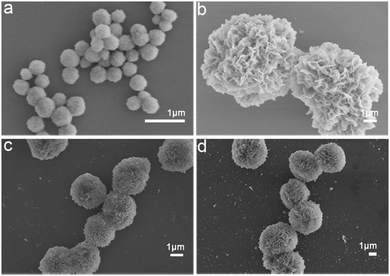 |
| Fig. 4 FESEM images of protein–Na5Yb9F32 hybrid nanomaterials prepared by using different types of proteins as the biotemplates: (a) no protein; (b) BSA; (c) collagen; and (d) FtsZ. | |
Enzymatic activity of trypsin–Na5Yb9F32 hybrid nanoflowers
The enzymatic activity of trypsin–Na5Yb9F32 hybrid nanoflowers and free trypsin was evaluated using the Folin assay. Urea and ACN are widely used to denature native proteins in proteomics experiments. Therefore, different concentrations of urea and ACN were added to the casein solution to investigate the performance of trypsin–Na5Yb9F32 and free trypsin under denaturing conditions (Fig. 5a and b). When compared to free trypsin, trypsin–Na5Yb9F32 nanomaterials displayed higher relative activity in the presence of a large amount of urea or ACN (Fig. 5a and b). This indicated that trypsin–Na5Yb9F32 had high tolerance to denaturing conditions.
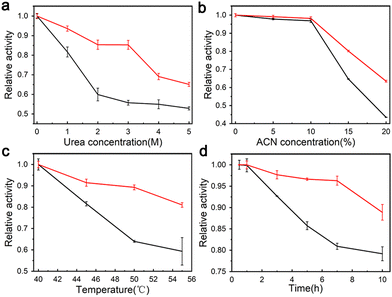 |
| Fig. 5 Enzymatic activity of trypsin–Na5Yb9F32 hybrid nanoflowers (red) and free trypsin (black) under different conditions. Enzymatic activity was measured under denaturing conditions by adding various concentrations of urea (a) and acetonitrile (ACN) (b). Enzymatic activity was determined at different incubation temperatures (c) and different incubation times at 55 °C (d). | |
The enzymatic activity of trypsin–Na5Yb9F32 nanoflowers and free trypsin was measured at various temperatures to evaluate their thermal stability. The activities of trypsin–Na5Yb9F32 and free trypsin at different temperatures were normalised to that at 40 °C as relative activity for comparison. When the temperature was higher than 40 °C, the synthesised trypsin–Na5Yb9F32 nanoflowers possessed a much higher relative activity than free trypsin, indicating high tolerance of trypsin–Na5Yb9F32 towards inactivation due to high temperature (Fig. 5c).
The enzymatic activity of trypsin–Na5Yb9F32 nanoflowers and free trypsin was also real-time monitored at 55 °C (Fig. 5d). The activity of free trypsin was significantly reduced after 3 h of incubation, while the trypsin–Na5Yb9F32 nanoflowers always maintained much higher enzymatic activity during the whole time of incubation, indicating high heat resistance of trypsin–Na5Yb9F32 (Fig. 5d). These results indicated that the trypsin–Na5Yb9F32 nanoflowers possessed good stability against thermal and chemical denaturation. It may be due to the increased stability of immobilised enzymes on the metal surface and enhanced mass transfer due to the high surface areas of nanoflowers.
Reusability of trypsin–Na5Yb9F32 hybrid nanoflowers
The reusability of trypsin–Na5Yb9F32 hybrid nanoflowers was investigated using recombinant collagen V-CL, a good model system for visualizing the enzymatic cleavage activity of trypsin. The V-domain is easily digested by trypsin when it is below the melting temperature of V-CL (37 °C), whereas the CL domain is resistant to trypsin cleavage. Therefore, the digested product of V-CL showed a single band of CL in SDS-PAGE, making it a convenient method for detecting the activity and reusability of trypsin–Na5Yb9F32. SDS-PAGE experiments showed that the V-CL protein from the control sample migrated to a single band around 45 kDa, whereas the addition of trypsin–Na5Yb9F32 caused the digested product of CL to migrate as another single band, demonstrating that V-CL was completely digested by trypsin-Na5Yb9F32 (Fig. 6). After repeated use of 8 cycles, the trypsin–Na5Yb9F32 hybrid nanoflowers maintained similarly high activity to completely cleave V-CL (Fig. 6). In conclusion, trypsin–Na5Yb9F32 hybrid nanoflowers had excellent reusability, which was one of the most favoured properties of immobilised enzymes.
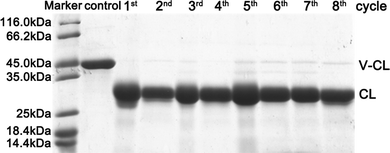 |
| Fig. 6 SDS-PAGE analysis of the repeated use of trypsin–Na5Yb9F32 hybrid nanoflowers towards the cleavage of recombinant collagen (V-CL). | |
Photoluminescence of trypsin–Na5Yb9F32 hybrid nanoflowers
Lanthanide ion-doped luminescent materials have been widely used in bioimaging and optoelectronics because of their low toxicity, large Stokes shift, and strong resistance to photodegradation. The photoluminescence properties of trypsin–Na5Yb9F32 hybrid nanoflowers doped with Tb3+ and Eu3+ were characterised using a solid state fluorescence spectrometer (Fig. 7). A mixture of trypsin (0.95 mg mL−1), Yb(NO3)3 (46 mM), NaF (138 mM) and Tb(NO3)3 (or Eu(NO3)3, 5 mM) was incubated at 25 °C for 12 hours to obtain trypsin–Na5Yb9F32:Tb3+ and trypsin–Na5Yb9F32:Eu3+ nanoflowers, respectively. The SEM images of the doped nanopowders indicated that the addition of Eu or Tb does not affect the final morphology and the doped samples have the same flower-like morphology as undoped trypsin–Na5Yb9F32. The emission spectrum of the as-prepared Tb-doped trypsin–Na5Yb9F32 nanoflowers at an excitation wavelength of 369 nm showed a strong green emission at ∼543 nm along with other weak emission lines which can be attributed to intra-configurational f–f transitions of Tb3+ ions. The observed emission lines at 490, 543, 588 and 620 nm were ascribed to the intra-4f8 transitions from the 5D4 energy level to 7F6, 7F5, 7F4 and 7F3 energy levels, respectively.46 Similarly, Fig. 7b shows the room-temperature photoluminescence emission spectrum of Eu-doped trypsin–Na5Yb9F32 nanoflowers under 369 nm laser excitation. It displayed multiple emission peaks at 593, 614, 650 and 691 nm ascribed to the intra-4f6 transitions from the lowest excited 5D0 level to the 7F1, 7F2, 7F3 and 7F4 levels, respectively.47–50 These results showed that the protein–inorganic Na5Yb9F32 nanomaterials displayed good photoluminescence features and their colours could be easily adjusted by using different kinds of lanthanide ions.
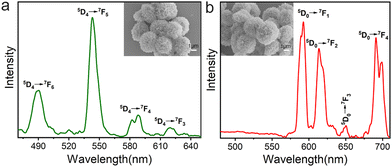 |
| Fig. 7 Emission spectra of luminescent materials of trypsin–Na5Yb9F32 doped with specified lanthanide ions: (a) Tb3+ and (b) Eu3+. The emission spectra were recorded at λex = 369 nm and λex = 394 nm for Tb3+ and Eu3+, respectively. SEM images of the doped hybrid nanomaterials are shown in the insets. | |
Conclusions
Nanoflowers are a novel type of nanoparticles with superior features such as high surface area and strong adsorption capacity and have attracted increasing attention in the fields of catalysis, biosensors, and medicine. Rare earth fluoride nanoparticles display fabulous advantages such as low phonon energies and high chemical stability and have been extensively used in biosensing and photovoltaic devices. However, the fabrication of rare earth fluoride nanoparticles with flower-like morphology remains an unmet demand.
We have for the first time developed well-ordered protein–rare earth fluoride nanoflowers using trypsin as the unique template for biomineralization. The XRD and XPS graphs indicated that pure Na5Yb9F32 rare earth fluoride crystals were achieved via this trypsin-templated biomineralization process, while the SEM and TEM images demonstrated that the as-prepared Na5Yb9F32 mesocrystals possess exquisite flower-like architecture. The FT-IR and TGA characterization confirmed the encapsulation of trypsin in the Na5Yb9F32 mesocrystals. The time-dependent evolution of trypsin–Na5Yb9F32 hybrid nanoflowers suggested that trypsin played a vital role by guiding the nucleation of Na5Yb9F32 to form a well-ordered scaffold and cohering the petals together.
Enzymatic studies indicated that in contrast to free trypsin, the trypsin–Na5Yb9F32 nanoflowers display excellent stability against thermal and chemical denaturation. Notably, the immobilized trypsin has superior re-usability. Furthermore, the trypsin–Na5Yb9F32 nanomaterials display excellent photoluminescence features and their colours could be easily tuned by using different kinds of lanthanide ions. The one-pot environmentally amiable biomineralization approach leads to the production of multifunctional trypsin–Na5Yb9F32 hybrid nanoflowers combining the attractive optical and catalytic features of rare earth fluorides and trypsin, respectively.
Different proteins such as BSA, collagen and FtsZ have all shown remarkable capability to create flower-like nanoparticles, suggesting that protein-templated biomineralization provides a universal and robust approach for the synthesis of protein–rare earth fluoride hybrid nanoflowers. The novel luminescent protein–rare earth fluoride nanoflowers may have great potential in biosensors, industrial biocatalysis and medical diagnostic technologies.
Conflicts of interest
The authors declare no competing conflicts of interest.
Acknowledgements
This work was supported by grants from the National Natural Science Foundation of China (grant no. 22074057 and 21775059) and the Natural Science Foundation of Gansu Province (grant no. 20YF3FA025 and 18YF1NA004).
References
- J. Zeng and Y. Xia, Nat. Nanotechnol., 2012, 7, 415–416 CrossRef CAS PubMed.
- J. L. Wang, X. Q. You, C. Xiao, X. P. Zhang, S. H. Cai, W. L. Jiang, S. S. Guo, S. H. Cao and Z. Chen, Appl. Catal., B, 2019, 259, 118060 CrossRef CAS.
- C. C. Zhai, L. Y. Miao, Y. B. Zhang, L. Q. Zhang, H. Li and S. X. Zhang, Chem. Eng. J., 2022, 431, 134107 CrossRef CAS.
- T. Yin, Y. J. Li, K. X. Bian, R. Y. Zhu, Z. W. Liu, K. Niu, H. Liu, Z. R. Gao and D. W. Gao, Mater. Sci. Eng., C, 2018, 93, 716–723 CrossRef CAS PubMed.
- L. C. Palmer, C. J. Newcomb, S. R. Kaltz, E. D. Spoerke and S. I. Stupp, Chem. Rev., 2008, 108, 4754–4783 CrossRef CAS PubMed.
- P. Fratzl, O. Kolednik, F. D. Fischer and M. N. Dean, Chem. Soc. Rev., 2016, 45, 252–267 RSC.
- L. B. Gower, Chem. Rev., 2008, 108, 4551–4627 CrossRef CAS PubMed.
- J. Ge, J. D. Lei and R. N. Zare, Nat. Nanotechnol., 2012, 7, 428–432 CrossRef CAS PubMed.
- L. Zhu, L. Gong, Y. F. Zhang, R. Wang, J. Ge, Z. Liu and R. N. Zare, Chem. – Asian J., 2013, 8, 2358–2360 CrossRef CAS PubMed.
- J. Y. Sun, J. C. Ge, W. M. Liu, M. H. Lan, H. Y. Zhang, P. F. Wang, Y. M. Wang and Z. W. Niu, Nanoscale, 2014, 6, 255–262 RSC.
- Z. Lin, Y. Xiao, L. Wang, Y. Q. Yin, J. N. Zheng, H. H. Yang and G. N. Chen, RSC Adv., 2014, 4, 13888–13891 RSC.
- Z. Lin, Y. Xiao, Y. Q. Yin, W. L. Hu, W. Liu and H. H. Yang, ACS Appl. Mater. Interfaces, 2014, 6, 10775–10782 CrossRef CAS PubMed.
- L. B. Wang, Y. C. Wang, R. He, A. Zhuang, X. P. Wang, J. Zeng and J. G. Hou, J. Am. Chem. Soc., 2013, 135, 1272–1275 CrossRef CAS PubMed.
- X. L. Wang, J. F. Shi, Z. Li, S. H. Zhang, H. Wu, Z. Y. Jiang, C. Yang and C. Y. Tian, ACS Appl. Mater. Interfaces, 2014, 6, 14522–14532 CrossRef CAS PubMed.
- Z. H. Zhang, Y. C. Zhang, R. R. Song, M. H. Wang, F. F. Yan, L. H. He, X. Z. Feng, S. M. Fang, J. H. Zhao and H. Z. Zhang, Sens. Actuators, B, 2015, 211, 310–317 CrossRef CAS.
- R. Hu, X. B. Zhang, Z. L. Zhao, G. Z. Zhu, T. Chen, T. Fu and W. H. Tan, Angew. Chem., Int. Ed., 2014, 53, 5821–5826 CrossRef CAS PubMed.
- J. F. Shi, S. H. Zhang, X. L. Wang, C. Yang and Z. Y. Jiang, J. Mater. Chem. B, 2014, 2, 4289–4296 RSC.
- K. Li, J. H. Wang, Y. J. He, M. A. Abdulrazaq and Y. J. Yan, J. Biotechnol., 2018, 281, 87–98 CrossRef CAS PubMed.
- G. K. Kouassi, J. Irudayaraj and G. McCarty, J. Nanobiotechnol., 2005, 3, 1 CrossRef PubMed.
- R. S. Prakasham, G. S. Devi, C. S. Rao, V. S. S. Sivakumar, T. Sathish and P. N. Sarma, Appl. Biochem. Biotechnol., 2010, 160, 1888–1895 CrossRef CAS PubMed.
- S. A. Ansari and Q. Husain, Biotechnol. Adv., 2012, 30, 512–523 CrossRef CAS PubMed.
- J. Njagi and S. Andreescu, Biosens. Bioelectron., 2007, 23, 168–175 CrossRef CAS PubMed.
- J. H. Lin, W. Qu and S. S. Zhang, Anal. Biochem., 2007, 360, 288–293 CrossRef CAS PubMed.
- Y. W. Zhang, Y. Zhang, H. Wang, B. Yan, G. L. Shen and R. Q. Yu, J. Electroanal. Chem., 2009, 627, 9–14 CrossRef CAS.
- P. Takhistov, Biosens. Bioelectron., 2004, 19, 1445–1456 CrossRef CAS PubMed.
- R. X. Wang, Z. G. Tian and L. Y. Chen, Int. J. Pharm., 2011, 406, 153–162 CrossRef CAS PubMed.
- P. S. Kumar, S. Ramakrishna, T. R. Saini and P. V. Diwan, Pharmazie, 2006, 61, 613–617 CAS.
- P. Wanakule, G. W. Liu, A. T. Fleury and K. Roy, J. Controlled Release, 2012, 162, 429–437 CrossRef CAS PubMed.
- J. K. Kim, J. Anderson, H. W. Jun, M. A. Repka and S. Jo, Mol. Pharmaceutics, 2009, 6, 978–985 CrossRef CAS PubMed.
- K. J. McHugh, L. H. Jing, S. Y. Severt, M. Cruz, M. Sarmadi, H. S. N. Jayawardena, C. F. Perkinson, F. Larusson, S. Rose, S. Tomasic, T. Graf, S. Y. Tzeng, J. L. Sugarman, D. Vlasic, M. Peters, N. Peterson, L. Wood, W. Tang, J. Yeom, J. Collins, P. A. Welkhoff, A. Karchin, M. Tse, M. Y. Gao, M. G. Bawendi, R. Langer and A. Jaklenec, Sci. Transl. Med., 2019, 11, eaay7162 CrossRef CAS PubMed.
- W. G. J. H. M. van Sark, J. de Wild, J. K. Rath, A. Meijerink and R. E. I. Schropp, Nanoscale Res. Lett., 2013, 8, 81 CrossRef PubMed.
- Y. T. Zhong, Z. R. Ma, F. F. Wang, X. Wang, Y. J. Yang, Y. L. Liu, X. Zhao, J. C. Li, H. T. Du, M. X. Zhang, Q. H. Cui, S. J. Zhu, Q. C. Sun, H. Wan, Y. Tian, Q. Liu, W. Z. Wang, K. C. Garcia and H. J. Dai, Nat. Biotechnol., 2019, 37, 1322 CrossRef CAS PubMed.
- D. D. Zhang, L. W. Wen, R. Huang, H. H. Wang, X. L. Hu and D. Xing, Biomaterials, 2018, 153, 14–26 CrossRef CAS PubMed.
- T. Grzyb and D. Przybylska, Inorg. Chem., 2018, 57, 6410–6420 CrossRef CAS PubMed.
- H. L. Qiu, C. H. Yang, W. Shao, J. Damasco, X. L. Wang, H. Agren, P. N. Prasad and G. Y. Chen, Nanomaterials, 2014, 4, 55–68 CrossRef PubMed.
- M. L. Tan, B. del Rosal, Y. Q. Zhang, E. M. Rodriguez, J. Hu, Z. G. Zhou, R. W. Fan, D. H. Ortgies, N. Fernandez, I. Chaves-Coira, A. Nunez, D. Jaque and G. Y. Chen, Nanoscale, 2018, 10, 17771–17780 RSC.
- Q. G. Meng, R. J. Witte, P. S. May and M. T. Berry, Chem. Mater., 2009, 21, 5801–5808 CrossRef CAS.
- V. Sudarsan, S. Sivakumar, F. C. J. M. van Veggel and M. Raudsepp, Chem. Mater., 2005, 17, 4736–4742 CrossRef CAS.
- T. Aubert, F. Grasset, S. Mornet, E. Duguet, O. Cador, S. Cordier, Y. Molard, V. Demange, M. Mortier and H. Haneda, J. Colloid Interface Sci., 2010, 341, 201–208 CrossRef CAS PubMed.
- P. H. Mutin and A. Vioux, Chem. Mater., 2009, 21, 582–596 CrossRef CAS.
- X. Wang, J. Zhuang, Q. Peng and Y. D. Li, Inorg. Chem., 2006, 45, 6661–6665 CrossRef CAS PubMed.
- A. Yoshizumi, Z. Yu, T. Silva, G. Thiagarajan, J. A. Ramshaw, M. Inouye and B. Brodsky, Protein Sci., 2009, 18, 1241–1251 CrossRef CAS PubMed.
- J. C. Munyemana, H. He, S. Ding, J. Yin, P. Xi and J. Xiao, RSC Adv., 2018, 8, 2708–2713 RSC.
- F. Yang, S. Zhang, S. Ding, Y. Hou, L. Yu, X. Chen and J. Xiao, Int. J. Biol. Macromol., 2016, 91, 294–298 CrossRef CAS PubMed.
- X. Sun, X. Cai, R. Q. Wang and J. Xiao, Anal. Biochem., 2015, 477, 21–27 CrossRef CAS PubMed.
- H. Lai, A. Bao, Y. Yang, Y. Tao, H. Yang, Y. Zhang and L. Han, J. Phys. Chem. C, 2008, 112, 282–286 CrossRef CAS.
- S. Rodriguez-Liviano, F. J. Aparicio, T. C. Rojas, A. B. Hungría, L. E. Chinchilla and M. Ocaña, Cryst. Growth Des., 2012, 12, 635–645 CrossRef CAS.
- H. Guo, F. Li, J. Li and H. Zhang, J. Am. Ceram. Soc., 2011, 94, 1651–1653 CrossRef CAS.
- X. Yang, X. Dong, J. Wang and G. Liu, Mater. Lett., 2009, 63, 629–631 CrossRef CAS.
- S. Majeed, M. Bashir and S. Shivashankar, J. Nanopart. Res., 2015, 17, 1–15 CrossRef CAS.
|
This journal is © The Royal Society of Chemistry 2023 |
Click here to see how this site uses Cookies. View our privacy policy here.