DOI:
10.1039/D3ME00002H
(Review Article)
Mol. Syst. Des. Eng., 2023,
8, 1097-1129
Stimuli-responsive structure–property switchable polymer materials
Received
6th January 2023
, Accepted 20th March 2023
First published on 23rd March 2023
Abstract
The development of polymeric materials with switchable properties upon exposure to external stimuli such as light, heat, force, acids/bases, and chemicals has attracted much attention due to their potential applications in smart coatings, drug carriers, soft robotics, etc. Thus, a systematic understanding of the relationship between the chemical structures and stimuli-responsive properties as well as the corresponding mechanisms of switchable stimuli-responsive polymers is important to guide the sophisticated design of functional macromolecules for specific applications. In this review, we outline the representative chemical structures that enable reversible structural switching to be achieved under photo-, mechano-, CO2- and chemo-stimuli, with a brief discussion on thermo- and pH-activation. Polymer materials containing these chemical moieties exhibit unique behaviours on changing their mechanical, thermal or photochemical property in a switchable manner, and their applications in healing materials, rewritable surfaces, drug delivery carriers, conductive hydrogels and luminescent materials are highlighted. Based on the comprehensive summary of structural switchable moieties and applications of polymer materials, this review gives insight into how bottom-up approaches can be used to inspire the future design of switchable multi-stimuli-responsive polymer materials.
Design, System, Application
Stimuli-responsive polymers are promising materials whose properties can be controllably and predictably changed by external stimuli and have a wide range of applications including molecular sensing, drug carriers, coatings, artificial muscles, and thermoelectric materials. At the heart of these materials are the moieties that alter their chemical structure in response to an external stimuli, resulting in a change in macroscopic properties such as colour, morphology, and phase transition temperature. This review covers the design of polymers responsive to six different stimuli (light, pH, thermal, force, CO2, and redox), with a section regarding polymers responsive to multiple stimuli also included. This review is structured according to the key enabling functional groups and discusses tuning of the properties of polymers by changes in their position or chemical structure. We believe that this class of polymers will see greater utilization with the maturity of this field, with a more extensive library of stimuli-responsive moieties.
|
1 Introduction
In the past two decades, major efforts have been devoted to creating on-demand stimuli-responsive materials to mimic natural systems.1–4 Stimuli-responsive polymers are a class of promising “smart” materials that are capable of altering their properties when triggered by external stimuli in a controllable manner.1,5–8 Depending on the polymer structure, the triggering stimuli can be physical (temperature, light, mechanical force, magnetic field, electric interaction, etc.), chemical (pH, redox, molecules, supramolecular interaction, etc.) or biological (enzyme, etc.). Due to their versatile stimuli-responsive functions and cost-effective preparation, smart polymer materials, such as nanoparticles, films and hydrogels, are attracting great interest for extensive applications such as drug carriers, coatings, molecular sensing, artificial muscles, and thermoelectric materials.9–14
Among the stimuli-responsive polymers, reversibly switchable smart polymer materials and surfaces can achieve reversible “on–off” switching to tune their structures and properties several times.9,15,16 In nature, several creatures, e.g., cuttlefish, chameleon and gray treefrog, are masters at reversibly changing their appearance such as colour and pattern in response to their surrounding environment.17–19 These reversible transformation features can be attributed to pigment translocation or molecular switch in their chromatophores, allowing them to mimic their background environments. Additionally, biopolymers such as proteins and nucleic acids extensively exist in living organic systems and undergo conformational changes to modulate and maintain physiological parameters. Consequently, these stimuli-responsive creatures and biomacromolecules have inspired scientists to develop various synthetic polymeric materials to mimic their adaptive behaviours.20–22
Although pigment translocation still lacks applications in materials science, molecular switching is a promising and effective approach to endow materials with switchable physical and chemical properties. Many reversibly switchable molecules, such as photochromic molecules, host–guest molecule pairs, and mechanically interlocked molecules, have been developed and found many applications.23–28 In recent years, design at the molecular level by exploiting reversibly switchable molecules has enabled the synthesis of functional monomers, and then polymers to produce novel polymeric materials with unique stimuli-responsive behaviors.9,10,16 Many applications of reversibly switchable polymers have been found to control the appearance of materials or serve as a release system.
Herein, we attempt to summarize the numerous stimuli-responsive building units and how they alter their conformation or geometry reversibly in a polymer chain, and subsequently used to construct switchable polymer materials with stimuli-responsive functional groups and their reversibly conformational or geometric changes in a polymer chain to construct smart materials with switchable properties. Beyond the well-studied reversible phase transition behaviors via intermolecular hydrophobic interactions (e.g., lower critical solution temperature (LCST) and upper critical solution temperature (UCST)) or supramolecular interactions, polymers with reversibly switchable chemical structures through isomerization, cyclization, ionization and bond cleavage are highlighted. Furthermore, structure-switchable functional groups triggered by various stimuli, including photo, mechanical, carbon dioxide (CO2) and redox, are catalogued to express their structure transformation mechanisms, while thermal- and acidic/basic (pH)-responsive polymers will be briefly discussed. Subsequently, polymeric materials containing switchable functional groups are introduced to demonstrate their state-of-the-art applications and structure–property relationships. Examples of the combination of different stimuli-responsive functional groups to develop dual- or multiple-stimuli-responsive polymeric materials and surfaces are also introduced. Finally, we address the remaining challenges and outlook for the future development of reversibly switchable smart polymeric materials.
2 Polymers with switchable structures and their applications
2.1 Photo-responsive polymers
As representative polymers,29,30 photo-responsive polymers are polymers that can respond to light, leading to a reversible change in either their conformation or chemical structure. They have a variety of potential applications ranging from drug delivery to actuators and self-healing materials. Some of the key photo-responsive chemical structures include azobenzene, diarylethenes, spiropyrans and disulfides. These moieties impart different photo-responsive properties to polymers. For instance, cis–trans isomerism can modulate the crystallinity and glass transition temperature (Tg) of polymers. Meanwhile, diarylethenes and spiropyrans can change their colour and fluorescence. In this section, we review photo-responsive polymers with emphasis on how changes in their chemical structure modulate their properties.
2.1.1 Azobenzene.
The most common light-responsive moiety is azobenzene. Its cis isomer and photo-induced conversion were reported in 1937,31 and subsequently extensive research has focused on its properties and applications.32Trans azobenzene, the thermally favoured isomer, is converted to cis azobenzene upon exposure to UV irradiation, while the reverse can be mediated by exposure to either visible light or thermally in the dark (Fig. 1a).33 It has been found that this process is highly sensitive, and both its rate and quantum yield can be influenced by the solvent, temperature, substituents on the phenyl ring, and irradiation wavelength. As many as four different mechanisms have been postulated (rotation, inversion, concerted inversion, and inversion-assisted rotation), with multiple pathways being used to explain empirical observations.34 More recently, progress has also been made in achieving trans to cis conversion via visible light irradiation, either by direct functionalisation of the azobenzene ring,35,36 extended conjugation,37 or photo upconversion strategies.38 This has enabled photo isomerism to be achieved without exposure to harmful UV rays, which is particularly important in the case of biological applications. Azobenzene and its derivatives are also often used because they are stable and do not exhibit significant side reactions.39 Some of the azo-benzene containing polymers are shown in Fig. 1b.
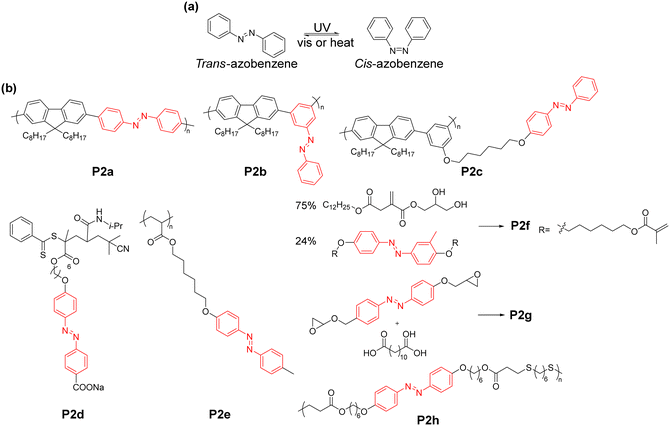 |
| Fig. 1 (a) Cis and trans isomers of azobenzene. (b) Examples of polymers containing azobenzene units. | |
The cis–trans transition of azobenzene has been used to modulate the fluorescence of polymers. Kuehne's group reported the preparation of a series of light-modulated monodisperse conjugated polymer particles.40 Azobenzene was conjugated with fluorene via Suzuki–Miyaura polymerisation and grafted on a poly(vinylpyrrolidone-co-vinyl acetate) (PVPVA) nucleus (P2a–c). In the case of P2a, given that the trans to cis switching occurs along the polymer backbone, large-scale motion is required. In contrast, for both P2b and P2c, the trans to cis switching only resulted in movement of their side chain, while P2c is completely non-conjugated with the polymer backbone as the azobenzene moiety is separated from the polymer backbone by the alkyl chain. Upon exposure to UV, the fluorescence of all three polymers shifted from about 450 nm to 600 nm (Fig. 2a). This was attributed to the non-radiative decay in the trans-configuration, which is significantly reduced in the cis-configuration, although the mechanism was not fully understood.41 The fluorescence switching was significantly slower for P2a compared to P2b and P2c because the entire polymer backbone needed to move, which is due to the fact that the fluorescence switching of P2a is triggered by the conformation change of the entire P2a polymer backbone. Consequently, the cis to trans transition for P2a was not fully reversible. As another example, in the work by Ren and co-workers, they prepared an amphiphilic diblock copolymer, P2d.42 The fluorescence of the polymer was sensitive towards pH, temperature and UV. In its neutral and basic conditions, the fluorescence of the polymer increased upon exposure to UV light, corresponding to the photo-isomerism of the azobenzene from trans to cis. Alternatively, under acidic conditions, only a slight increase in fluorescence was observed. This was due to the highly aggregated structure under acidic conditions, which inhibited the trans to cis photo-isomerism.
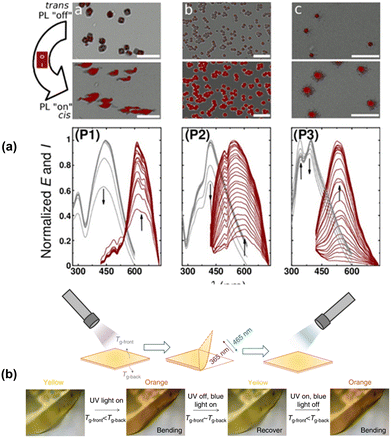 |
| Fig. 2 (a) Photoluminescence (PL) of P2a–c upon exposure to UV light.40 Reproduced from ref. 40 with permission from the American Chemical Society, Copyright 2013. (b) Actuation behavior of the acid-based azobenzene-containing epoxy polymer P2f.49 Reproduced from ref. 49 with permission. Copyright 2018, Nature Publishing Group. | |
In principle, the cis–trans transition can lead to a change in the melting point of azobenzene and the glass transition temperature (Tg) of polymers with azobenzene moieties. Although the melting points of the cis and trans isomers with unfunctionalized azobenzene only differed by 3 °C, (68 °C for trans and 71 °C for cis),43 polymers with azobenzene carrying long alkoxy chains can exhibit significantly higher differences in melting point, with photo-induced liquefaction observable.44 As early as the 1980s, the photo-responsive properties of azobenzene-containing polymers have been reported, and a change in viscosity upon irradiation was also noted upon exposure to UV light.45 However, a change in Tg upon irradiation has only recently been reported.
Wu's group also reported the preparation of an azobenzene-containing polymer P2evia reversible addition–fragmentation chain-transfer (RAFT) polymerization.46P2e was a hard solid at room temperature with a Tg of about 48 °C. Upon exposure to UV light, the surface of the polymer liquefied despite remaining at a temperature of only 28.5 °C. The Tg of the generated cis isomer was measured to be around −10 °C. Upon illumination with 530 nm visible light, the liquid polymer turned back into a solid, corresponding to the cis to trans isomerism. These types of materials can possibly be useful for repairable coatings or photo-switchable adhesives. However, at present, the liquefaction of bulk materials is still difficult to achieve due to the limited penetration of UV light (molar absorptivity of azobenzene is about 2.0 × 104 mol−1 L cm−1 at 365 nm).47 These polymers were also found to exhibit actuation properties, bending towards a UV light source, but returning to their original shape upon exposure to blue light.48
The cis–trans photo-isomerism of azobenzene can also produce mechanical responses. Koyama's group reported the preparation of a dodecyl glyceryl itaconate polymer crosslinked with a meta-methylazobenzene-containing moiety to form a liquid crystalline network, i.e., P2f.49 This polymer exhibited a Tg of 29 °C, which was reduced to 16 °C upon exposure to UV irradiation, with a corresponding decrease in Young's modulus from 140 MPa to 65.6 MPa. However, exposure to visible light restored the Tg within 2–4 seconds, enabling efficient photomechanical actuation. Exposure to UV light (354 nm) resulted in film bending towards the light, while exposure to blue light (465 nm) restored its flatness (Fig. 2b). The light intensity was also correlated with the bending speed. The mechanism of this bending was postulated to be due to the differences in the degree of absorption of photons between the front surface of the polymer and the back surface, which resulted in a difference in Tg between the front and the back of the film, with the inner strain causing the bending. As another example, in the work by Zhao and Xia, they prepared dodecanedioic acid-based epoxy networks, P2g.50 The networks were dynamic under high temperature in the presence of a catalyst, making them reprocessable and vitrimeric.51 The UV-induced torque could drive a cylinder and a “vehicle”, with no apparent degradation even after 100 rotations. Hayward's group also reported the preparation of an acrylate–thiol based polymer, P2h.52 Actuation behaviour was observed upon illumination with UV light even at 25 °C, but was found to be reversible upon exposure to green light only at temperatures above 60 °C.
2.1.2 Diarylethenes.
Photochromic diarylethenes (Fig. 3a), which were first reported in 1988,55 are photochromic molecules that can undergo 6π-electron cyclisation upon exposure to UV light and reversibly return to the initial open-ring isomer upon irradiation with visible light.56 Unlike azobenzene, this transition is usually thermally irreversible. The 2-aryl position is often substituted to prevent oxidative aromatisation. This transition often leads to significantly different frontier molecular orbital levels, resulting in a change in colour, fluorescence, and even electrical conductivity and electroluminescence in the case of conjugated polymers with such diarylethene units.57 Therefore, they have received significant attention in photo-tunable optoelectronics. Hetero-aryl groups, such as dithienylethenes, are also often used in place of phenyl rings to improve the stability of the closed ring form.58
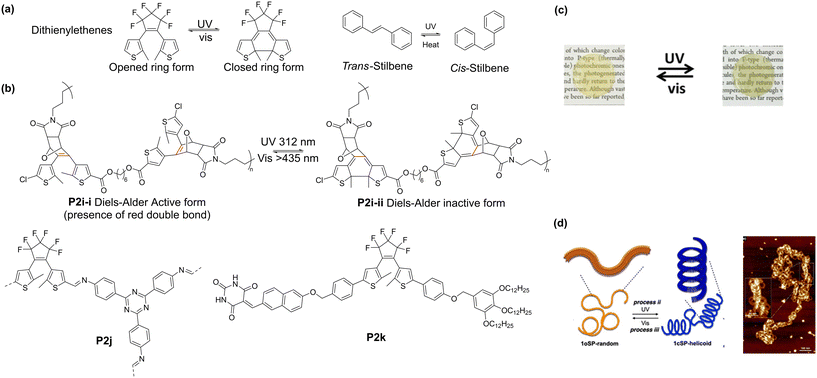 |
| Fig. 3 (a) Light-mediated ring opening and closing of diarylethenes and cis–trans isomerism of stilbene. (b) Examples of diarylethene-containing polymers. (c) Photochromic properties of P2j in PMMA matrix. Yellow (left image): ring-opened form. Green (right image): ring-closed form.53 Reproduced from ref. 53 with permission from The Royal Society of Chemistry, Copyright 2019. (d) Helical folding obtained by post-polymerization irradiation of P2k.54 Reproduced from ref. 54 with permission. Copyright 2021, the American Chemical Society. | |
The thermal stability of diarylethenes was used to control the reversibility of the Diels–Alder reaction in an adhesive material.59 Polymer P2i (Fig. 3b), was prepared by Diels–Alder reaction between the furan and the maleimide groups, forming a dithienylethene moiety. The Diels–Alder reaction was reversible by heating the polymer to 90 °C, resulting in a decrease in adhesion strength. However, upon exposure to 312 nm UV light, 6π-electron cyclisation occurring, resulting in the disappearance of the double bond of the diarylethene and preventing the occurrence of the reverse Diels–Alder reaction. Consequently, even when the polymer was heated to 90 °C, the adhesion strength did not decrease. This also demonstrated the thermal stability of the closed-ring configuration. Upon exposure to visible light (>435 nm), the reverse open-ring reaction occurred, allowing the reverse Diels–Alder reaction to proceed when heated.
Another application of photochromic diarylethenes is in photochromic films. Gu's group prepared an dithienyl-containing polyimine as a conjugated polymer network (P2j).53 The yellow polymer solid turned olive green upon exposure to UV light (365 nm) for 50 s, which was reversed by exposure to visible light (>500 nm) in about the same time. This is relatively fast compared to other polymers, which require more than 2 min to undergo the same change. Upon repeated cycling, a small amount of weight loss was observed (∼5%), but the overall physical structure of the polymer film was found to be stable. The composite films prepared by dispersing P2j in a PMMA matrix were found to undergo the same transition as the neat polymer (Fig. 3c). Another example of photochromic polymers was demonstrated by Bianco's group.60 Six different variations of diarylethenes were prepared to examine the effect of functionality, and combined with dicyclohexylmethane 4,4′-diisocyanate to form polyurethanes. Interestingly, a linear relationship was observed between the molar extinction coefficient and the peak wavelength of the closed-ring form. Recently, Yagai's group also demonstrated the potential of diarylethene to control the folding of polymers54 by making use of the difference in π–π stacking between the ring-closed and ring-opened form of diarylethene. Supramolecular polymers of P2k were prepared by cooling hot methylcyclohexane solution to obtain randomly coiled polymers. However, polymer P2k adopted a helical structure, as evidenced by the AFM imaging when the polymer was irradiated with UV light (293 nm), while upon cooling, AFM imaging showed that helical structures were adopted (Fig. 3d). In contrast, the polymer preferred a random supramolecular structure when exposed to visible light (620–645 nm).
Stilbenes are another class of diarylethenes that exhibit photo-responsiveness (Fig. 3a), though their mechanism differs from that of dithienylethenes given that they undergo trans to cis photo-isomerism similar to azobenzene. One example of this is in Harada's work, where they installed stilbene into a “daisy chain” crosslink.61 The trans to cis transition resulted in a significant change in polymer length, allowing for muscle-like contraction and expansion of the polymer, mediated by selective photoirradiation.
2.1.3 Spiropyran.
Photochromic spiropyrans, which were first reported by Fischer and Hirshberg in 1953,62 can cleave their C–O bond upon irradiation with UV to form a ring-opened merocyanine form (Fig. 4a).63 Besides a change in colour, this reversible transition upon exposure to visible light also results in a change in dipole, polarity, and even molecular volume.64 There is no conjugation between the two halves of the spiral given that they are orthogonal to each other. Consequently, the spiropyran form is neutral, non-polar and can absorb light typically in the UV region. Meanwhile, the merocyanine form is conjugated and has a significant dipole moment, which can be changed in response to pH. This merocyanine form is typically strongly coloured and can absorb light in the visible region.65 In addition, spiropyrans can also be responsive to redox and temperature changes depending on whether they contain any specific functionality.66
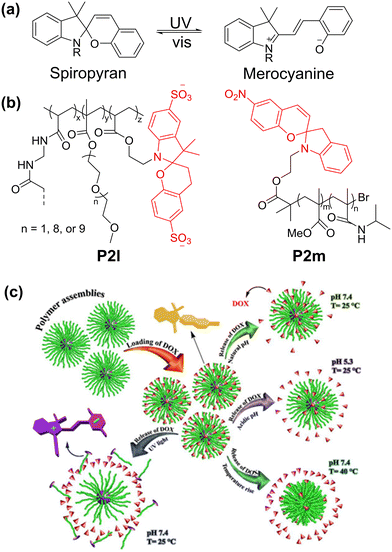 |
| Fig. 4 (a) Light-driven conversion between spiropyran and merocyanine forms. (b) Light-responsive spiropyran-containing polymers. (c) Effect of pH, UV and elevated temperature on release of doxorubicin from polymer P2k micelles.70 Reproduced from ref. 70 with permission. Copyright 2020, Elsevier. | |
Exploiting the difference in molecular volume and charge between the two forms of spiropyran, the light-driven expansion of hydrogels has been explored by Stupp's group.67 Sulfonate groups were included in spiropyran to increase its water solubility, while the methacrylate group was added to enable free radical polymerization. Subsequently, the spiropyran methacrylate was co-polymerized with oligo(ethylene glycol) methyl ether methacrylate and N,N′-methylenebis(acrylamide) as a crosslinker to form P2l (Fig. 4b). When irradiated with light, the charge density of the polymer increased, allowing water to enter the polymer network, and then swelled up to 30%. Other examples of spiropyran-based hydrogels demonstrating actuation include the work of Sumaru68 and Florea.69
Spiropyrans have also been explored in drug delivery systems. For instance, Salami-Kalajahi and Roghani-Mamaqani's group prepared a poly(methyl methacrylate)-b-poly(N-isopropylacrylamide) block copolymer with spiropyran as the end group (P2m).70 Doxorubicin (DOX, a chemotherapy drug) was loaded in the self-assembled P2m, and its release was examined under different pH values and UV irradiation. Less than 30% doxorubicin release was observed in neutral conditions, while 60–85% of DOX was found to be released in acidic and elevated temperature conditions (Fig. 4c). Alternatively, under UV irradiation, DOX release was found to be the fastest, with almost 100% release after 2 days, demonstrating the potential of light control for drug release. However, in this case, UV light as the trigger is suboptimal due to its lack of penetrative power, cytotoxicity and mutagenic effects on cells. A more elegant solution is the use of NIR up-conversion to provide stimuli for the ring opening. Some efforts have been reported making use of lanthanides nanoparticles to facilitate the up-conversion.71,72
2.1.4 C
C cis–trans photo-isomerisation.
Stereochemical control of polymers is a well-established approach towards controlling their properties. For instance, Hirabayashi prepared a succinic anhydride–propylene oxide polymer comprising predominantly Z isomer.73 When isomerised into the E form in the presence of morpholine, the polymer exhibited higher crystallinity, higher bio-degradability and higher Tg. However, unlike the well-documented cis–trans photo-isomerism of azobenzene, the corresponding cis–trans photo isomerism in C
C bonds is much less common. In this area, the common synthetic approach is co-polymerization and cyclization.74–76
In 2019, Lu′s group reported an example of cis–trans photo isomerism in polymers (Fig. 5).77 Ethylene oxide and maleic anhydride were co-polymerized to form P2n predominantly in the amorphous cis form. Upon treatment with diethylamine, the double bond isomerised to the more thermodynamically stable trans form, which was crystalline in nature. Subsequently, irradiation with UV light in the presence of benzophenone for 5 h resulted in about 30% conversion from the trans to cis isomer, accompanied by the corresponding loss of crystallinity. Another example in the same year is the work by Buchard's group.78 Polycarbonate P2o was prepared by ring-opening polymerization to obtain the predominantly cis form. This was isomerised into the more thermodynamically stable trans-form (52% after 68 h) upon exposure to UV light at room temperature in the presence of CuI. The trans isomer was a viscoelastic solid, while the cis isomer was a powder, demonstrating a practical approach to changing the properties of polymers by exposure to UV light. However, unlike the other polymers discussed, this isomerism has not been reported to be reversible.
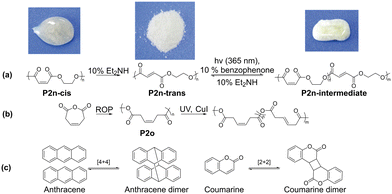 |
| Fig. 5 (a) Photo cis–trans isomerisation of polymers with corresponding modulation of their crystallinity.77 (b) Ring-opening polymerisation to form P2o, which could be photo-isomerised into the trans form. (c) Photodimerisation of anthracene and coumarin moieties. Reproduced from ref. 77 with permission. Copyright 2019, John Wiley and Sons. | |
2.1.5 Anthracene and coumarins.
Another mechanism of photo-responsive moieties is photodimerization such as that exhibited by anthracenes [4 + 4], and coumarins [2 + 2] (Fig. 5c). This mechanism is useful for controllable crosslinking of polymeric networks, such as in the work by Saito et al.,79 where they made use of anthracene to reversibly crosslink epoxy networks, enabling light-mediated healability. He's group also achieved similar results using coumarin as a photocrosslinker.80 Pleasingly, these photodimerization reactions can often be controlled by modulating the wavelength of the incident light, with longwave UV (∼355 nm) promoting the dimerization, while shortwave UV (∼265 nm) promotes bond cleavage.
2.1.6 Disulfide linkage.
Disulfides bonds are known to undergo homolytic photocleavage to form sulfenyl radicals, which is commonly exploited in dynamic covalent networks. Kessler's group prepared an epoxy network comprising 4,4-diglycidyloxyazobenzene, sebacic acid and 4,4-dithiodibutryic acid (P2p, Fig. 8).81 The resultant polymer exhibited a smectic liquid crystalline structure. The number of disulfide bonds was limited to just 10% to prevent the polymer from being over-dynamic, which reduced the stability of the liquid crystalline phase. The azobenzene moiety imparted photo-induced shape memory and actuation properties, while the disulfide bonds enabled photo-induced self-healing within 3 min (Fig. 8). In another example reported by Gautrot, disulfide bonds were used as a crosslinker to prepare a hyaluronic acid-based hydrogel.82 Similar to P2p, the polymer exhibited photo-induced self-healing, showing that the broken sample could be welded by adding a photo-initiator, followed by irradiation with UV light for 2 min. Some weakening of the material was observed compared to the pristine sample although the polymer was strong enough to be handled without special precautions (Fig. 6).
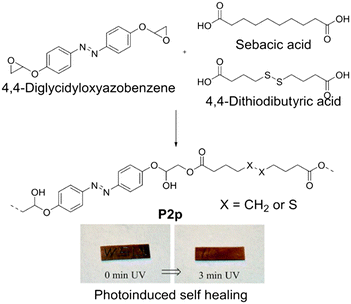 |
| Fig. 6 Disulfide-mediated self-healing of P2m. Reproduced from ref. 81 with permission. Copyright 2017, The Royal Society of Chemistry. | |
2.2 Force-responsive structure
In recent years, there has been a growing interest in polymers that can transduce mechanical inputs into specific chemical transformations. The resultant chemical responses can be categorised as either scissile (i.e., covalent bond-breaking reactions) or non-scissile (i.e., non-covalent bond disruptions and conformational changes) transformations.83 By tailoring the composition, types and positions of mechanophores (mechanically activated chemical groups) on the polymer chain, the mechanoresponsive behaviour of these materials can be programmed at a molecular level. In addition, the mechanochromic behaviour displayed by many mechanophores further highlights their potential to be used for the accurate detection of stress.84 In this section, we elaborate on the force-induced structural transformations of different chemical structures, including pyran derivatives, supramolecular assemblies, and other emerging structures.
2.2.1 Pyran derivatives.
Pyran derivatives, such as spiropyran and naphthopyran, are some of the most studied mechanophores. Through a 6-electron electrocyclic ring-opening reaction, the weak C–O bond in the pyran ring can be broken. This transforms the ring-closed pyrans to the ring-opened merocyanine, displaying mechanochromic behaviour in the process.87 Depending on the combination of the polymer chain attachment points and the nature of the substituents, the threshold force required to induce this transformation can be altered.88
Qiu et al. fabricated 6-nitro-spiropyran mechanophores with different attachment points (Fig. 7a).85 The threshold activations were found to occur at ∼60% strain and 4–7 MPa stress (Fig. 7b). This activation resulted in the appearance of absorption peaks at 500–650 nm, corresponding to the formation of merocyanine. A rapid decrease in absorption strength was recorded as the unloading of compressive strain gradually increased from 62%, showing that the polymers possessed a reversible mechanochromic response (Fig. 7c). Although both electronic and geometric effects influenced the mechanochromic behaviour of the mechanophores, the latter was found to be the dominant factor. Osler and co-workers investigated the mechanochemical reactivity of isomeric 2H- and 3H-naphthopyran mechanophores using solution-phase ultrasonication experiments and in crosslinked polydimethylsiloxane (PDMS) elastomers (Fig. 7d).89 The ring-opening reaction of the 2H-naphthopyran substrate was found to produce a red merocyanine dye (λmax = 485 nm), while the 3H-naphthopyran formed a yellow merocyanine (λmax = 440 nm) upon mechanical activation. The yellow dye in the 3H-naphthopyran polymer faded completely after 10 min of force removal, returning to the original colourless state. In contrast, the reddish colouration in the 2H-naphthopyran polymer was more persistent, taking a longer time to fade after the stress relaxation and still being visible in the pristine film (Fig. 7e), showing the greater stability of the merocyanine compound formed from the 2H-naphthopyran.
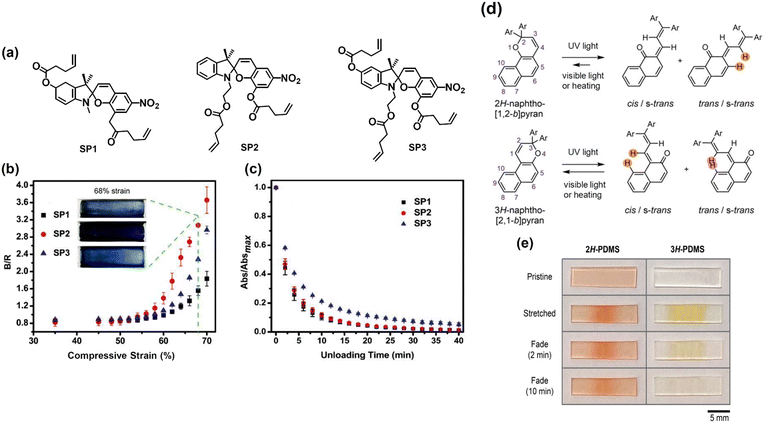 |
| Fig. 7 (a) Chemical structures of the 6-nitro-spiropyran mechanophores with differing attachment points. (b) Graph of B/R (blue channel intensity/red channel intensity) as a function of compressive strain for the various spiropyran samples. Insets: Photographs of the spiropyran samples at 68% strain. (c) Graph of Abs/Absmax (absorption intensity/maximum absorption intensity) as a function of unloading time in darkness from 62% compressive strain for the spiropyran samples. (d) Ring-opening reaction of the 2H- and 3H-naphthopyrans, forming merocyanine isomers. (e) Photographs of the 2H- and 3H-naphthopyran PDMS films immediately after being in tension and after subsequent stress relaxation. (a)–(c) are reproduced from ref. 85 with permission. Copyright 2019, The Royal Society of Chemistry. (d) and (e) are reproduced from ref. 86 with permission. Copyright 2021, Wiley. | |
2.2.2 Supramolecular assemblies.
Supramolecular assemblies, which consist of self-organising complexes held together by non-covalent bonds, often exhibit superior mechanoresponsive behaviour, with a high level of sensitivity to mechanical forces. In these assemblies, the breakage of the weak non-covalent bonds, e.g., van der Waals interactions, hydrogen bonding, and π-stacking interactions, between complexes triggers a structural transformation. Compared to other mechanoresponsive polymers, which rely on the breakage of covalent bonds in the mechanophores, supramolecular assemblies are advantageous in being more sensitive due to their lower activation energy as well as having generally higher reversibility.88,90–93
Sagara's group investigated the mechanoresponsive behaviour of rotaxane-based supramolecular mechanophores.94 The rotaxane mechanophores were composed of a luminophore-containing ring, which was threaded onto an axle with a matching quencher and two stoppers (Fig. 8a). Reversible fluorescence changes were observed even after 50 loading and unloading cycles due to the molecular shuttling of the mechanophores. An increase in fluorescent intensity was measured under the uniaxial deformation, but immediately reversed when the deformation was released (Fig. 8b). However, when excessive force was applied at the strain of 600%, the ring with the luminophore slipped past the stoppers, permanently breaking the mechanical bond. This dethreading resulted in an irreversible fluorescence change. The same group recently reported the fabrication of a cyclophane-based mechanophore containing two fluorescent 1,6-bis(phenylethynyl)pyrene moieties (Fig. 8c).95 The pyrene moieties could form an excimer with an emission around the wavelength of 530 nm, whereas the pyrene monomer emission was at 470 nm, which a induced colour change based on the mechanophore assembly structures. Excimer emission dominated the emission spectra of the dilute solutions with a low composition of cyclophane mechanophore (0.8 wt%) and polyurethane elastomers. Upon deformation, conformational changes occurred in the polymer, leading to a greater monomer emission. This phenomenon was observed when the mechanophore was covalently embedded in a linear, segmented polyurethane elastomer, and the film produced exhibited a distinct colour change from cyan to blue at a strain of 600%.
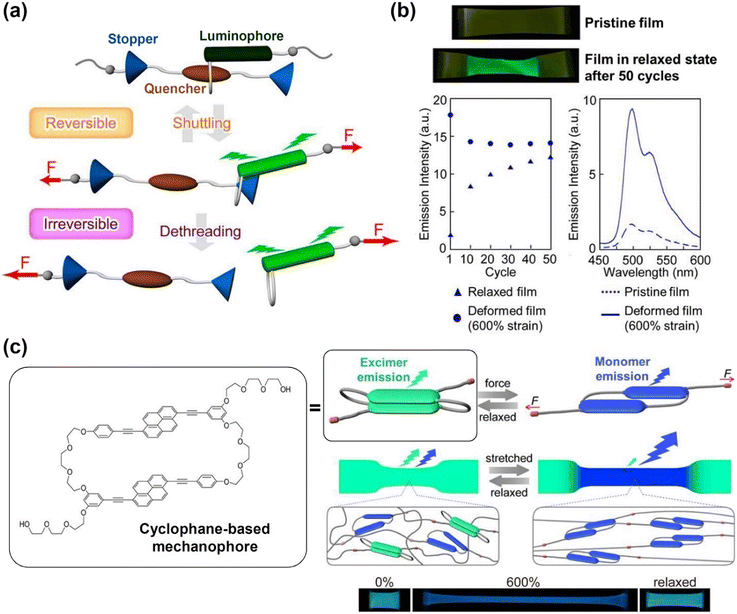 |
| Fig. 8 (a) Schematic diagram of the shuttling and dethreading function of rotaxane-based supramolecular mechanophores. (b) Photographs of the rotaxane-based pristine film and the fluorescent deformed film and plots of the emission intensity against cycle and wavelength for the films under the different conditions as specified. (c) Schematic illustration of the conformational changes in the cyclophane mechanophore leading to mechanochromic luminescence. Reproduced from ref. 94 and 95 with permission. Copyright 2021, the American Chemical Society. | |
Imato and co-workers developed mechanoresponsive supramolecular polymers using fluorescent pyrene and naphthalene diimides incorporated in the mid-chain of poly(ε-caprolactone)s.96 With no mechanical stimuli, fluorescence quenching occurred as the pyrene formed an intramolecular charge transfer complex with the naphthalene diimides. The application of force triggered the dissociation of the complex, leading to strong fluorescent emission.
Tetraarylsuccinonitrile is the another mechanoluminescent moiety that can generate colored radicals using mechanical stimuli. Otsuka's group introduced a tetraarylsuccinonitrile moiety at the crosslinking points of a polymer gel.97 After freezing the polymer gel, the swelled solvent induced homolytic dissociation of the central carbon–carbon bond of the tetraarylsuccinonitrile skeleton, resulting in not only a color change but also light emission of the gel (Fig. 9b). The structure change and mechanoluminescence were reversible upon heating and freezing the gel. The same group also introduced tetraarylsuccinonitrile functions into polystyrene and silsesquioxane composite elastomers, which allowed the materials to display reversible mechanofluorescence upon grinding.98,99
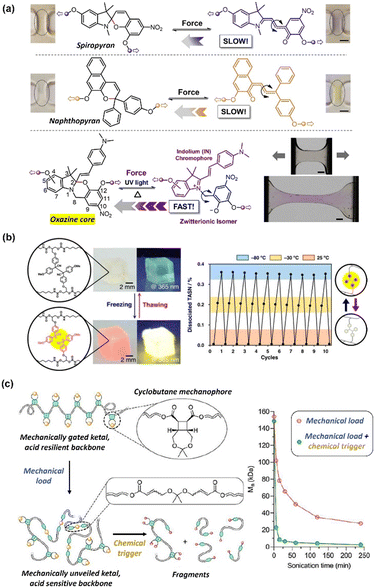 |
| Fig. 9 (a) Force-induced chemical transformations of spiropyran, naphthopyran and oxazine. Inset: Photographs of polymer films before and after the application of force. Reproduced from ref. 101 with permission. Copyright 2021, Elsevier. (b) Reversible color change and light emission of the tetraarylsuccinonitrile-containing gel at ambient temperature (25 °C) and freezing (−80 °C) conditions. (c) Schematic diagram of the mechanical-gated ketal degrading into fragments with the application of both mechanical load and chemical trigger as stimuli and plot of molecular weight (Mn) against sonification time for the mechanically gated polymers in the presence of different stimuli. Reproduced from ref. 104 and 107 with permission. Copyright 2018 and 2020, the American Chemical Society, respectively. | |
Lin et al. incorporated a cyclobutane mechanophore on a polymer backbone as a mechanical gate to regulate an acid-sensitive ketal.100 Given that both the mechanical load and chemical trigger are required to achieve significant molecular weight degradation, this allowed the polymer to retain its degradable properties, while building resistance to unintended degradation (Fig. 9c). The integrity of the polymer backbone was maintained in the presence of only a chemical trigger using trifluoroacetic acid. However, upon the application of a mechanical load through ultrasonication, polymer degradation occurred to an apparent limiting molecular weight of 28 kDa. When the two stimuli were combined, an 11-fold decrease in molecular weight to 2.5 kDa was achieved. Although the bond cleavage was not reversible, the design and introduction of a mechanophore enabled the polymer chains to exhibit unique degradation functions.
2.2.3 Other force-responsive polymers.
Recent developments have led to the emergence of other novel mechanoresponsive structures. Qian et al. employed an oxazine-based mechanophore scaffold to create a fast and reversible force-responsive material.101 Unlike conventional spiropyran and naphthopyran mechanophores, oxazine undergoes a purely ring-opening reaction with no double-bond isomerism involved during its mechanical activation. This process formed coloured zwitterion species, which reverted to the initial state orders of magnitude faster than the merocyanine to spiropyran and naphthopyran transformation (Fig. 9a). The threshold activation strain of the oxazine mechanophore in the bulk polydimethylsiloxane varied in the range of ∼90–125%. No phase lag or response fatigue was observed even after 8 continuous loading–unloading cycles.
2.3 CO2-responsive structure
Carbon dioxide-switchable polymers are stimuli-responsive polymers that employ CO2 as the primary triggering mechanism to alter and reversibly switch the polymer properties. For instance, controlling the degree of hydrophobicity in the presence of CO2 (hydrophilic) or the absence of CO2 (hydrophobic). Incorporating CO2-switchable components has gained much attention in recent years due to their unique advantages including abundance, non-toxic and environmentally friendly nature with no accumulation of chemical species, rapid response, good penetration depth and low cost.102,103 Most importantly, the excellent precision to control and manipulate the polymer chain structure allows great versatility and autonomy. Generally, this strategy revolves around the design and tailoring of the polymer chain structure of CO2-responsive materials in terms of their functionalities, as shown in Fig. 16. The various types of CO2-responsive functionalities are composed of copolymers of various compositions, as well as hybridized with functional nanomaterials to form composites.102,104
Among the polymerisation strategies, atom transfer radical polymerisation (ATRP) is typically preferred due to its low impurities, simplicity, and controlled polymer growth. Generally, this polymerization involves chemical functionalization of the precursor and polymerization of the CO2-responsive monomer to form a CO2-responsive copolymer. CO2-responsive copolymers have huge potential for application in catalysis, nanoreactors, switchable surfactant/stabilizers, drug delivery, and separation.105 Alternatively, hypoxia-responsive polymers are also highly relevant in biomedical applications such as tumor treatment.106 In this section, we discuss the most recent development of CO2-responsive polymers, their mechanism, and their applications.
2.3.1 Guanidine.
Guanidine is an amine derivative that contains three nitrogen groups (two ‘amides’ and one ‘imine’) on one carbon atom, which is also known as a carbon-imidic diamide, as illustrated in Fig. 16. It has an acid dissociation constant (pKa) value of ∼13.5 and is categorized as an organic superbase, given the resonance stability of its conjugated acids. Generally, guanidines undergo a protonation reaction upon CO2 simulation and are converted into guanidinium species. However, the deprotonation reaction can only be achieved by heating instead of inert gas bubbling (Ar or N2) due to their high pKa value.107 For instance, Lowe and co-workers developed a polyarginine homopolymer and block copolymer via a two-step modification including RAFT polymerization and post-polymerization modification. Interestingly, the copolymer reversibly formed micelles under N2 and dissociated into a linear chain under CO2 exposure in an aqueous medium.108 In another work, Theato and Schattling synthesized a random copolymer of various L-arginine and acrylamide using a similar two-step modification and discovered its dual temperature and CO2-responsive behaviors. The copolymer exhibited a reversible hydrophilic/hydrophobic performance under CO2 or Ar exposure, which can be utilized in drug delivery systems, sensors, and even as “smart” CO2 trapping surfaces.109
2.3.2 Amidine.
Amidine contains two nitrogen atoms in the ‘amide’ and ‘imine’ functional groups in its structure, which resembles carboxylic acids and has an average pKa value of ∼9.0 (in the range of 5–12). As shown in Fig. 10, the protonation reaction converts the imino nitrogen to a symmetrical amidinium ion, which can be stabilized by resonance. In general, the stronger basicity of amidine groups causes the equilibrium constant between CO2 and amidine to be substantial (KtE = 102–105), leading to higher CO2 sensitivity and responsivity. Jessop and co-workers first reported the preparation of CO2-responsive aliphatic amidine-containing surfactants in 2005.110 Subsequent work by Yan et al. synthesized an amidine-based copolymer via ATRP of the difunctional monomer (N-amidino)dodecyl acrylamide (AD). These tuneable drug delivery vesicles exhibited smart expansion and contraction cycles upon CO2 stimulation with controlled-release capability.111 Guo and co-workers adopted a post-polymerization modification strategy, converting poly(4-chloromethyl styrene) (PCMS) into poly(pazidomethyl styrene) (PAMS) with high amidine functionalities via a Cu(I)-catalyzed cycloaddition “click” reaction. The resultant homopolymers displayed a reversible hydrophobic–hydrophilic evolution in CHCl3/water when exposed to CO2 and N2 in an aqueous medium, showing potential application in sensors, “smart” surfaces, and drug delivery.111 However, amidine is highly susceptible to hydrolysis in the absence of a CO2 environment, which disrupts its self-assembly. Moreover, the complex synthesis of amidines makes them a less preferred choice.
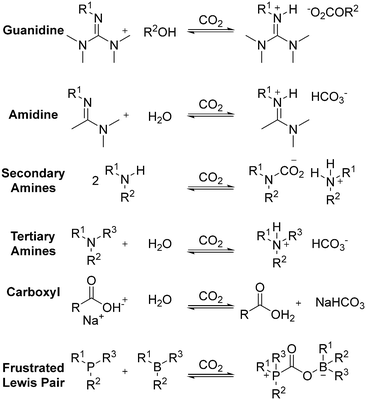 |
| Fig. 10 Functional groups and chemical changes involved in CO2-responsive polymers. | |
2.3.3 Amine-based copolymers.
All amine-based copolymers can react with CO2 to form the corresponding bicarbonate salts in water, including the bulky primary and secondary amines, and tertiary amines, as shown in Fig. 10. Among them, tertiary amines possess the best CO2 reversibility and switchability, which is attributed to their weak alkalinity (pKa = 6.7–8.0).112 The protonation reaction by the bicarbonate anion (pH = 4.0–5.0) can easily occur at ambient temperature, whereas heat is required for primary and secondary amines. Polymers containing tertiary amines can be fully separated in water/THF co-solvent due to their exceptional ionic conversion capability in water. Moreover, the simple manufacturing process and commercial availability of tertiary amines make them the prime choice.113 Functional monomers with tertiary amines include 2-(dimethylamino)ethyl methacrylate (DMAEMA), 2-(diethylamino)ethyl methacrylate (DEAEMA), and melamine derivative [N2,N4,N6-tris(3-(dimethylamino)propyl)-1,3,5-triazine-2,4,6-triamine] (MET) and others. These monomers offer great possibility to design CO2-responsive chain structures by varying their composition/sequence (homopolymers, block, random, alternating, gradient and statistical copolymers), and topologies (graft polymer, hyper-branched, and polymer network). They demonstrated great utilization as catalysts,114 nanoreactors,115 switchable surfactants/stabilizers,104,116–121 and membrane separators.113,122,123
2.3.3.1 Poly(N,N-dimethylaminoethyl methacrylate) (PDMAEMA).
To date, poly(N,N-dimethylaminoethyl methacrylate) PDMAEMA and poly(N,N-diethylaminoethyl methacrylate) PDEAEMA have been labeled as the perfect CO2-switchable moieties and are significantly utilized in many applications. The comparable pKa value of the amine copolymers to the aqueous medium allows rapid and reversible protonation/deprotonation under alternating CO2/inert gas stimulations.113 Despite their small structural difference, Zhao and Yin found that the graphene oxide (GO)/pyrene end-functionalized PDMAEMA (Py-PDMAEMA) nanofiltration membrane performed better than the GO/Py-PDEAEMA nanofiltration membrane. They assembled Py-PDMAEMA on GO sheets through π–π stacking and electrostatic interaction and fabricated the membranes via vacuum filtration on a PVDF microfiltration membrane. The hydrophilic PDMAEMA displayed good solubility and extended chain conformation in an aqueous medium regardless of the alternate CO2/Ar bubbling, which maintained an excellent size exclusion effect and charge property, as shown in Fig. 11. On the contrary, the hydrophobic PDEAEMA collapsed after eliminating CO2 and covered the GO surface, which resulted in a reduction in the charge property and size exclusion effect of the membranes.123 Zhao and Fan synthesized a random copolymer of poly((N,N-dimethyl aminoethylmethacrylate)-co-4-methyl-[7-(methacryloyl)oxy-ethyl-oxy]coumarin) (P(DMAEMA-co-CMA)) and an amphiphilic diblock copolymer of PS-b-P(DMAEMA-co-CMA) (PS is hydrophobic polystyrene). The CO2-responsive single-chain polymeric nanoparticles aggregated and self-assembled into core–shell micelles in water. The reversible protonation/deprotonation reaction of the tertiary amine groups made the SCNPs swell and shrink reversibly under CO2/N2 stimulation, showing great potential as effective gas-tuneable nanoreactors.115 Recently, block and random copolymers of DMAEMA and poly(methyl methacrylate) (PMMA) were synthesized through RAFT solution polymerization. The team obtained latexes that could coagulate and re-disperse under alternating N2 and CO2 bubbling. They found that the optimum protonation could be achieved by randomizing the MMA content over blocks of MMA. The rapid protonation was contributed by the larger gap between the nitrogen atoms, making them a promising CO2-switchable stabilizer.116
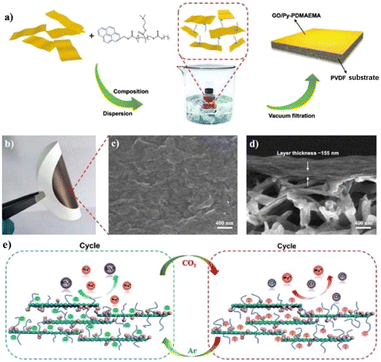 |
| Fig. 11 (a) Schematic illustration of the fabrication process of CO2-responsive GO/Py-PDMAEMA nanofiltration membrane. (b) Photo of the resulting membrane. (c) Top and (d) cross-section scanning electron microscopy (SEM) images of the membrane. (e) Mechanism of CO2-responsive GO/Py-PDMAEMA composite nanofiltration membrane with gas-induced charge sign reversal on the rejection of salts. Reproduced from ref. 123 with permission. Copyright 2020, Elsevier. | |
2.3.3.2 Poly(N,N-diethylaminoethyl methacrylate) (PDEAEMA).
In addition to the typical applications of amine-based copolymers, gas-enabled self-cleaning membranes have gained immense popularity. For instance, Zhang and co-workers122 developed a blend comprised of the CO2-responsive copolymer polyacrylonitrile-co-poly(N,N-diethylaminoethyl methacrylate) (PAN-co-PDEAEMA) with PAN matrix via a phase inversion process. The controllable hydrophilicity/hydrophobicity of the membrane surface dictated its self-cleaning efficiency. Upon CO2 stimulation, the collapsed hydrophobic PDEAEMA segments instantly transformed into a chain-extended state due to the protonation of the tertiary amine groups. The reaction induced hydrophilicity, which deterred the adsorption of proteins on the membrane surface. Deprotonation of the tertiary amine groups by N2 bubbling returned them to the hydrophobic state. Remarkably, the cleaning efficiency of this green strategy is comparable to that of the traditional acid and alkaline cleaning.122
In their recent work, Feng and Jiang118 studied the effects of the chemical structure and segment composition on the topological effect and tendency of the CO2 response by evaluating the properties of self-assembled micelles, as shown in Fig. 12. They synthesized three types of amphiphilic copolymers based on poly(ethylene oxide) (PEO, hydrophilic component) and DEAEMA (hydrophobic component) in the form of di-block, tri-block, and a novel cyclic structure via ATRP polymerization. As illustrated in Fig. 12b, the cyclic copolymer (copolymer 1) possessed the largest particle given that it promoted micelle formation and increased the aggregation of the micelles. A plot of particle size for the different copolymers are also depicted in Fig. 12c corresponding to their treatment times shoring that copolymer 1 exhibits the largest particle size.
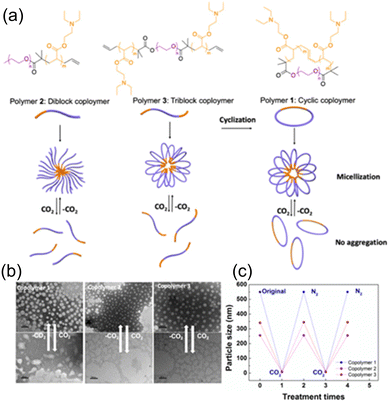 |
| Fig. 12 (a) Chemical structures of polymer 1 as a cyclic amphiphilic block copolymer, 2 as a di-block copolymer, and 3 as a tri-block copolymer. Schematic representation of the formation of micelles controlled by CO2. (b) Transmission electron microscopy (TEM) images (left) and change of hydrodynamic size (right) of the copolymers 1, 2, and 3 (0.2 wt%) in the presence or absence of CO2. The scale bars of TEM images in the absence CO2 (upper) are 1000 nm and that in the presence of CO2 (lower) are 100 nm. Reproduced from ref. 118 with permission. Copyright 2020, Elsevier. | |
The same group developed a new strategy to generate lightly crosslinked poly(diethylaminoethyl methacrylate-styrene) [P(DEA-St)] latexes with different PDEA contents via one-pot emulsion copolymerization, as displayed in Fig. 13.117 At a high DEA content (98.05 wt%), the latexes switched steadily between swelling and deswelling, which was attributed to the CO2-reversible protonation/deprotonation reaction of their PDEA portions. On the contrary, a low DEA content (below 13.74 wt%) made the latexes CO2-insensitive due to the insignificant electrostatic interactions. This crosslinked structure proved to be a promising strategy for other common latexes such as PMMA and PS.120,121
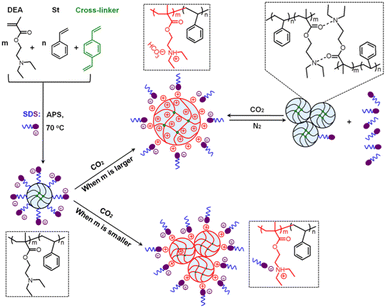 |
| Fig. 13 Schematic illustration of the fabrication of P(DEA-St) latexes with SDS used as an emulsifier, as well as the mechanism for the responsive destabilization, reversible redispersion, and switchable expansion/collapse transition of the latexes induced by CO2/N2. Reproduced from ref. 117 with permission. Copyright 2020, Elsevier. | |
2.3.3.3 Melamine derivative [N2,N4,N6-tris(3-(dimethylamino)propyl)-1,3,5-triazine-2,4,6-triamine].
Yin and Feng124 reported the preparation of a CO2-stimulated reversible graphene dispersion by non-covalently functionalizing reduced graphene oxide with a CO2-sensitive melamine derivative, N2,N4,N6-tris(3-(dimethylamino)propyl)-1,3,5-triazine-2,4,6-triamine (MET). They found that the excellent graphene dispersion is attributed to the strong van der Waals interaction between MET and the graphene surface. Reversible aggregation/dispersion could be easily realized by interchanging CO2 and N2 bubbling, which manipulated the desorption/adsorption of MET on the graphene surface. This novel gas-triggered aggregation/dispersion smart graphene with controllable stimuli-responsive dispersity demonstrates great potential in the medical and biochemical fields. Recently, the same group developed a biocompatible polylactic acid (PLA) bioinspired honeycomb-like porous film with ON/OFF CO2 gas-triggered switchable wettability.125–127 This group employed the breath figure method to prepare the non-responsive PLA and CO2-sensitive MET. The film displayed noticeable hydrophobic–hydrophilic conversion, as shown in Fig. 14. By tuning the PLA/MET ratio, they could precisely position the hydrophilic CO2-sensitive groups in the inner surface and control the water penetration and wettability of the pore. Consequently, the enhanced surface wettability improved the cell–film interaction and the subsequent cell attachment. The combination of a biocompatible polymer and natural gas trigger allows the preparation of future intelligent materials for biomedical and bioengineering applications.
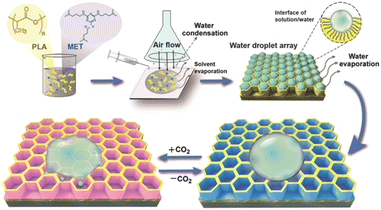 |
| Fig. 14 Schematic diagram of the honeycomb-like porous film prepared from the PLA/MET complex and its CO2-triggered reversible wettability. Reproduced from ref. 125 with permission. Copyright 2021, the American Chemical Society. | |
2.3.4 Carboxyl-based copolymers.
Generally, carboxyl has a relatively low pKa value of ∼5.0 to 9.0. It undergoes a protonation reaction upon CO2 simulation and converts the sodium salt of acid from anionic surfactants to non-ionized erucic acid, as shown in Fig. 10. For instance, Zhang and Feng developed a CO2-responsive anionic worm-like micellar system based on sodium erucate.128 The protonation/deprotonation process interchanged between the worm-like micelles and low-viscosity emulsion particles, which is attributed to the reversible conversion of the sodium salt of the erucic acid (anionic surfactant) into the non-ionized erucic acid. In another work, Fischer and co-workers reported the preparation of carboxyl functionalized, CO2-switchable nanoparticles via surfactant-free mini-emulsion polymerization by employing a reactive surfactant known as surfmer, i.e., N-methacryloyl-11-aminoundecanoic acid (treated equimolarly with NaOH).129 The obtained latex had a size of about 100 nm with good dispersion. The protonation reaction was carried out via CO2 bubbling to instantly form carbonic acid, which covalently attached onto the surface of the colloid, and could be deprotonated by ultrasonic treatment. In another work by Wang and Zhu, they fabricated zwitterionic crosslinked polymer particles comprising DEAEMA and sodium methacrylate (SMA).130 These particles possessed CO2/N2-adjustable isoelectric points in the pH range of 7.5–8.0, and subsequently were used as an emulsifier for Pickering emulsions. The optimization of the cationic amine group and the anionic carboxyl group provides insight for the preparation of future CO2-responsive polymer materials.
2.3.5 Lewis acid–base pair copolymers.
The most common approach and strategy for the preparation of CO2-responsive polymers is by modifying the polymer chain functionalities, as shown in the earlier sections. There has been an increase in the development of innovative dual CO2-responsive polymer systems based on the frustrated Lewis pair (FLP) theory in recent years. Stephan and co-workers first introduced this theory in 2006,132 where the Lewis acid and base offer latent interactions to connect small molecules such as dihydrogen (H2) and carbon dioxide (CO2) to create a connecting (bridging) structure that possesses reversible cleavage and re-bonding feature, as illustrated in Fig. 15. For instance, Yan and co-workers demonstrated this theory by adding Lewis acid–base pairs to a polymer, and successfully developed a recyclable nano-catalyst with good activity and recyclability, showing great potential as a wastewater treatment membrane.133
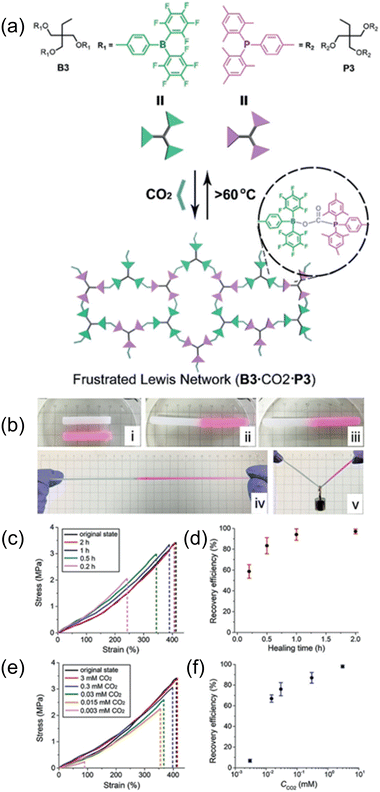 |
| Fig. 15 (a) Trefoil-like frustrated Lewis pair (B3 and P3) as trivalent building blocks to form CO2-crosslinked frustrated Lewis networks with reversible CO2/thermal responsiveness via CO2-bridging dynamic covalent linkages. (b) Self-healing process of the frustrated Lewis network: i) two cut samples (one loaded with red pigment), ii) cut surfaces were treated with CO2 and brought into contact, iii) self-healed for 30 min under ambient conditions, iv) the healed gel under stretching, and v) on loading. (c) Stress–strain curves of original and healed gel samples after various healing times. (d) Plot of the gel recovery efficiency versus healing time. (e) Stress–strain curves of original and healed gel samples under various levels of CO2 stimulus. (f) Plot of the gel recovery efficiency versus external CO2 concentration. Error bars in (d) and (f) denote the standard deviations from at least three experiments. Reproduced from ref. 131 with permission. Copyright 2019, Wiley. | |
Subsequently, they adopted the same theory and developed a novel polymer system exhibiting ultrafast response, self-healing capability, reversibility, and excellent mechanical robustness. As shown in Fig. 15, this is due to the bridging of CO2 in the polymer segment, which subsequently induced assembly in organic solvent systems, demonstrating potential in future gas-sensitive smart materials.131 In their most recent work, they developed a gel material with a double-crosslinked FLP network comprised of dynamic CO2 gas-bridged connections and permanent chemical crosslinks by reacting triaryl borane (TB), triarylphosphine (TPP), and CO2. Notably, the strength and toughness of the gel could be tuned by adjusting the CO2 content, which dictated the density of supramolecular nodes in the network. Furthermore, the excellent self-healing stimulated by CO2via dynamic covalent network reconstruction allows these innovative soft materials to be potentially applied as sensors, actuators, and biomedical devices.134
2.4 Redox-induced structure switch
The development of redox-responsive polymeric materials has been dramatically researched over the years to optimize and regulate the delivery and release of encapsulated therapeutic agents, such as doxorubicin (DOX), camptothecin (CPT), and paclitaxel (PTX), especially for tumour treatment. Their environment displays many redox activities involving biological reductants and oxidants, making redox-responsive materials a suitable material for the preparation of drug delivery systems.135 The typical redox-responsive species include (i) reactive oxygen species (ROS), (ii) glutathione species (GSH), (iii) ROS + GSH species, and the recent (iv) hydrogen sulphide (H2S)-based species, as displayed in Fig. 16.136 Generally, there are two approaches to incorporate drugs in delivery systems. The first approach involves loading a drug in an amphiphilic micelle assembly, where hydrophobic–hydrophilic conversion is the primary responsive mechanism. The second approach involves incorporating the drug in a polymer chain integrated with a responsive species, where bond cleavage is the main responsive mechanism.137
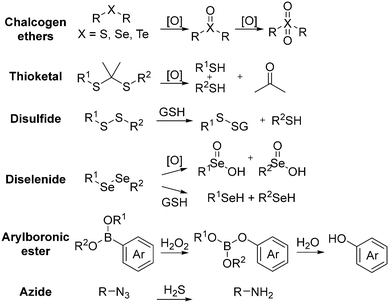 |
| Fig. 16 Functional groups and chemical changes involved in redox-responsive polymers. | |
ROS is a highly reactive species made up of a group of oxygen-containing molecules. It is categorized into two types, i.e., the radical type comprised of hydroxyl radical (HO˙) and superoxide (O2−) and the non-radical type comprised of hydrogen peroxide (H2O2), HOCl, and singlet oxygen (1O2). Among them, H2O2 is a popular ROS-responsive material due to its relative stability and longer half-life. A low concentration of these species makes them a useful biological signal in living organisms, while excessive amounts can lead to an imbalance between ROS and antioxidants, which is known as oxidative stress. This oxidative stress damages lipids, proteins, and DNA, adversely leading to physical harm.138 Examples of ROS-based species include chalcogen ethers, thioketals, aryl boronic esters, and many others.
Alternatively, GSH is a reduction-responsive tripeptide containing cysteine, glycine, and glutamate. The huge redox potential difference between the intracellular (∼10 mM) and extracellular environments (∼10 μM) at any concentration make GSH a proper biological signal for intracellular delivery. Most importantly, GSH production maintains the redox equilibrium by balancing the oxidative stress produced by ROS.139 Ideally, the employment of both ROS and GSH will be favourable because of the versatility and performance. Besides the typical species, H2S, the simplest gaseous thiol, has gained attention lately. H2S exists in deprotonated anion form due to its acidic nature and has demonstrated positive physiological and pathological effects, especially in encapsulated therapeutics.140–142
2.4.1 Disulfide linkage.
A widely used GSH-responsive species, disulfide, is comprised of a sulfur–sulfur (S–S) linkage with a bond energy of 240 kJ mol−1. In the presence of GSH, the disulfide linkage undergoes oxidative cleavage to form thiols, as shown in Fig. 16, contributing to successive drug release. The similarity between the thiol–disulfide exchange reaction to the biological system makes it an ideal drug delivery capsule.139 Generally, the disulfide linkage is incorporated via various strategies. The first strategy is integrating the disulfide linkage into the main chain, as demonstrated in the recent studies by Ghosh and co-workers.143 Their group incorporated a disulfide group into the supramolecular assembly of a protein, bovine serum albumin (BSA), which underwent disulfide linkage cleavage upon GSH exposure. Subsequently, the protein was released, leading to enzymatic activity. In another work, Ju and He designed and fabricated a reduction-sensitive amphiphilic triblock copolymer poly(ethyl ethylene phosphate)-b-poly(disulfide)-b-poly(ethyl ethylene phosphate) (PEEP-PDS-PEEP) through thiol–disulfide polycondensation and ring-opening polymerization (ROP).144 The copolymer demonstrated good self-assembly and profoundly released the DOX hydrophobic drug upon reduction-trigger via poly(disulfide) scission and dissociation. Recently, Wang et al. introduced a disulfide bond into the conjugates of oxaliplatin(IV) and oleic acid (OA) to form a reduction-responsive nanoscale assembly.145 Similar to previous work, the self-assembly structure underwent disulfide bond cleavage and displayed high anticancer potency and drug efficiency.
The second strategy is by pendant group integration, where a disulfide-containing component is attached to the main backbone of the polymer. For instance, Shen et al. attached the disulfide-containing cleavable prodrug monomer camptothecin (CPT) to a methoxy poly(ethylene glycol) (mPEG)-based polymer chain by RAFT living polymerization and developed an amphiphilic copolymer.147 Besides CPT, doxorubicin (DOX) was encapsulated via hydrophobic and π–π stacking interactions. In their work, the co-release of both anticancer drugs was well controlled and demonstrated a synergetic effect with enhanced anticancer efficacy, as illustrated in Fig. 18.
The last strategy is by crosslinking with a cleavable disulfide-containing crosslinker. Bhattacharya and co-workers covalently crosslinked the disulfide group on a polymer chain and reported an excellent drug release profile.148 In 2020, Zhao et al. directly polymerized methacrylic acid with the reductive-sensitive crosslinker bis(2-methacryloyloxyethyl) disulfide (DSDMA) or N,N′-bis(acryloyl) cystamine (BAC) using S-propranolol as a model template to form a degradable molecularly imprinted polymer (MIP).146 As shown in Fig. 17, the team found that the MIP-disulfide could readily degrade via bond cleavage in a reductive environment and had a better release performance than the traditional ethylene glycol dimethacrylate (EDMA)-based MIP. This approach can be effectively adopted as an intracellular controlled drug delivery system.
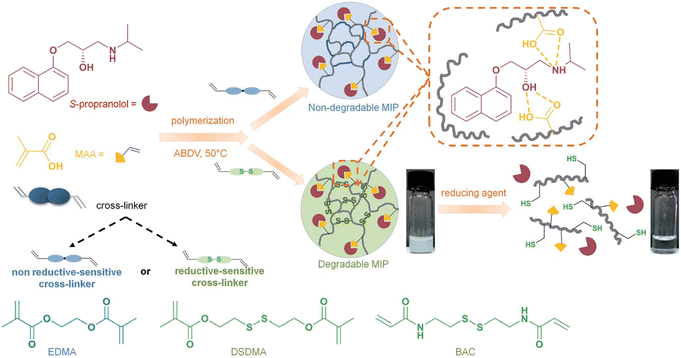 |
| Fig. 17 Schematic representation of synthesis and reduction-induced degradation of S-propranolol molecularly imprinted polymers for drug delivery. Adapted with permission from ref. 146 Copyright 2020, The Royal Society of Chemistry. | |
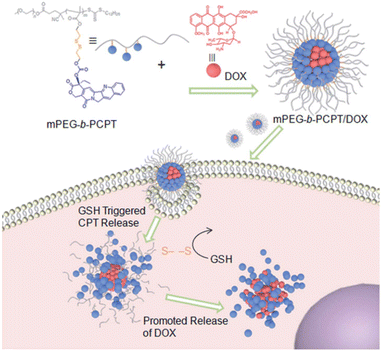 |
| Fig. 18 Schematic illustration of the preparation of mPEG-b-PCPT/DOX nanomedicine and the “drug release promoting release strategy” of mPEG-b-PCPT/DOX for synergistic cancer chemotherapy. Adapted with permission from ref. 147 Copyright 2019, The Royal Society of Chemistry. | |
2.4.2 Chalcogen linkage.
Chalcogens are chemical elements found in group XIV of the periodic table, which are comprised of sulfur (S), selenium (Se), and tellurium (Te). These three common chalcogens possess similar chemical reactivities and readily undergo oxidation, as illustrated in Fig. 16. However, the difference in their electronegativity and atomic radius influences their oxidation sensitivity and reactivity. The one with the lowest electronegativity, tellurium, has the highest sensitivity, followed by selenium (Se), and lastly sulfur (S). These elements are typically integrated into the main chain and/or within the pendant groups to form various ether-based copolymers such as thioether (S-based), selenoether (Se-based), and telluroether (Te-based), which are activated based on the hydrophilicity of the ether group.
Similarly, telluroether has the highest sensitivity, followed by selenoether, and lastly thioether. For instance, Lee et al. developed three ROS-responsive amphiphilic block copolymers based on polycaprolactone (PCL) with selenide and sulfide pendent.149 As expected, PCL-co-selenide demonstrated faster oxidation compared to PCL-co-sulfide due to the high sensitivity of selenide. However, it transformed back to its amphiphilic nature after the β-elimination reaction, leading to a poor disassociation and drug release performance. Their result showed that α-ethylthio caprolactone displayed an excellent H2O2-induced hydrophobic–hydrophilic transition from ethyl thioether to its sulfoxide. This event resulted in excellent disassembly, demonstrating a promising vesicle for photodynamic therapy.150
Xu and group intensively researched selenoether-based copolymers. In their recent high-impact work, they successfully developed selenium-containing nanoparticles combining various cancer treatments (radiotherapy, immunotherapy, and chemotherapy), as illustrated in Fig. 19. Seleninic acid was formed upon the cleavage of the diselenide bonds under γ-radiation, which exhibited anticancer activity by upregulating the reaROS levels in cancer cells, paving a promising path for simultaneous cancer treatment.113,151 The tumor-targeting peptide-modified nanoparticles could actively accumulate in cancer cells through systemic administration. Following radiotherapy, the diselenide portion in the polymer was oxidized to seleninic acid, and the loaded drug DOX was released in the nucleus, realizing its chemotherapy function. The seleninic acid blocked HLA-E expression, and further enhanced the NK cell-mediated immunotherapy by releasing cytokines and lytic molecule (granzyme). Recently, Xu and team employed the hydrothermal process to fabricate carbon-dots with selenium–sulfur dynamic covalent bonds. The Se–S-CDs increased the thioredoxin reductase (TrxR) activity and enhanced the cell viability, demonstrating promising biological applications such as cell culturing.27,152,153
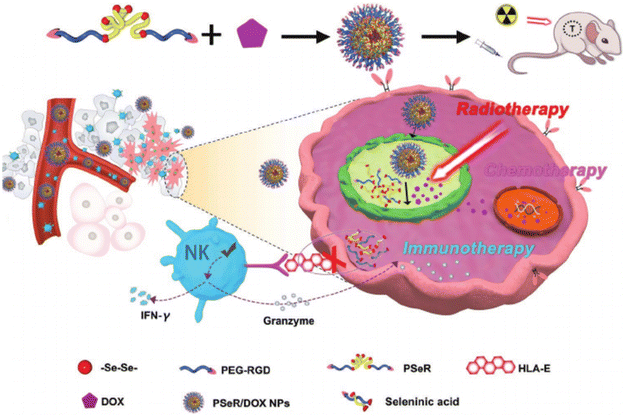 |
| Fig. 19 Schematic illustration of utilizing selenium-containing nanoparticles to implement combined chemotherapy, radiotherapy, and immunotherapy. Adapted with permission from ref. 155 Copyright 2020, Wiley. | |
2.4.3 Thioketal linkage.
Thioketal is a sulfur-based ROS-responsive group that undergoes an oxidative backbone cleavage mechanism to produce two thiols and a ketone product. Hence, polymers containing this linkage will degrade and dissociate in the presence of ROS, as shown in Fig. 16, and are widely used in drug delivery systems.154–157 Wang et al. employed the thioketal linkage to develop a pathologically responsive mitochondrial gene delivery vector. Their recent work demonstrated the thioketal-based polymer dissociation by high mitochondrial ROS exposure to release functional DNA genes that improve the gene transfection efficiency and correct genetic abnormalities, showing great discovery in in situ mitochondrial gene therapy.154 In a recent study, Li and Chen developed a thioketal-gated mesoporous silica nanoparticle by grafting ROS-sensitive mPEG on the particle surface. The vancomycin-loaded MSP displayed an excellent controlled release profile through thioketal cleavage. Besides, the antibacterial properties and non-cytotoxicity show its capability as an antibacterial material for wound treatment.157
2.4.4 Aryl boronic ester.
Aryl boronic ester is an ROS-responsive functional group, which is widely utilized in the preparation of H2O2-responsive materials due to its selectivity and sensitivity towards H2O2. The linkage is usually attached as a pendant group and reacts with H2O2 by the backbone breakage or hydrophilicity switch. For instance, Hsu and co-workers synthesized a PCL bearing pendant aryl boronic esters. Exposure to H2O2 induced the migration of the aryl group to the oxygen atom, known as nucleophilic addition, followed by the hydrolysis of the borate ester to release phenol, which underwent 1,6-rearrangement. Subsequently, this rearrangement exposed the active phenol group. The copolymer showed high sensitivity to H2O2 and good hydrolytic stability, allowing it to be used as a drug delivery carrier. Garcia et al. synthesized a diblock copolymer based on a hydrophilic PEG segment and an aryl boronic ester-functionalized hydrophobic polycarbonate segment. They systematically studied the oxidation of nanoparticles and found that the dissociation of aryl boronic ester was highly dependent on the polymer concentration, while the amount of H2O2 could control the drug release amount.158 In recent work, Jager prepared a novel ROS-responsive amphiphilic block copolymer comprised of a hydrophobic boronic ester-based ROS in the hydrophilic backbone. The copolymer yielded either the hydrophilic carboxylic acid or an amphiphilic phenol upon exposure to H2O2 and displayed a hydrophilic switch before the controlled drug release.159
2.4.5 Diselenide linkage.
Both oxidation responsiveness and reduction responsiveness can be found in diselenide-based copolymers due to the similar chemical properties of selenium and sulfur. The dual-responsive capability allows diverse applications in both physiological and pathological environments. In addition, the bond energy of diselenide (Se–Se 172 kJ mol−1) is much lower than that of the carbon-selenium bond, C–Se (244 kJ mol−1), and disulfide bonds (S–S, 240 kJ mol−1), making it more reactive to an external stimulus. These findings were evinced in the work by Luo and Zhang, where (mPEG–PCL–Se)2 tri-block copolymer micelles showed faster DOX release than the (mPEG–PCL–S)2 tri-block copolymer micelles.161 In another work, Tsai and co-workers developed a diselenide-linked polymeric nanogel (PEG–Se–Se). They performed the redox-responsive drug release study using 0.1% H2O2 and 0.1 mg mL−1 of GSH in PBS buffer with pH of 7.4 at 37 °C. The drug-loaded diselenide nanogel underwent bond cleavage under stimuli (H2O2 and GSH), resulting in the release of the encapsulated DOX. The nanogel exhibited good DOX release profiles in the presence of both GSH (reductive) and H2O2 (oxidative).162
Recently, Tian and co-workers designed 2-methacryloyloxyethyl phosphorylcholine (MPC) with a diselenide bond-containing crosslinker (N,N′-bis(methacryloyl) selenocystamine (BMASC)). Copolymerization resulted in the formation of a novel zwitterionic nanogel with an exceptional dual redox-labile property. As illustrated in Fig. 20, the diselenide linkage of the drug-loaded nanogel cleaved and dissociated in the reducing (GSH) and oxidative (H2O2) environments. Subsequently, the disintegrated nanogel released the drug effectively. In addition to its excellent release profile, the low leakage and non-cytotoxicity of the nanogel make it a suitable drug delivery carrier.160
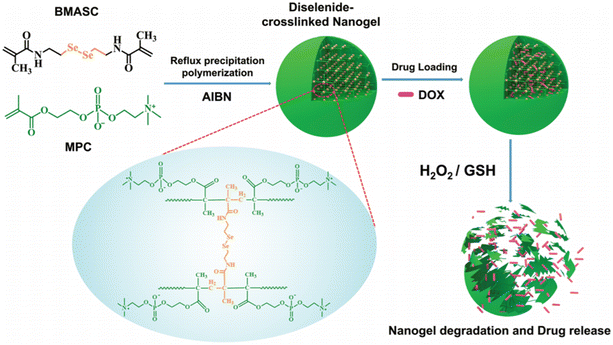 |
| Fig. 20 Illustration of the preparation, biodegradable behavior and dual redox-responsive drug release of P(MPC-Se-Se-MPC) nanogels. Adapted with permission from ref. 160 Copyright 2020, Wiley. | |
2.4.6 Azide-based linkage.
Currently, the bio-orthogonal azido group has been identified as a potential H2S-responsive copolymer due to its high H2S-responses, where azide reduction is the primary mechanism. For instance, Zhang and Lin prepared a series of H2S-trigger N-(2-hydroxyethyl)-4-azide-1,8-naphthalimide-ended amphiphilic diblock copolymers, poly(2-hydroxyethyl methacrylate)-block-poly(methyl methacrylate) (N3-Nap-PHEMA-b-PMMA-N3). Upon the introduction of H2S, the DOX-loaded micelles underwent charge reversal from negative to positive as the azido functional group reduced. The cellular uptake of DOX-loaded micelles was improved by employing an electrostatic attraction, contributing to the fast DOX release rate for potential tumor diagnosis and therapy.140
In another work, Yan and Sang created a novel O-azido methyl benzoate (AzMB)-containing block copolymer that is highly responsive to H2S stimulus. As shown in Fig. 21, the cleavage of the AzMB functional group changed the amphiphilicity, leading to controlled dissociation of the self-assembled nanocapsule.141 Similarly, Almutairi and Kawasaki developed a self-assembled H2S-responsive cholesterol-modified dextran (SC-Dex) nanogel in their recent work. In the presence of H2S, cholesterol dissociated from the main dextran chain via reduction and arrangement of the aryl azide functional group. Subsequently, the nanogel swelled and released the encapsulated protein controllably.142
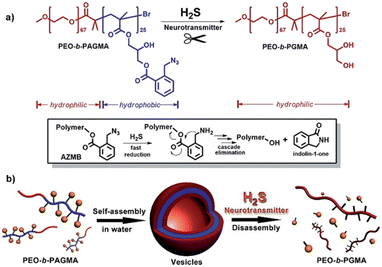 |
| Fig. 21 (a) H2S-responsive cleavage of O-azido methyl benzoate (AzMB)-containing diblock copolymer (PEO-b-PAGMA) and H2S-induced cascade reaction mechanism. (b) Schematic illustration of polymer self-assembly into vesicles and H2S-responsive controlled disassembly process. Copyright (2016) adapted with permission from ref. 141 Copyright, 2020 Wiley. | |
2.4.7 Other redox-responsive polymers.
Besides the several linkages mentioned above, other redox-sensitive bonds such as ferrocene-based and polyoxalate-based bonds were incorporated to create redox-responsive polymer delivery systems. Ferrocene is an organometallic chemical compound that is comprised of two coplanar cyclopentadienyl rings bound on opposite sides of a central iron (Fe) atom. These metallocenes are usually ROS responsive, stable, and have an excellent reversible redox property. Hydrophobic–hydrophilic conversion is the primary responsive mechanism, where the hydrophobic ferrocene groups are oxidized into hydrophilic ferricenium upon exposure to ROS. For instance, Xu and co-workers designed a ferrocene-containing amphiphilic block polymer (PACMO-b-PAEFC) via ATRP, with poly(2-acryloyl oxyethyl ferrocenecarboxylate) (PAEFC) as the hydrophobic component and poly(N-acryloyl morpholine) (PACMO) as the hydrophilic component. Under ROS triggering, the reductive ferrocene groups of the PACMO-b-PAEFC micelles were converted into the hydrophilic ferrocenium, resulting in swelling and releasing the encapsulated drugs. In addition, the oxidative micelles could reversibly convert back upon reduction by vitamin C, as shown in Fig. 22.163 In another work, Höcherl and Jäger164 synthesized a novel self-immolating H2O2-sensitive biodegradable polyoxalate prodrug via a simple one-pot synthesis. The drug molecules were integrated into the backbone of the polymer with responsive polyoxalate bonds. The linkage underwent rapid chain-cleavage in an oxidative environment and effectively released the drugs as required.
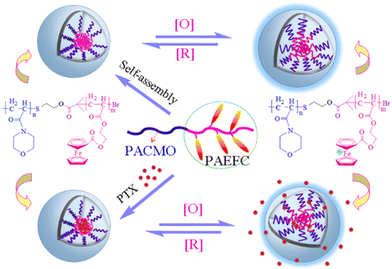 |
| Fig. 22 Schematic diagram of the loading and in vitro release of drug from the copolymer micelles upon triggering by oxidation and reduction stimuli. Adapted with permission from ref. 163 Copyright 2017, the American Chemical Society. | |
2.5 pH-responsive polymers
pH-responsive polymers have great potential to be applied in fields such as drug delivery, although at present, significant limitations in polymer encapsulation-based delivery systems have been observed in clinical trials.90,165,166 By carefully strategizing and tailoring the side chains of the polymer, the pH-responsive behaviours of these polymers can be manipulated via the bottom-up approach, achieving the appropriate response from the polymer at the chemical environment of the active site.9,90,167–171 In this section, the pH-induced structural transformation of several responsive polymers including acid-labile polymers, crosslinking polymers, and zwitterionic polymers will be discussed. Given that there are a myriad of examples of pH-responsive polymers, we aim to highlight a few works and applications. The interested reader is referred to other comprehensive reviews in the literature regarding these polymers.172,173
2.5.1 Boronic acid linkage.
Boronic acid-functionalised polymers pose a great advantage of structural reversibility in detection and sensor applications due to their reversible dynamic covalent bonds formed upon complexation with diol/catechol moieties.174 The pH sensitivity of the complexation and dissociation from crosslinking of the polymer chains allows adverse changes in the physical properties of the polymer, resulting in a response to a change in the chemical environment.175 In the following section, several examples of boronic acid polymers are highlighted to emphasize their efficiency as pH-responsive polymers for several loading and release responses on various substrates.
Ju et al. prepared a pH-responsive cellulose/4-vinyl-phenylboronic acid (VPBA) composite bio-hydrogel using electron-beam irradiation at room temperature for the polymerisation of VPBA to poly VPBA.176 The novel composite bio-hydrogel was believed to have potential application in the biomedical field due to the incorporation of the biocompatible cellulose matrix.177 They made use of the concept of reversibility of the complex formed between glucose monomers in cellulose and the phenylboronic acid in VPBA.178 They also highlighted that the equilibrium established in aqueous medium between the neutral and anionic form of the borate was a crucial factor for the formation of covalent complexes, as shown in Fig. 23a. The complex formed between anionic boronic acid and glucose was stable, whereas the complex of neutral phenylboronic acid and glucose was unstable. The resulting ionisation equilibrium shifted towards the anionic phenylboronic acid. This demonstrates that two factors, i.e., pH and glucose concentration, affect the equilibrium position between the charged and uncharged phenylboronic acid. Thus, the polymer characteristics such as hydrophilicity can be drastically affected upon regulation of the glucose level. Lastly, they demonstrated the responsive properties of the novel composite bio-hydrogel through the self-regulated release of insulin from the composite hydrogel through various concentrations of glucose solution. The release of the insulin was labelled via fluorescein isothiocyanate (FITC) for the tracking of fluorescence intensity of the external milieu. It was believed that a threshold concentration of glucose was responsible for triggering the spike in the release between 3.0–5.0 g L−1, as shown in Fig. 23b.
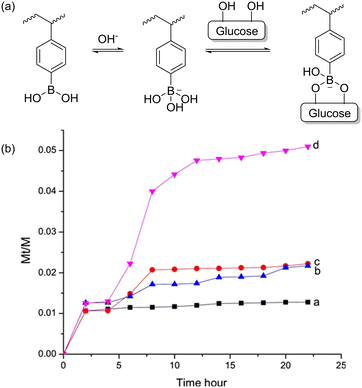 |
| Fig. 23 (a) Equilibria of poly-4-vinyl-phenylboronic acid between neutral and anionic form and complexation with glucose. (b) Release profiles of FITC-insulin from cellulose/VPBA hydrogels at 28 °C, pH = 9.0, under various glucose concentrations: 0 g L−1 (a), 1.0 g L−1 (b), 3.0 g L−1 (c) and 5.0 g L−1 (d). Reproduced from ref. 176 with permission. Copyright 2018, Elsevier. | |
2.5.2 Zwitterionic polymers.
Zwitterionic polymeric materials have attracted great interest due to their outstanding anti-fouling properties such as protein resistance179,180 and slowing the growth of microorganisms.181,182 However, currently, several limitations of zwitterionic materials such as their fragile and rigid nature, which limit their biocompatibility and utility in flexible medical devices,183,184 need to be circumvented before the potentials of these materials can be fully realised. The examples illustrated in this section aim to address the limitations of zwitterionic polymers and highlight their potential as biomedical materials.
Cheng's group designed and synthesized an all-in-one tunable zwitterionic material (poly2-((2-hydroxyethyl)(2-methacrylamidoethyl)(methyl)ammonio)acetate (pCBMAA-1)) encompassing elasticity, switchable antimicrobial–antifouling properties and stability.185 In their design, a hydroxy group was introduced on one of the side chains of the quaternary ammonium to enable switchability from the cationic antimicrobial form to the zwitterionic antifouling form, as shown in Fig. 24a. CBMAA-2 is a variation of their original zwitterionic polymer CBMAA-1, with a seven-membered ring structure in the cationic form rather than the six-membered ring in CBMAA-1. The initial synthesis of the two polymers is illustrated in Fig. 24b. The physical properties of the two polymers were examined. As shown in Fig. 24c, both PCMAA-1 (blue) and PCMAA-2 (green) could withstand higher tensile and compressive strain than the methacrylate-based polymer pCBMOH-H. This was attributed to the higher hydrophilicity and stronger hydrogen bonds of methacrylamide compared to methacrylate, resulting in a softer and more elastic material. Surface plasmon resonance (SPR) results also showed that both pCBMAA-1 and pCBMAA-2 displayed excellent antifouling properties as the amount of protein adsorbed was below the detection limit (0.3 ng cm−2) of the SPR sensor.
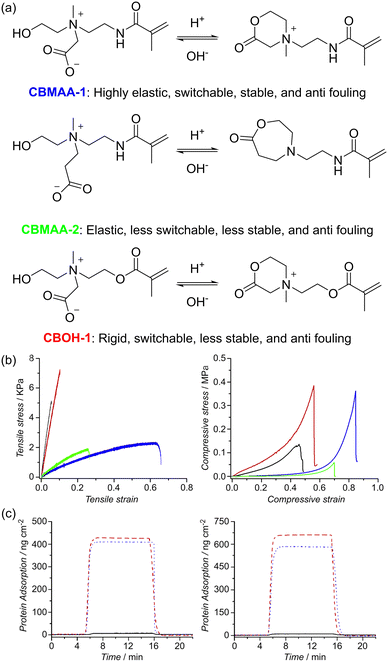 |
| Fig. 24 (a) Chemical structure and characteristics of carboxybetaine derivatives. All listed carboxybetaine derivatives show excellent antifouling properties. (b) Tensile (left) and compression (right) results for pCBMAA-1 (blue), pCBMAA-2 (green), pCBOH-1 (red), and pCBMA-2 (black) hydrogels prepared at 1.5 M (c) SPR sensorgrams showing ultralow fouling properties of zwitterionic pCBMAA-1 (left) and pCBMAA-2 (right) polymer brushes against the adsorption of 1 mg mL−1 fibrinogen (black solid line), undiluted human plasma (red dash line) and undiluted human blood serum (blue dotted line). Reproduced from ref. 185 with permission. Copyright 2013, Elsevier. | |
2.6 Thermal-induced structure switch
Heat is a common stimulus that changes the physical properties of polymers,186 in some cases due to the change in chemical structure via breaking or forming bonds, leading to even more significant changes such as changes in polymer solubility. These polymers are useful in some applications such as drug delivery,187,188 thermoelectric generators,189,190 thermal energy storage191–193 and liquid chromatography.194,195 In this section, we discuss polymers with thermal-induced chemical structural changes, with particular focus on reversible chemical transformation and phase transitions, which can act as a new type of phase-change material.196,197 Also, we only give a brief overview of this topic, and the interested reader is referred to other comprehensive reviews.198,199
2.6.1 Diels–Alder cycloaddition.
One of the most well-established reversible temperature-dependent reactions is the Diels–Alder (DA) cycloaddition. Briefly, cycloaddition typically occurs between electron-rich dienes, such as furans and pyrroles, with electron-deficient dienophiles, such as maleimides. These classical building blocks have been widely utilized in the synthesis of a variety of self-healing polymericmaterials200,201 and nanogels.202 Some examples of these polymers that are capable of undergoing reversible DA and retro-DA reactions will be discussed.
Sanyal and co-workers reported the first example of utilizing DA cycloaddition to conjugate maleimide-containing molecules on furan-containing polymer brushes under mild and reagent-free conditions.203 The reaction was found to be highly efficient, functionalising all the polymer brushes at 60 °C (Fig. 25). The reaction was also found to be reversible at 110 °C via the retro-DA pathway. As a proof of concept, a fluorescent boron-dipyrromethene (BODIPY)–maleimide dye and a protein binding ligand were prepared and conjugated on the polymer brush. Green fluorescence was clearly observed after the reaction (Fig. 25b), which was completely quenched after the retro-DA (Fig. 25c).
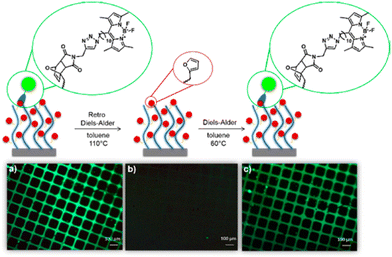 |
| Fig. 25 Fluorescence images of polymer brushes functionalized with BODIPY–maleimide (a) after Diels–Alder, (b) after retro-Diels–Alder and (c) after a second Diels–Alder reaction. Reproduced from ref. 203 with permission. Copyright 2017, the American Chemical Society. | |
Another example of reversible DA reaction was demonstrated by Du Prez and co-workers.204 Triazolinedione (TAD) reversible click chemistry was combined with microcontact interactions to print, erase and reprint polymer brushes on a surface. The polymer brushes were first fabricated via ATRP of a TAD bearing-initiator on the surface of an alkene-modified substrate; in this case, glass or silicone-supported 10-undecenyl trichlorosilane self-assembled membrane (SAM) was used. Subsequent surface-induced ATRP produced substrates functionalised with micropatterned polymethylacrylate brushes. The indole–TAD click reaction occurred at room temperature, while the reverse reaction was observed at a higher temperature of 120 °C. Upon covalent immobilization of the indole derivative on the substrate via ATRP, the desired imprinted micropattern could be observed by introducing fluorophores and examined using fluorescence microscopy. Erasure of TAD from the substrate could be achieved through an irreversible trans-click reaction by immersing the substrate in a solution of 2,4-hexadien-1-ol, regenerating the indole on the substrate. Fig. 26 illustrates the general rewritable process of the polymer brush and the substrate.
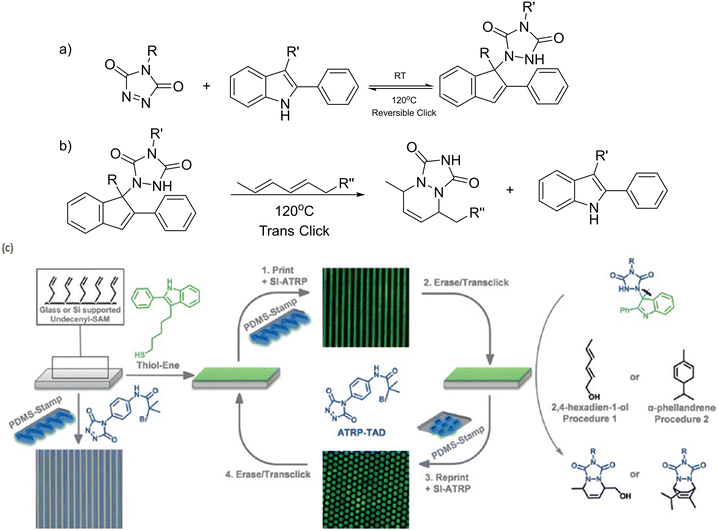 |
| Fig. 26 (a) TAD click reaction with an indole derivative, which is reversible at higher temperatures. (b) Example of a trans-click reaction: after the TAD–indole adduct is cleaved, the indole derivative is regenerated. (c) Functionalization of alkene-modified substrates by TAD click chemistry and generation of rewritable surfaces employing the trans-click approach using either 2,4-hexadien-1-ol (procedure 1) or a-phellandrene (procedure 2). Reproduced from ref. 204 with permission. Copyright 2017, Wiley. | |
2.6.2 Lower critical solution temperature (LCST)/upper critical solution temperature (UCST) polymers.
Reversibility in chemical bonds can also translate into drastic physical changes in the properties of polymers, such as solubility and miscibility of the polymer in aqueous medium. LCST refers to temperature below which polymers are fully miscible, while UCST refers to the temperature above which they are fully miscible. The presence of an LCST is often driven by unfavorable entropy changes upon mixing due to strong solvent–solute interactions. To date, the most explored polymer exhibiting LCST is poly(N-isopropylacrylamide) (PNIPAM), which exhibits an LCST with water of around 32 °C depending on the conditions. This thermal-responsive polymer is used to efficiently inhibit thermal runaways in devices such as lithium-ion batteries or supercapacitators205–210 and building applications.211 Some further examples of the applications of LCST/UCST polymers are presented in this section, although a full discussion is beyond the scope of this review, which has been thoroughly discussed elsewhere.212–214
In 2015, Chen and co-workers fabricated a thermal copolymer, poly(N-isopropylacrylamide-co-acrylamide) (PNIPAM/AM), using free radical polymerization with N-isopropylacrylamide (NIPAM) and acrylamide (AM) as monomers and bisacrylamide as a crosslinking reagent and AIBN as the polymerization initiator.215 The copolymer was soluble in aqueous solutions below 32–34 °C given that it was hydrophilic.216,217 The PNIPAM/AM solution was transformed into white hydrogels due to the disruption of the hydrogen bonds between the N-isopropyl groups and water, as shown in Fig. 27. Interestingly, the transition temperature for the phase change of the sol–gel process could be controlled by the adjusting the ratio of the monomers, i.e., PNIPAM and AM, in the copolymer mix. They utilized the polymer as an electrolyte in supercapacitors and showcased the sol–gel process of the polymer to allow suppression or migration of conductive ions in the device upon an increase or reduction of the temperature, hence regulating the overall specific capacitance and impedance of the device.
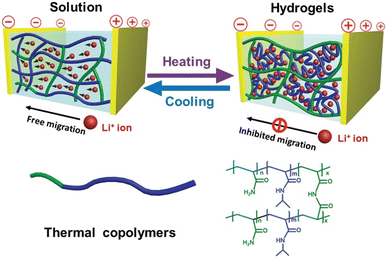 |
| Fig. 27 Illustration of sol–gel transition of electrolyte that slows the migration of conductive ions between the electrodes. Upon increasing the temperature, the electrolyte solution transforms to a hydrogel through hydrophobic association. Reproduced from ref. 215 with permission. Copyright 2015, Wiley. | |
Another example of a PNIPAM-based thermo-responsive polymer was reported by Chen and co-workers.218 PNIPAM was dissolved in an ionic liquid solvent (1-methyl-3-methylimidazolium-bis(trifluoromethylsulfonyl)imide) to form an ionogel. At room temperature, this ionogel was plastic-like and rigid with an elastic modulus of 62.5 MPa. However, when heated above the UCST (∼60.5 °C), the ionogel became transparent and soft with an elastic modulus of 0.66 kPa, representing a change in stiffness ratio by a factor of 105 with a mild temperature change. The use of the phase transition to improve mechanical properties was also demonstrated by Marcellan's group.219 Hong and Wu's group further exploited PNIPAM to prepare muscle-like hydrogels, which could be steered by light.220 This was achieved by incorporating gold nanoparticles in the polymer to enhance the photo-thermal effect. Consequently, the robot could be driven by using green laser irradiation.
2.7 Multi-stimuli-responsive structure switch
The abovementioned functional groups can be incorporated into a polymer chain to achieve dual or multi-stimuli-responsive polymer materials.7,8,221 These polymer materials enable reversible switching of their structures and properties via different stimuli for drug release, molecule sensing, healable materials, and smart surface applications. In this section, a few examples of polymer materials with a single type of multi-stimuli-responsive group or with multiple functional groups will be introduced.
Spiropyran is one of the most widely used photochromic compounds for multi-stimuli applications, boasting high responsivity to a multitude of environmental factors, such as light, pH, temperature and the presence of ions. As a distinct color change occurs during the reversible ring-opening formation of merocyanine from spiropyran, this makes it ideal to be used in the development of optical sensors.222 Zhang et al. fabricated spiropyran-modified poly(N-isopropylacrylamide)-based multi-stimuli responsive microgels.223 The microgel-based optical devices (etalons) showed reversible responsiveness to light, pH, temperature and copper(II) cation (Cu2+) concentrations. The microgels also exhibited two-photon fluorescence, highlighting their potential for applications in bioimaging. Similarly, Nhien and coworkers synthesized a novel multi-stimuli responsive amphiphilic aggregation-induced emission (AIE) copolymer using tetraphenylethylene–spiropyran monomers.224 The polymer exhibited photo-switchable properties, with ratiometric fluorescence between the green tetraphenylethylene (517 nm) and red merocyanine emissions (627 nm) the Förster resonance energy transfer (FRET) process. In the ring-opened merocyanine form, the emission of the material showed a strong dependence on pH, temperature, and cyanide anion (CN−) concentration. Furthermore, due to its good biocompatibility coupled with low limit of detection (LOD = 0.26 μM) for aqueous CN−, this material is a promising FRET sensor for the detection of CN− in living cells.
Supramolecular polymer systems are popular components in the design of multi-stimuli responsive polymers. Through the use of multiple non-covalent binding motifs, these polymers are often capable of exhibiting reversible responsiveness to a myriad of stimuli.227–229 For example, Jiang et al. reported the preparation of a stable supramolecular polymer containing tripodal pillar[5]arene tails. This supramolecular polymer could undergo a reversible gel–sol transition via pH, thermal, and force stimuli and demonstrated strong AIE after gelation (Fig. 28b).225 Moreover, the polymer also displayed strong interaction with mercuric cation (Hg2+) to form a gel, indicating its potential application to remove Hg2+ from water. Hatai and coworkers used coumarin moieties together with a central 1,3,5-trisubstituted benzene core and poly(ethylene glycol) linkers to create a multi-stimuli responsive supramolecular polymer.230,231 The material was found to be light and pH sensitive, owing to the photodimerization of coumarin and the cleavage of the hydrazone bonds, respectively. The addition of Cu2+ ions was found to induce disassembly of the nanoaggregates. By controlling the 3 stimuli (light, pH and Cu2+ concentration), the aggregation behavior of the polymer could be controlled. This allowed the polymer to successfully achieve the encapsulation and targeted release of hydrophobic molecules, such as drugs and dyes. Xiong and coworkers employed a novel AIE luminogen to design an organic multi-stimuli-responsive fluorescent material.228,232 Consisting of vinylpyridine motifs on a tetraphenylethene backbone, the AIE luminogen displayed reversible fluorescence under varying force, pH, and temperature stimuli. Due to the supramolecular intermolecular interactions and presence of a shrinkable space in the packing structure of the polymer, this material could sustain fluorescence emission even under external pressure as high as 11.25 GPa.
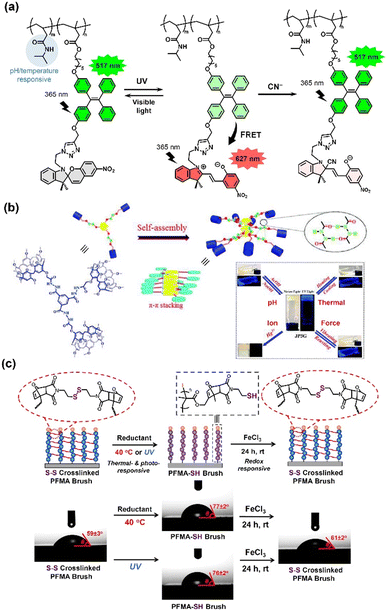 |
| Fig. 28 (a) Chemical structures of AIE-containing copolymers and their reversible energy transfer via the FRET process. The structure switch can be quenched by adding CN− ions. Adapted from ref. 224 with permission. Copyright 2020, the American Chemical Society. (b) Chemical structure and illustration of tripodal pillar[5]arene-based multi-stimuli-responsive supramolecular polymers. Adapted from ref. 225 with permission. Copyright 2018, the American Chemical Society. (c) Crosslinking and decrosslinking reactions of PFMA brush via thermal-, photo- and redox stimuli. The multi-stimuli responsive surfaces exhibited reversible and precise control of surface wettability, with images of the water contact angle measurement shown. Adapted from ref. 226 with permission. Copyright 2020, the American Chemical Society. | |
The Diels–Alder mechanism conjugates double-bond systems by reacting diene bonds with a single double bond, which is typically reversible by light and heat. This multi-stimulus-responsive conjugation has been used to impart reversibility to functional polymers. Yamamoto and co-workers demonstrated a multi-responsive reversible topologically converting polymers using anthracene and coumarin derivatives.233 Exploiting the Diels–Alder mechanism, the polymers reversibly dimerized from a linear to cyclic structure and cleaved the anthryl groups by light or heat stimuli. Poly(ethylene oxide) with electron-donating and electron-withdrawing substituted anthryl end groups was irradiated at 365 nm for photo-dimerisation. Afterwards, linearization of the polymers was successfully achieved by heating at 150 °C. Sim et al. also employed the Diels–Alder mechanism and disulfide linkage to create multi-stimuli-responsive polymer brushes.226 The authors uniquely applied bis(2-maleimidoethyl) disulfide to form a crosslinked poly(furfuryl methacrylate) (PFMA) brush gel via a Diels–Alder reaction. The disulfide bond (S–S) could cleave upon thermal or photo stimulus and rebridge under oxidative stimulus. The combination of Diels–Alder moieties and disulfide bonds allowed the polymer brush gels to be crosslinked and decrosslinked with thermal, photo and oxidative stimuli. The surface wettability and patterns could be precisely and spatially modulated and potentially applied for adsorptive/desorptive interfaces and rewritable interfaces.
The combination of functional groups and/or polymers with different stimuli-responsive properties also provide an effective method to prepare multi-stimuli responsive polymers. For example, Gebeyehu et al. developed a dual thermo- and photo-responsive supramolecular polymer based on uracil and oligomeric polypropylene glycol.234 The thermo-sensitive polypropylene backbone was end-capped with photo-sensitive uracil moieties using a one-step Michael addition reaction and self-assembly of the oligomers was initiated by double hydrogen bonding interactions between the uracil moieties upon immersion in aqueous conditions. This polymer could self-assemble to form micelles and exhibit reversible phase transition behavior in aqueous solutions. By manipulating the concentration and duration of UV irradiation and aqueous solution temperature, these micelles displayed tunable and reversible hydrophilic/hydrophobic properties, which could potentially mimic biological processes for applications in drug delivery and gene transport. Jiang and the co-workers reported the preparation of triple-stimuli-responsive polymers by incorporating a redox-responsive ferrocene unit and a pH/CO2-responsive N,N-diethylamino ethyl group.235 These polymers exhibited unique reversible phase transition behavior in an aqueous solution. For example, in an acidic environment, the polymers formed spherical particles, where the size and morphology of their aggregates changed upon oxidative stimuli given that the ferrocene segment switches their hydrophobic/hydrophilic properties.
3 Conclusions and outlook
The field of stimuli-responsive switchable polymers has progressed tremendously in the past two decades. Many of the stimuli-responsive moieties such as azobenzenes, spiropyrans, disulfide groups, and amidine groups have seen new applications, focusing on tuning their properties and their incorporation in new polymers. The fundamental understanding of the chemistry, structure, geometry and conformation of stimuli-responsive moieties as well as how these factors modulate the switchable functions and properties of the corresponding switchable stimuli-responsive polymers have also been significantly realized, allowing us to appropriately predict the properties of the polymers based on their chemical structure (as summarized in Table 1). Besides these well-known stimuli-responsive moieties, new stimuli-responsive structures have also been designed and developed, such as rotaxanes and some supramolecular polymers. The discovery of these stimuli-responsive species opens new opportunities for the development of advanced materials with desirable properties. Multi-stimuli-responsive polymer materials consisting of different stimuli-responsive moieties or a single stimuli-responsive moiety and capable of responding to different stimuli represent another interesting area to be explored. With the advancement of stimuli-responsive polymers, we envision that they will continue to find new applications beyond the drug delivery, molecular sensing and self-healing functions emphasized in this review, while other applications such as artificial muscles and chameleon-like materials have also seen significant advances. We also believe that their current limitations such as rate of response, reversibility, and selectivity will be overcome with further research. Overall, we believe that the field of stimuli-responsive polymer materials will continue to advance and play an important role in future emerging smart materials.
Table 1 Summary of working principals of stimuli-responsive polymers and their corresponding structures
Type of stimuli |
Working principal |
Typical structures |
Light |
Cis–Trans isomerism |
|
Ring opening/closure |
|
Homolytic cleavage |
|
Cycloaddition |
|
Force |
Ring opening/closure |
|
Macromolecular conformational change |
|
CO2 |
Ionic incorporation (acid/base reaction) |
|
Covalent incorporation |
|
Redox |
Oxidative cleavage |
|
Addition of oxygen |
|
Rearrangement |
|
pH |
Ring opening/closure |
|
Brønsted acid/base reaction |
|
Thermal |
Change in solvation above and below LCST/UCST |
|
Diels–Alder reaction |
|
Conflicts of interest
There are no conflicts to declare.
Acknowledgements
This work was supported and acknowledged by the Agency of Science, Technology, and Research (A*STAR) Advanced Manufacturing & Engineering – Young Individual Research Grant (AME-YIRG-A2084c0165).
Notes and references
- M. A. Stuart, W. T. Huck, J. Genzer, M. Muller, C. Ober, M. Stamm, G. B. Sukhorukov, I. Szleifer, V. V. Tsukruk and M. Urban,
et al., Emerging applications of stimuli-responsive polymer materials, Nat. Mater., 2010, 9(2), 101 CrossRef PubMed.
- M. Yoshida and J. Lahann, Smart Nanomaterials, ACS Nano, 2008, 2(6), 1101 CrossRef CAS PubMed.
- O. Sato, Dynamic molecular crystals with switchable physical properties, Nat. Chem., 2016, 8(7), 644 CrossRef CAS PubMed.
- F. Guo and Z. Guo, Inspired smart materials with external stimuli responsive wettability: a review, RSC Adv., 2016, 6(43), 36623 RSC.
- J. Zhuang, M. R. Gordon, J. Ventura, L. Li and S. Thayumanavan, Multi-stimuli responsive macromolecules and their assemblies, Chem. Soc. Rev., 2013, 42(17), 7421 RSC.
- F. Liu and M. W. Urban, Recent advances and challenges in designing stimuli-responsive polymers, Prog. Polym. Sci., 2010, 35(1–2), 3 CrossRef CAS.
- K. M. Herbert, S. Schrettl, S. J. Rowan and C. Weder, 50th Anniversary Perspective: Solid-State Multistimuli, Multiresponsive Polymeric Materials, Macromolecules, 2017, 50(22), 8845 CrossRef CAS.
- X. Fu, L. Hosta-Rigau, R. Chandrawati and J. Cui, Multi-Stimuli-Responsive Polymer Particles, Films, and Hydrogels for Drug Delivery, Chem, 2018, 4(9), 2084 CAS.
- T. Wei, Z. Tang, Q. Yu and H. Chen, Smart Antibacterial Surfaces with Switchable Bacteria-Killing and Bacteria-Releasing Capabilities, ACS Appl. Mater. Interfaces, 2017, 9(43), 37511 CrossRef CAS PubMed.
- R. Liang, L. Wang, H. Yu, A. Khan, B. Ul Amin and R. U. Khan, Molecular design, synthesis and biomedical applications of stimuli-responsive shape memory hydrogels, Eur. Polym. J., 2019, 114, 380 CrossRef CAS.
- J. Cao, X. Y. Tan, N. Jia, J. Zheng, S. W. Chien, H. K. Ng, C. K. I. Tan, H. Liu, Q. Zhu and S. Wang,
et al., Designing good compatibility factor in segmented Bi0.5Sb1.5Te3 – GeTe thermoelectrics for high power conversion efficiency, Nano Energy, 2022, 96, 107147 CrossRef CAS.
- J. Cao, J. Zheng, H. Liu, C. K. I. Tan, X. Wang, W. Wang, Q. Zhu, Z. Li, G. Zhang and J. Wu, Flexible elemental thermoelectrics with ultra-high power density, Mater. Today Energy, 2022, 25, 100964 CrossRef CAS.
- M. H. Chua, X. Y. D. Soo, W. P. Goh, Z. M. Png, Q. Zhu and J. Xu, Thioxanthylium Cations: Highly Reversible Hydrochromic Mate-rials with Tunable Color and Moisture Sensitivity, Chem. – Eur. J., 2022, e202201975 CAS.
- S. Wang, P. J. Ong, S. Liu, W. Thitsartarn, M. J. B. H. Tan, A. Suwardi, Q. Zhu and X. J. Loh, Recent Advances in Host-Guest Supramolecular Hydrogels for Biomedical Applications, Chem. – Asian J., 2022, 17(18), e202200608 CAS.
- M. Ilčíková, J. Tkáč and P. Kasák, Switchable Materials Containing Polyzwitterion Moieties, Polymers, 2015, 7(11), 2344 CrossRef.
- H. Sun, C. P. Kabb, M. B. Sims and B. S. Sumerlin, Architecture-transformable polymers: Reshaping the future of stimuli-responsive polymers, Prog. Polym. Sci., 2019, 89, 61 CrossRef CAS.
- R. C. Duarte, A. A. V. Flores and M. Stevens, Camouflage through colour change: mechanisms, adaptive value and ecological significance, Philos. Trans. R. Soc., B, 2017, 372, 1724 CrossRef PubMed.
- L. M. Mathger, E. J. Denton, N. J. Marshall and R. T. Hanlon, Mechanisms and behavioural functions of structural coloration in cephalopods, J. R. Soc., Interface, 2009, 6(Suppl 2), S149 Search PubMed.
- H. Nilsson Skold, S. Aspengren and M. Wallin, Rapid color change in fish and amphibians - function, regulation, and emerging applications, Pigm. Cell Melanoma Res., 2013, 26(1), 29 CrossRef PubMed.
- J. K. Muiruri, J. C. C. Yeo, Q. Zhu, E. Y. Ye, X. J. Loh and Z. B. Li, Poly(hydroxyalkanoates): Production, Applications and End-of-Life Strategies-Life Cycle Assessment Nexus, ACS Sustainable Chem. Eng., 2022, 10(11), 3387 CrossRef CAS.
- H. J. Zhu, J. M. J. Qiang, C. G. Wang, C. Y. Chan, Q. Zhu, E. Y. Ye, Z. B. Li and X. J. Loh, Flexible polymeric patch based nanotherapeutics against non-cancer therapy, Bioact. Mater., 2022, 18, 471 CrossRef CAS PubMed.
- S. Sugiarto, R. R. Pong, Y. C. Tan, Y. Leow, T. Sathasivam, Q. Zhu, X. J. Loh and D. Kai, Advances in sustainable polymeric materials from lignocellulosic biomass, Mater. Today Energy, 2022, 26, 101022 CAS.
- E. Moulin, L. Faour, C. C. Carmona-Vargas and N. Giuseppone, From Molecular Machines to Stimuli-Responsive Materials, Adv. Mater., 2020, 32(20), e1906036 CrossRef PubMed.
- X. Cao, A. Gao, J.-t. Hou and T. Yi, Fluorescent supramolecular self-assembly gels and their application as sensors: A review, Coord. Chem. Rev., 2021, 434, 213792 CrossRef CAS.
- H. Lambert, A. C. Bonillo, Q. Zhu, Y. W. Zhang and T. C. Lee, Supramolecular gating of guest release from cucurbit 7 uril using de novo design, npj Comput. Mater., 2022, 8(1), 471–491 Search PubMed.
- X. J. Loh, V. P. N. Nguyen, N. Kuo and J. Li, Encapsulation of basic fibroblast growth factor in thermogelling copolymers preserves its bioactivity, J. Mater. Chem., 2011, 21(7), 2246 RSC.
- X. J. Loh, W. Guerin and S. M. Guillaume, Sustained delivery of doxorubicin from thermogelling poly (PEG/PPG/PTMC urethane) s for effective eradication of cancer cells, J. Mater. Chem., 2012, 22(39), 21249 RSC.
- X. J. Loh, Poly (DMAEMA-co-PPGMA): Dual-responsive “reversible” micelles, J. Appl. Polym. Sci., 2013, 127(2), 992 CrossRef CAS.
- S. Wang, J. K. Muiruri, X. Y. D. Soo, S. Liu, W. Thitsartarn, B. H. Tan, A. Suwardi, Z. Li, Q. Zhu and X. J. Loh, Bio-PP and PP-based Biocomposites: Solutions for a Sustainable Future, Chem. – Asian J., 2022, e202200972 Search PubMed.
- S. Sugiarto, R. Pong, Y. Tan, Y. Leow, T. Sathasivam, Q. Zhu, X. Loh and D. Kai, Advances in sustainable polymeric materials from lignocellulosic biomass, Mater. Today Energy, 2022, 26, 101022 CAS.
- G. S. Hartley, The Cis-form of Azobenzene, Nature, 1937, 140(3537), 281 CrossRef CAS.
- H. M. D. Bandara and S. C. Burdette, Photoisomerization in different classes of azobenzene, Chem. Soc. Rev., 2012, 41(5), 1809 RSC.
- M. Koch, M. Saphiannikova, S. Santer and O. Guskova, Photoisomers of Azobenzene Star with a Flat Core: Theoretical Insights into Multiple States from DFT and MD Perspective, J. Phys. Chem. B, 2017, 121(37), 8854 CrossRef CAS PubMed.
- T. Fujino, S. Y. Arzhantsev and T. Tahara, Femtosecond Time-Resolved Fluorescence Study of Photoisomerization of trans-Azobenzene, J. Phys. Chem. A, 2001, 105(35), 8123 CrossRef CAS.
- J. Volarić, J. Buter, A. M. Schulte, K.-O. van den Berg, E. Santamaría-Aranda, W. Szymanski and B. L. Feringa, Design and Synthesis of Visible-Light-Responsive Azobenzene
Building Blocks for Chemical Biology, J. Org. Chem., 2022, 87(21), 14319 CrossRef PubMed.
- A. A. Beharry, O. Sadovski and G. A. Woolley, Azobenzene Photoswitching without Ultraviolet Light, J. Am. Chem. Soc., 2011, 133(49), 19684 CrossRef CAS PubMed.
- F. Cheng, Y. Zhang, R. Yin and Y. Yu, Visible light induced bending and unbending behavior of crosslinked liquid-crystalline polymer films containing azotolane moieties, J. Mater. Chem., 2010, 20(23), 4888 RSC.
- Z. Jiang, M. Xu, F. Li and Y. Yu, Red-Light-Controllable Liquid-Crystal Soft Actuators via Low-Power Excited Upconversion Based on Triplet–Triplet Annihilation, J. Am. Chem. Soc., 2013, 135(44), 16446 CrossRef CAS PubMed.
- G. S. Kumar and D. C. Neckers, Photochemistry of azobenzene-containing polymers, Chem. Rev., 1989, 89(8), 1915 CrossRef CAS.
- N. Anwar, T. Willms, B. Grimme and A. J. C. Kuehne, Light-Switchable and Monodisperse Conjugated Polymer Particles, ACS Macro Lett., 2013, 2(9), 766 CrossRef CAS PubMed.
- H. Satzger, S. Spörlein, C. Root, J. Wachtveitl, W. Zinth and P. Gilch, Fluorescence spectra of trans- and cis-azobenzene – emission from the Franck–Condon state, Chem. Phys. Lett., 2003, 372(1), 216 CrossRef CAS.
- H. Ren, D. Chen, Y. Shi, H. Yu and Z. Fu, Multi-responsive fluorescence of amphiphilic diblock copolymer containing carboxylate azobenzene and N-isopropylacrylamide, Polymer, 2016, 97, 533 CrossRef CAS.
- T. Morizo and K. Kenji, Isomerization of cis-Azobenzene in the Solid Phase, Bull. Chem. Soc. Jpn., 1964, 37(9), 1284 CrossRef.
- T. Yamamoto, Y. Norikane and H. Akiyama, Photochemical liquefaction and softening in molecular materials, polymers, and related compounds, Polym. J., 2018, 50(8), 551 CrossRef CAS.
- H. S. Blair, H. I. Pague and J. E. Riordan, Photoresponsive effects in azo polymers, Polymer, 1980, 21(10), 1195 CrossRef CAS.
- H. Zhou, C. Xue, P. Weis, Y. Suzuki, S. Huang, K. Koynov, G. K. Auernhammer, R. Berger, H.-J. Butt and S. Wu, Photoswitching of glass transition temperatures of azobenzene-containing polymers induces reversible solid-to-liquid transitions, Nat. Chem., 2017, 9(2), 145 CrossRef CAS PubMed.
- T. Yoshino, M. Kondo, J.-i. Mamiya, M. Kinoshita, Y. Yu and T. Ikeda, Three-Dimensional Photomobility of Crosslinked Azobenzene Liquid-Crystalline Polymer Fibers, Adv. Mater., 2010, 22(12), 1361 CrossRef CAS PubMed.
- M. Chen, B. Yao, M. Kappl, S. Liu, J. Yuan, R. Berger, F. Zhang, H. J. Butt, Y. Liu and S. Wu, Entangled Azobenzene-Containing Polymers with Photoinduced Reversible Solid-to-Liquid Transitions for Healable and Reprocessable Photoactuators, Adv. Funct. Mater., 2019, 30(4), 1906752 CrossRef.
- Y. Yue, Y. Norikane, R. Azumi and E. Koyama, Light-induced mechanical response in crosslinked liquid-crystalline polymers with photoswitchable glass transition temperatures, Nat. Commun., 2018, 9(1), 3234 CrossRef PubMed.
- X. Lu, S. Guo, X. Tong, H. Xia and Y. Zhao, Tunable Photocontrolled Motions Using Stored Strain Energy in Malleable Azobenzene Liquid Crystalline Polymer Actuators, Adv. Mater., 2017, 29(28), 1606467 CrossRef PubMed.
- J. Zheng, Z. M. Png, S. H. Ng, G. X. Tham, E. Ye, S. S. Goh, X. J. Loh and Z. Li, Vitrimers: Current research trends and their emerging applications, Mater. Today, 2021, 51, 586 CrossRef CAS.
- A. S. Kuenstler, K. D. Clark, J. Read de Alaniz and R. C. Hayward, Reversible Actuation via Photoisomerization-Induced
Melting of a Semicrystalline Poly(Azobenzene), ACS Macro Lett., 2020, 9(6), 902 CrossRef CAS PubMed.
- Q.-T. Fu, X. Yan, T. Li, X.-Y. Zhang, Y. He, W.-D. Zhang, Y. Liu, Y. Li and Z.-G. Gu, Diarylethene-based conjugated polymer networks for ultrafast photochromic films, New J. Chem., 2019, 43(39), 15797 RSC.
- T. Fukushima, K. Tamaki, A. Isobe, T. Hirose, N. Shimizu, H. Takagi, R. Haruki, S.-i. Adachi, M. J. Hollamby and S. Yagai, Diarylethene-Powered Light-Induced Folding of Supramolecular Polymers, J. Am. Chem. Soc., 2021, 143(15), 5845 CrossRef CAS PubMed.
- M. Irie and M. Mohri, Thermally irreversible photochromic systems. Reversible photocyclization of diarylethene derivatives, J. Org. Chem., 1988, 53(4), 803 CrossRef CAS.
- M. Irie, T. Fukaminato, K. Matsuda and S. Kobatake, Photochromism of Diarylethene Molecules and Crystals: Memories, Switches, and Actuators, Chem. Rev., 2014, 114(24), 12174 CrossRef CAS PubMed.
- K. Matsuda and M. Irie, Diarylethene as a photoswitching unit, J. Photochem. Photobiol., C, 2004, 5(2), 169 CrossRef CAS.
- J. Zhang and H. Tian, The Endeavor of Diarylethenes: New Structures, High Performance, and Bright Future, Adv. Opt. Mater., 2018, 6(6), 1701278 CrossRef.
- A. M. Asadirad, S. Boutault, Z. Erno and N. R. Branda, Controlling a polymer adhesive using light and a molecular switch, J. Am. Chem. Soc., 2014, 136(8), 3024 CrossRef CAS PubMed.
- L. Oggioni, C. Toccafondi, G. Pariani, L. Colella, M. Canepa, C. Bertarelli and A. Bianco, Photochromic Polyurethanes Showing a Strong Change of Transparency and Refractive Index, Polymers, 2017, 9(9), 462 CrossRef PubMed.
- S. Ikejiri, Y. Takashima, M. Osaki, H. Yamaguchi and A. Harada, Solvent-Free Photoresponsive Artificial Muscles Rapidly Driven by Molecular Machines, J. Am. Chem. Soc., 2018, 140(49), 17308 CrossRef CAS PubMed.
- Y. Hirshberg and E. Fischer, Multiple Reversible Color Changes Initiated by Irradiation at Low Temperature, J. Chem. Phys., 1953, 21(9), 1619 CrossRef CAS.
- V. I. Minkin, Photo-, Thermo-, Solvato-, and Electrochromic Spiroheterocyclic Compounds, Chem. Rev., 2004, 104(5), 2751 CrossRef CAS PubMed.
- R. Klajn, Spiropyran-based dynamic materials, Chem. Soc. Rev., 2014, 43(1), 148 RSC.
- L. Wang and Q. Li, Photochromism into nanosystems: towards lighting up the future nanoworld, Chem. Soc. Rev., 2018, 47(3), 1044 RSC.
- L. Kortekaas and W. R. Browne, The evolution of spiropyran: fundamentals and progress of an extraordinarily versatile photochrome, Chem. Soc. Rev., 2019, 48(12), 3406 RSC.
- C. Li, A. Iscen, L. C. Palmer, G. C. Schatz and S. I. Stupp, Light-Driven Expansion of Spiropyran Hydrogels, J. Am. Chem. Soc., 2020, 142(18), 8447 CrossRef CAS PubMed.
- T. Satoh, K. Sumaru, T. Takagi and T. Kanamori, Fast-reversible light-driven hydrogels consisting of spirobenzopyran-functionalized poly(N-isopropylacrylamide), Soft Matter, 2011, 7(18), 8030 RSC.
- W. Francis, A. Dunne, C. Delaney, L. Florea and D. Diamond, Spiropyran based hydrogels actuators—Walking in the light, Sens. Actuators, B, 2017, 250, 608 CrossRef CAS.
- B. Razavi, A. Abdollahi, H. Roghani-Mamaqani and M. Salami-Kalajahi, Light- and temperature-responsive micellar carriers prepared by spiropyran-initiated atom transfer polymerization: Investigation of photochromism kinetics, responsivities, and controlled release of doxorubicin, Polymer, 2020, 187, 122046 CrossRef CAS.
- L. Zhou, Z. Chen, K. Dong, M. Yin, J. Ren and X. Qu, DNA-mediated Construction of Hollow Upconversion Nanoparticles for Protein Harvesting and Near-Infrared Light Triggered Release, Adv. Mater., 2014, 26(15), 2424 CrossRef CAS PubMed.
- S. Chen, Y. Gao, Z. Cao, B. Wu, L. Wang, H. Wang, Z. Dang and G. Wang, Nanocomposites of Spiropyran-Functionalized Polymers and Upconversion Nanoparticles for Controlled Release Stimulated by Near-Infrared Light and pH, Macromolecules, 2016, 49(19), 7490 CrossRef CAS.
- S. Takenouchi, A. Takasu, Y. Inai and T. Hirabayashi, Effects of Geometrical Difference of Unsaturated Aliphatic Polyesters on Their Biodegradability II. Isomerization of Poly(maleic anhydride-co-propylene oxide) inthe Presence of Morpholine, Polym. J., 2002, 34(1), 36 CrossRef CAS.
- Q. A. Zhu and Y. X. Lu, Facile Synthesis of Bicyclic Amidines and Imidazolines from 1,2-Diamines, Org. Lett., 2010, 12(18), 4156 CrossRef CAS PubMed.
- W. T. Neo, Q. Ye, M. H. Chua, Q. Zhu and J. Xu, Solution-Processable Copolymers Based on Triphenylamine and 3, 4-Ethylenedioxythiophene: Facile Synthesis and Multielectrochromism, Macromol. Rapid Commun., 2020, 41(21), 2000156 CrossRef CAS PubMed.
- Q. Zhu, S. Wang, X. Wang, A. Suwardi, M. H. Chua, X. Y. D. Soo and J. Xu, Bottom-up engineering strategies for high-performance thermoelectric materials, Nano-Micro Lett., 2021, 13(1), 1 CrossRef PubMed.
- Z. Q. Wan, W. M. Ren, S. Yang, M. R. Li, G. G. Gu and X. B. Lu, Reversible Transformation between Amorphous and Crystalline States of Unsaturated Polyesters by Cis-Trans Isomerization, Angew. Chem., Int. Ed., 2019, 58(49), 17636 CrossRef CAS PubMed.
- T. M. McGuire, C. Pérale, R. Castaing, G. Kociok-Köhn and A. Buchard, Divergent Catalytic Strategies for the Cis/Trans Stereoselective Ring-Opening Polymerization of a Dual Cyclic Carbonate/Olefin Monomer, J. Am. Chem. Soc., 2019, 141(34), 13301 CrossRef CAS PubMed.
- T. Hughes, G. P. Simon and K. Saito, Light-Healable Epoxy Polymer Networks via Anthracene Dimer Scission of Diamine Crosslinker, ACS Appl. Mater. Interfaces, 2019, 11(21), 19429 CrossRef CAS PubMed.
- Q. Chen, Q. Yang, P. Gao, B. Chi, J. Nie and Y. He, Photopolymerization of Coumarin-Containing Reversible Photoresponsive Materials Based on Wavelength Selectivity, Ind. Eng. Chem. Res., 2019, 58(8), 2970 CrossRef CAS.
- Y. Li, Y. Zhang, O. Rios, J. K. Keum and M. R. Kessler, Photo-responsive liquid crystalline epoxy networks with exchangeable disulfide bonds, RSC Adv., 2017, 7(59), 37248 RSC.
- L. Wu, S. Di Cio, H. S. Azevedo and J. E. Gautrot, Photoconfigurable, Cell-Remodelable Disulfide Cross-linked Hyaluronic Acid Hydrogels, Biomacromolecules, 2020, 21(12), 4663 CrossRef CAS PubMed.
- K. M. Wiggins, J. N. Brantley and C. W. Bielawski, Methods for activating and characterizing mechanically responsive polymers, Chem. Soc. Rev., 2013, 42(17), 7130 RSC.
- H. Traeger, D. J. Kiebala, C. Weder and S. Schrettl, From Molecules to Polymers—Harnessing Inter- and Intramolecular Interactions to Create Mechanochromic Materials, Macromol. Rapid Commun., 2021, 42(1), 2000573 CrossRef CAS PubMed.
- W. Qiu, P. A. Gurr, G. da Silva and G. G. Qiao, Insights into the mechanochromism of spiropyran elastomers, Polym. Chem., 2019, 10(13), 1650 RSC.
- S. K. Osler, M. E. McFadden and M. J. Robb, Comparison of the reactivity of isomeric 2H- and 3H-naphthopyran mechanophores, J. Polym. Sci., 2021, 59(21), 2537 CrossRef CAS.
- E. Izak-Nau, D. Campagna, C. Baumann and R. Göstl, Polymer mechanochemistry-enabled pericyclic reactions, Polym. Chem., 2020, 11(13), 2274 RSC.
- Y. Chen, G. Mellot, D. van Luijk, C. Creton and R. P. Sijbesma, Mechanochemical tools for polymer materials, Chem. Soc. Rev., 2021, 50(6), 4100 RSC.
- S. K. Osler, M. E. McFadden and M. J. Robb, Comparison of the reactivity of isomeric 2H- and 3H-naphthopyran mechanophores, J. Polym. Sci., 2021, 2537–2544 CrossRef CAS.
- X. J. Loh, Z.-X. Zhang, K. Y. Mya, Y.-l. Wu, C. B. He and J. Li, Efficient gene delivery with paclitaxel-loaded DNA-hybrid polyplexes based on cationic polyhedral oligomeric silsesquioxanes, J. Mater. Chem., 2010, 20(47), 10634 RSC.
- J. Y. Lim, S. S. Goh, S. S. Liow, K. Xue and X. J. Loh, Molecular gel sorbent materials for environmental remediation and wastewater treatment, J. Mater. Chem. A, 2019, 7(32), 18759 RSC.
- X. Fan, B. H. Tan, Z. Li and X. J. Loh, Control of PLA stereoisomers-based polyurethane elastomers as highly efficient shape memory materials, ACS Sustainable Chem. Eng., 2017, 5(1), 1217 CrossRef CAS.
- X. J. Loh, J. Gong, M. Sakuragi, T. Kitajima, M. Liu, J. Li and Y. Ito, Surface coating with a thermoresponsive copolymer for the culture and non-enzymatic recovery of mouse embryonic stem cells, Macromol. Biosci., 2009, 9(11), 1069 CrossRef CAS PubMed.
- T. Muramatsu, Y. Okado, H. Traeger, S. Schrettl, N. Tamaoki, C. Weder and Y. Sagara, Rotaxane-Based Dual Function Mechanophores Exhibiting Reversible and Irreversible Responses, J. Am. Chem. Soc., 2021, 143(26), 9884 CrossRef CAS PubMed.
- Y. Sagara, H. Traeger, J. Li, Y. Okado, S. Schrettl, N. Tamaoki and C. Weder, Mechanically Responsive Luminescent Polymers Based on Supramolecular Cyclophane Mechanophores, J. Am. Chem. Soc., 2021, 143(14), 5519 CrossRef CAS PubMed.
- K. Imato, R. Yamanaka, H. Nakajima and N. Takeda, Fluorescent supramolecular mechanophores based on charge-transfer interactions, Chem. Commun., 2020, 56(57), 7937 RSC.
- S. Kato, K. Ishizuki, D. Aoki, R. Goseki and H. Otsuka, Freezing-Induced Mechanoluminescence of Polymer Gels, ACS Macro Lett., 2018, 7(9), 1087 CrossRef CAS PubMed.
- T. Sumi, R. Goseki and H. Otsuka, Tetraarylsuccinonitriles as mechanochromophores to generate highly stable luminescent carbon-centered radicals, Chem. Commun., 2017, 53(87), 11885 RSC.
- F. Hoshino, T. Kosuge, D. Aoki and H. Otsuka, Mechanofluorescent polymer/silsesquioxane composites based on tetraarylsuccinonitrile, Mater. Chem. Front., 2019, 3(12), 2681 RSC.
- Y. Lin, T. B. Kouznetsova and S. L. Craig, Mechanically Gated Degradable Polymers, J. Am. Chem. Soc., 2020, 142(5), 2105 CrossRef CAS PubMed.
- H. Qian, N. S. Purwanto, D. G. Ivanoff, A. J. Halmes, N. R. Sottos and J. S. Moore, Fast, reversible mechanochromism of regioisomeric oxazine mechanophores: Developing in situ responsive force probes for polymeric materials, Chem, 2021, 7(4), 1080 CAS.
- M. F. Cunningham and P. G. Jessop, Carbon Dioxide-Switchable Polymers: Where Are the Future Opportunities?, Macromolecules, 2019, 52, 6801 CrossRef CAS.
- S. Lin and P. Theato, CO 2 - Responsive Polymers, Macromol. Rapid Commun., 2013, 34, 1118 CrossRef CAS PubMed.
- B. Jiang, Y. Zhang, X. Huang, T. Kang, S. J. Severtson, W.-J. Wang and L. Pingwei, Tailoring CO2-Responsive Polymers and Nanohybrids for Green Chemistry and Processes, Ind. Eng. Chem. Res., 2019, 58, 15088 CrossRef CAS.
-
P. G. Jessop and M. F. Cunningham, in CO2-switchable Materials, Royal Society of Chemistry, 2020, 10.1039/9781788012850-00001.
- T. Thambi, V. G. Deepagan, H. Y. Yoon, H. S. Han, S.-H. Kim, S. Son, D.-G. Jo, C.-H. Ahn, Y. D. Suh and K. Kim,
et al., Hypoxia-responsive polymeric nanoparticles for tumor-targeted drug delivery, Biomaterials, 2014, 35(5), 1735 CrossRef CAS PubMed.
- L. Phan, D. Chiu, D. J. Heldebrant, H. Huttenhower, E. John, X. Li, P. Pollet, R. Wang, C. A. Eckert and C. L. Liotta,
et al., Switchable Solvents Consisting of Amidine/Alcohol or Guanidine/Alcohol Mixtures, Ind. Eng. Chem. Res., 2008, 47, 539 CrossRef CAS.
- J. Y. Quek, P. J. Roth, R. A. Evans, T. P. Davis and A. B. Lowe, Reversible addition-fragmentation chain transfer synthesis of amidine-based, CO2-responsive homo and AB diblock (Co)polymers comprised of histamine and their gas-triggered self-assembly in water, J. Polym. Sci., Part A: Polym. Chem., 2013, 51(2), 394 CrossRef CAS.
- P. Schattling, I. Pollmann and P. Theato, Synthesis of CO2-responsive polymers by post-polymerization modification, React. Funct. Polym., 2014, 75, 16 CrossRef CAS.
- Y. Liu, P. G. Jessop, M. Cunningham, C. A. Eckert and C. L. Liotta, Switchable Surfactants, Science, 2006, 313, 958–960 CrossRef CAS PubMed.
- Q. Yan, R. Zhou, C. Fu, H. Zhang, Y. Yin and J. Yuan, CO2-responsive polymeric vesicles that breathe, Angew. Chem., Int. Ed., 2011, 50(21), 4923 CrossRef CAS PubMed.
- H. Liu, S. Lin, Y. Feng and P. Theato, CO2-Responsive polymer materials, Polym. Chem., 2017, 8(1), 12 RSC.
- L. Dong and Y. Zhao, CO2-switchable membranes: structures, functions, and separation applications in aqueous medium, J. Mater. Chem. A, 2020, 8(33), 16738 RSC.
- S. Chen, X. Lin, Z. Zhai, R. Lan, J. Li, Y. Wang, S. Zhou, Z. H. Farooqi and W. Wu, Synthesis and characterization of CO2-sensitive temperature-responsive catalytic poly(ionic liquid) microgels, Polym. Chem., 2018, 9(21), 2887 RSC.
- W. Fan, X. Tong, F. Farnia, B. Yu and Y. Zhao, CO2-Responsive Polymer Single-Chain Nanoparticles and Self-Assembly for Gas-Tunable Nanoreactors, Chem. Mater., 2017, 29(13), 5693 CrossRef CAS.
- M. Ramezanpour and A. Rezaee Shirin-Abadi, Emulsion polymerization using three types of RAFT prepared well-defined polymeric stabilizers based on 2-dimethylaminoethyl methacrylate (DMAEMA) under CO2 atmosphere: a comparative study, J. Polym. Res., 2021, 28(7), 1189–1198 Search PubMed.
- M. Mu, R. Yuan, G. Zhang, D. Wu, H. Quan, P. Han and Y. Feng, Tuning CO2-induced reversible redispersion or irreversible destabilisation for latex separation, J. Colloid Interface Sci., 2020, 573, 250 CrossRef CAS PubMed.
- Y. Jiang, T. Zhang, Z. Yi, Y. Han, X. Su and Y. Feng, Diblock, Triblock and Cyclic Amphiphilic Copolymers with CO2 Switchability: Effects of Topology, Polymers, 2020, 12(4), 984 CrossRef CAS PubMed.
- A. Rezaee Shirin-Abadi, A. Darabi, P. G. Jessop and M. F. Cunningham, Tuning the aggregation and redispersion behavior of CO2-switchable latexes by a combination of DMAEMA and PDMAEMA-b-PMMA as stabilizing moieties, Polymer, 2016, 106, 303–312 CrossRef CAS.
- A. R. Shirin-Abadi, P. G. Jessop and M. F. Cunningham, In Situ Use of Aqueous RAFT Prepared Poly(2-(diethylamino)ethyl methacrylate) as a Stabilizer for Preparation of CO2Switchable Latexes, Macromol. React. Eng., 2017, 11(1), 1600035 CrossRef.
- A. Rezaee Shirin-Abadi, A. Darabi, P. G. Jessop and M. F. Cunningham, Tuning the aggregation and redispersion behavior of CO2-switchable latexes by a combination of DMAEMA and PDMAEMA-b-PMMA as stabilizing moieties, Polymer, 2016, 106, 303 CrossRef CAS.
- J. Zhang, Y. Liu, J. Guo, Y. Yu, Y. Li and X. Zhang, A CO2-responsive PAN/PAN-co-PDEAEMA membrane capable of cleaning protein foulant without the aid of chemical agents, React. Funct. Polym., 2020, 149, 104503 CrossRef CAS.
- C. Yin, L. Dong, Z. Wang, M. Chen, Y. Wang and Y. Zhao, CO2-responsive graphene oxide nanofiltration membranes for switchable rejection to cations and anions, J. Membr. Sci., 2019, 592, 117374 CrossRef.
- H. Yin, H. Liu, W. Wang and Y. Feng, CO2-Induced Reversible Dispersion of Graphene by a Melamine Derivative, Langmuir, 2015, 31(44), 12260 CrossRef CAS PubMed.
- H. Yin, F. Zhan, Z. Li, H. Huang, P. Marcasuzaa, X. Luo, Y. Feng and L. Billon, CO2-Triggered ON/OFF Wettability Switching on Bioinspired Polylactic Acid Porous Films for Controllable Bioadhesion, Biomacromolecules, 2021, 22(4), 1721 CrossRef CAS PubMed.
- R. Liang, X. Yang, P. Y. M. Yew, S. Sugiarto, Q. Zhu, J. Zhao, X. J. Loh, L. Zheng and D. Kai, PLA-lignin nanofibers as antioxidant biomaterials for cartilage regeneration and osteoarthritis treatment, J. Nanobiotechnol., 2022, 327 CrossRef CAS PubMed.
- X. Y. D. Soo, S. Wang, C. C. J. Yeo, J. Li, X. P. Ni, L. Jiang, K. Xue, Z. Li, X. Fei and Q. Zhu, Polylactic acid face masks: Are these the sustainable solutions in times of COVID-19 pandemic?, Sci. Total Environ., 2022, 807, 151084 CrossRef CAS PubMed.
- Y. Zhang, H. Yin and Y. Feng, CO2-responsive anionic wormlike micelles based on natural erucic acid, Green Mater., 2014, 2(2), 95 CrossRef.
- V. Fischer, K. Landfester and R. Muñoz-Espí, Molecularly Controlled Coagulation of Carboxyl-Functionalized Nanoparticles Prepared by Surfactant-Free Miniemulsion Polymerization, ACS Macro Lett., 2012, 1(12), 1371 CrossRef CAS PubMed.
- P. Liu, W. Lu, W. J. Wang, B. G. Li and S. Zhu, Highly CO2/N2-switchable zwitterionic surfactant for pickering emulsions at ambient temperature, Langmuir, 2014, 30(34), 10248 CrossRef CAS PubMed.
- L. Chen, R. Liu, X. Hao and Q. Yan, CO2 - Cross-Linked Frustrated Lewis Networks as Gas-Regulated Dynamic Covalent Materials, Angew. Chem., Int. Ed., 2019, 58(1), 264 CrossRef CAS PubMed.
- G. C. Welch, R. R. San Juan, J. D. Masuda and D. W. Stephan, Reversible, Metal-Free Hydrogen Activation, Science, 2006, 314, 1124–1126 CrossRef CAS PubMed.
- L. Chen, R. Liu and Q. Yan, Polymer Meets Frustrated Lewis Pair: Second-Generation CO2 -Responsive Nanosystem for Sustainable CO2 Conversion, Angew. Chem., Int. Ed., 2018, 57(30), 9336 CrossRef CAS PubMed.
- R. Liu, Y. Wang and Q. Yan, CO2 - Strengthened Double-Cross-Linked Polymer Gels from Frustrated Lewis Pair Networks, Macromol. Rapid Commun., 2021, 42(6), e2000699 CrossRef PubMed.
- Z. Fan and H. Xu, Recent Progress in the Biological Applications of Reactive Oxygen Species-Responsive Polymers, Polym. Rev., 2020, 60(1), 114 CrossRef CAS.
- P.-H. Hsu and A. Almutairi, Recent progress of redox-responsive polymeric nanomaterials for controlled release, J. Mater. Chem. B, 2021, 9, 2179 RSC.
- X. Zhang, L. Han, M. Liu, K. Wang, L. Tao, Q. Wan and Y. Wei, Recent progress and advances in redox-responsive polymers as controlled delivery nanoplatforms, Mater. Chem. Front., 2017, 1(5), 807 RSC.
- F. Gao and Z. Xiong, Reactive Oxygen Species Responsive Polymers for Drug Delivery Systems, Front. Chem., 2021, 9, 649048 CrossRef CAS PubMed.
- J. K. Oh, Disassembly and tumor-targeting drug delivery of reduction-responsive degradable block copolymer nanoassemblies, Polym. Chem., 2019, 10(13), 1554 RSC.
- H. Zhang, X. Kong, Y. Tang and W. Lin, Hydrogen Sulfide Triggered Charge-Reversal Micelles for Cancer-Targeted Drug Delivery and Imaging, ACS Appl. Mater. Interfaces, 2016, 8(25), 16227 CrossRef CAS PubMed.
- Q. Yan and W. Sang, H2S gasotransmitter-responsive polymer vesicles, Chem. Sci., 2016, 7(3), 2100 RSC.
- P.-H. Hsu, R. Kawasaki, K. Yamana, H. Isozaki, S. Kawamura, A. Ikeda and A. Almutairi, Hydrogen Sulfide-Responsive Self-Assembled Nanogel, ACS Appl. Polym. Mater., 2020, 2(9), 3756 CrossRef CAS.
- S. Chakraborty, R. Khamrui and S. Ghosh, Redox responsive activity regulation in exceptionally stable supramolecular assembly and co-assembly of a protein, Chem. Sci., 2020, 12(3), 1101 RSC.
- P. Ju, J. Hu, F. Li, Y. Cao, L. Li, D. Shi, Y. Hao, M. Zhang, J. He and P. Ni, A biodegradable polyphosphoester-functionalized poly(disulfide) nanocarrier for reduction-triggered intracellular drug delivery, J. Mater. Chem. B, 2018, 6(44), 7263 RSC.
- X. Kuang, D. Chi, J. Li, C. Guo, Y. Yang, S. Zhou, C. Luo, H. Liu, Z. He and Y. Wang, Disulfide bond based cascade reduction-responsive Pt(IV) nanoassemblies for improved anti-tumor efficiency and biosafety, Colloids Surf., B, 2021, 203, 111766 CrossRef CAS PubMed.
- Y. Zhao, C. Simon, M. Daoud Attieh, K. Haupt and A. Falcimaigne-Cordin, Reduction-responsive molecularly imprinted nanogels for drug delivery applications, RSC Adv., 2020, 10(10), 5978 RSC.
- J. Shen, Q. Wang, J. Fang, W. Shen, D. Wu, G. Tang and J. Yang, Therapeutic polymeric nanomedicine: GSH-responsive release promotes drug release for cancer synergistic chemotherapy, RSC Adv., 2019, 9(64), 37232 RSC.
- K. Bhattacharya, S. L. Banerjee, S. Das, S. Samanta, M. Mandal and N. K. Singha, REDOX Responsive Fluorescence Active Glycopolymer Based Nanogel: A Potential Material for Targeted Anticancer Drug Delivery, ACS Appl. Bio Mater., 2019, 2(6), 2587 CrossRef CAS PubMed.
- J. J. C. Lee, S. Sugiarto, P. J. Ong, X. Y. D. Soo, X. Ni, P. Luo, Y. Y. K. Hnin, J. S. Y. See, F. Wei and R. Zheng, Lignin-g-polycaprolactone as a form-stable phase change material for thermal energy storage application, J. Energy Storage, 2022, 56, 106118 CrossRef.
- L. Yu, M. Zhang, F.-S. Du and Z.-C. Li, ROS-responsive poly(ε-caprolactone) with pendent thioether and selenide motifs, Polym. Chem., 2018, 9(27), 3762 RSC.
- S. Gao, T. Li, Y. Guo, C. Sun, B. Xianyu and H. Xu, Selenium-Containing Nanoparticles Combine the NK Cells Mediated Immunotherapy with Radiotherapy and Chemotherapy, Adv. Mater., 2020, 32(12), e1907568 CrossRef PubMed.
- L. Zhang, C. Sun, Y. Tan and H. Xu, Selenium-sulfur-doped carbon dots with thioredoxin reductase activity, CCS Chem., 2022, 4, 2239–2248 CrossRef CAS.
- X. J. Loh, S. H. Goh and J. Li, Biodegradable thermogelling poly [(R)-3-hydroxybutyrate]-based block copolymers: Micellization, gelation, and cytotoxicity and cell culture studies, J. Phys. Chem. B, 2009, 113(35), 11822 CrossRef CAS PubMed.
- Y. Wang, L. F. Hu, P. F. Cui, L. Y. Qi, L. Xing and H. L. Jiang, Pathologically Responsive Mitochondrial Gene Therapy in an Allotopic Expression-Independent Manner Cures Leber's Hereditary Optic Neuropathy, Adv. Mater., 2021, 33, 2103307 CrossRef CAS PubMed.
- L. Xu, M. Zhao, W. Gao, Y. Yang, J. Zhang, Y. Pu and B. He, Polymeric nanoparticles responsive to intracellular ROS for anticancer drug delivery, Colloids Surf., B, 2019, 181, 252 CrossRef CAS PubMed.
- W. Zhang, X. Hu, Q. Shen and D. Xing, Mitochondria-specific drug release and reactive oxygen species burst induced by polyprodrug nanoreactors can enhance chemotherapy, Nat. Commun., 2019, 10(1), 1704 CrossRef PubMed.
- J. Li, Z. Ding, Y. Li, J. Miao, W. Wang, K. Nundlall and S. Chen, Reactive oxygen species-sensitive thioketal-linked mesoporous silica nanoparticles as drug carrier for effective antibacterial activity, Mater. Des., 2020, 195, 109021 CrossRef CAS.
- E. A. Garcia, D. Pessoa and M. Herrera-Alonso, Oxidative instability of boronic acid-installed polycarbonate nanoparticles, Soft Matter, 2020, 16(10), 2473 RSC.
- E. Jager, V. Sincari, L. J. C. Albuquerque, A. Jager, J. Humajova, J. Kucka, J. Pankrac, P. Paral, T. Heizer and O. Janouskova,
et al., Reactive Oxygen Species (ROS)-Responsive Polymersomes with Site-Specific Chemotherapeutic Delivery into Tumors via Spacer Design Chemistry, Biomacromolecules, 2020, 21(4), 1437 CrossRef CAS PubMed.
- Y. Tian, M. Lei, L. Yan and F. An, Diselenide-crosslinked zwitterionic nanogels with dual redox-labile properties for controlled drug release, Polym. Chem., 2020, 11(13), 2360 RSC.
- L. Zhang, Y. Liu, K. Zhang, Y. Chen and X. Luo, Redox-responsive comparison of diselenide micelles with disulfide micelles, Colloid Polym. Sci., 2019, 297(2), 225 CrossRef CAS.
- B. Z. Hailemeskel, K. D. Addisu, A. Prasannan, S. L. Mekuria, C.-Y. Kao and H.-C. Tsai, Synthesis and characterization of diselenide linked poly(ethylene glycol) nanogel as multi-responsive drug carrier, Appl. Surf. Sci., 2018, 449, 15 CrossRef CAS.
- F. Xu, H. Li, Y. L. Luo and W. Tang, Redox-Responsive Self-Assembly Micelles from Poly(N-acryloylmorpholine-block-2-acryloyloxyethyl ferrocenecarboxylate) Amphiphilic Block Copolymers as Drug Release Carriers, ACS Appl. Mater. Interfaces, 2017, 9(6), 5181 CrossRef CAS PubMed.
- A. Höcherl, E. Jäger, A. Jäger, M. Hrubý, R. Konefał, O. Janoušková, J. Spěváček, Y. Jiang, P. W. Schmidt and T. P. Lodge,
et al., One-pot synthesis of reactive oxygen species (ROS)-self-immolative polyoxalate prodrug nanoparticles for hormone dependent cancer therapy with minimized side effects, Polym. Chem., 2017, 8(13), 1999 RSC.
- D. Bobo, K. J. Robinson, J. Islam, K. J. Thurecht and S. R. Corrie, Nanoparticle-Based Medicines: A Review of FDA-Approved Materials and Clinical Trials to Date, Pharm. Res., 2016, 33(10), 2373 CrossRef CAS PubMed.
- V. P. N. Nguyen, N. Kuo and X. J. Loh, New biocompatible thermogelling copolymers containing ethylene-butylene segments exhibiting very low gelation concentrations, Soft Matter, 2011, 7(5), 2150 RSC.
- R. Cheng, F. Meng, C. Deng, H.-A. Klok and Z. Zhong, Dual and multi-stimuli responsive polymeric nanoparticles for programmed site-specific drug delivery, Biomaterials, 2013, 34(14), 3647 CrossRef CAS PubMed.
- M. Karimi, A. Ghasemi, P. Sahandi Zangabad, R. Rahighi, S. M. Moosavi Basri, H. Mirshekari, M. Amiri, Z. Shafaei Pishabad, A. Aslani and M. Bozorgomid,
et al., Smart micro/nanoparticles in stimulus-responsive drug/gene delivery systems, Chem. Soc. Rev., 2016, 45(5), 1457 RSC.
- L. Gan, G. R. Deen, X. Loh and Y. Gan, New stimuli-responsive copolymers of N-acryloyl-N′-alkyl piperazine and methyl methacrylate and their hydrogels, Polymer, 2001, 42(1), 65 CrossRef CAS.
- X. J. Loh, B. J. H. Yee and F. S. Chia, Sustained delivery of paclitaxel using thermogelling poly (PEG/PPG/PCL urethane) s for enhanced toxicity against cancer cells, J. Biomed. Mater. Res., Part A, 2012, 100(10), 2686 CrossRef PubMed.
- E. A. Appel, M. J. Rowland, X. J. Loh, R. M. Heywood, C. Watts and O. A. Scherman, Enhanced stability and activity of temozolomide in primary glioblastoma multiforme cells with cucurbit [n] uril, Chem. Commun., 2012, 48(79), 9843 RSC.
- S. Dai, P. Ravi and K. C. Tam, pH-Responsive polymers: synthesis, properties and applications, Soft Matter, 2008, 4(3), 435 RSC.
- G. Kocak, C. Tuncer and V. Bütün, pH-Responsive polymers, Polym. Chem., 2017, 8(1), 144 RSC.
- G. Springsteen and B. Wang, A detailed examination of boronic acid–diol complexation, Tetrahedron, 2002, 58(26), 5291 CrossRef CAS.
- K. Kataoka, H. Miyazaki, M. Bunya, T. Okano and Y. Sakurai, Totally synthetic polymer gels responding to external glucose concentration: their preparation and application to on−off regulation of insulin release, J. Am. Chem. Soc., 1998, 120(48), 12694 CrossRef CAS.
- H. Peng, X. Ning, G. Wei, S. Wang, G. Dai and A. Ju, The preparations of novel cellulose/phenylboronic acid composite intelligent bio-hydrogel and its glucose, pH-responsive behaviors, Carbohydr. Polym., 2018, 195, 349 CrossRef CAS PubMed.
- D. Klemm, B. Heublein, H. P. Fink and A. Bohn, Cellulose: fascinating biopolymer and sustainable raw material, Angew. Chem., Int. Ed., 2005, 44(22), 3358 CrossRef CAS PubMed.
- S. Kitano, Y. Koyama, K. Kataoka, T. Okano and Y. Sakurai, A novel drug delivery system utilizing a glucose responsive polymer complex between poly (vinyl alcohol) and poly (N-vinyl-2-pyrrolidone) with a phenylboronic acid moiety, J. Controlled Release, 1992, 19(1), 161 CrossRef CAS.
- Z. Zhang, T. Chao, S. Chen and S. Jiang, Superlow Fouling Sulfobetaine and Carboxybetaine Polymers on Glass Slides, Langmuir, 2006, 22(24), 10072 CrossRef CAS PubMed.
- A. J. Keefe and S. Jiang, Poly(zwitterionic)protein conjugates offer increased stability without sacrificing binding affinity or bioactivity, Nat. Chem., 2012, 4(1), 59 CrossRef CAS PubMed.
- G. Cheng, G. Li, H. Xue, S. Chen, J. D. Bryers and S. Jiang, Zwitterionic carboxybetaine polymer surfaces and their resistance to long-term biofilm formation, Biomaterials, 2009, 30(28), 5234 CrossRef CAS PubMed.
- G. Cheng, H. Xue, Z. Zhang, S. Chen and S. Jiang, A Switchable Biocompatible Polymer Surface with Self-Sterilizing and Nonfouling Capabilities, Angew. Chem., Int. Ed., 2008, 47(46), 8831 CrossRef CAS PubMed.
- L. R. Carr, H. Xue and S. Jiang, Functionalizable and nonfouling zwitterionic carboxybetaine hydrogels with a carboxybetaine dimethacrylate crosslinker, Biomaterials, 2011, 32(4), 961 CrossRef CAS PubMed.
- Z. Zhang, T. Chao and S. Jiang, Physical, Chemical, and Chemical−Physical Double Network of Zwitterionic Hydrogels, J. Phys. Chem. B, 2008, 112(17), 5327 CrossRef CAS PubMed.
- B. Cao, L. Li, Q. Tang and G. Cheng, The impact of structure on elasticity, switchability, stability and functionality of an all-in-one carboxybetaine elastomer, Biomaterials, 2013, 34(31), 7592 CrossRef CAS PubMed.
- A. S. Hoffman, “Intelligent” Polymers in Medicine and Biotechnology, Artif. Organs, 1995, 19(5), 458 CrossRef CAS PubMed.
- M. A. Ward and T. K. Georgiou, Thermoresponsive Polymers for Biomedical Applications, Polymers, 2011, 3(3), 1215–1242 CrossRef CAS.
- A. K. Bajpai, S. K. Shukla, S. Bhanu and S. Kankane, Responsive polymers in controlled drug delivery, Prog. Polym. Sci., 2008, 33(11), 1088 CrossRef CAS.
- T. A. Yemata, A. K. K. Kyaw, Y. Zheng, X. Z. Wang, Q. Zhu, W. S. Chin and J. W. Xu, Enhanced thermoelectric performance of poly(3,4-ethylenedioxythiophene):poly(4-styrenesulfonate) (PEDOT:PSS) with long-term humidity stability via sequential treatment with trifluoroacetic acid, Polym. Int., 2020, 69(1), 84 CrossRef CAS.
- H. Zhou, M. H. Chua, Q. Zhu and J. W. Xu, High-performance PEDOT:PSS-based thermoelectric composites, Compos. Commun., 2021, 27, 100877 CrossRef.
- Z. M. Png, X. Y. D. Soo, M. H. Chua, P. J. Ong, A. Suwardi, C. K. I. Tan, J. W. Xu and Q. Zhu, Strategies to reduce the flammability of organic phase change Materials: A review, Sol. Energy, 2022, 231, 115 CrossRef CAS.
- Z. M. Png, X. Y. D. Soo, M. H. Chua, P. J. Ong, J. W. Xu and Q. Zhu, Triazine derivatives as organic phase change materials with inherently low flammability, J. Mater. Chem. A, 2022, 10(7), 3633 RSC.
- K. W. Shah, P. J. Ong, M. H. Chua, S. H. G. Toh, J. J. C. Lee, X. Y. D. Soo, Z. M. Png, R. Ji, J. Xu and Q. Zhu, Application of Phase Change Materials in Building Components and the use of Nanotechnology for its improvement, Energy Build., 2022, 112018 CrossRef.
- I. Tan, F. Roohi and M.-M. Titirici, Thermoresponsive polymers in liquid chromatography, Anal. Methods, 2012, 4(1), 34 RSC.
- P. Maharjan, B. W. Woonton, L. E. Bennett, G. W. Smithers, K. DeSilva and M. T. W. Hearn, Novel chromatographic separation — The potential of smart polymers, Innovative Food Sci. Emerging Technol., 2008, 9(2), 232 CrossRef CAS.
- Q. Zhu, M. H. Chua, P. J. Ong, J. J. Cheng Lee, K. Le Osmund Chin, S. Wang, D. Kai, R. Ji, J. Kong and Z. Dong,
et al., Recent advances in nanotechnology-based functional coatings for the built environment, Mater. Today Adv., 2022, 15, 100270 CrossRef CAS.
- X. Y. D. Soo, Z. M. Png, M. H. Chua, J. C. C. Yeo, P. J. Ong, S. Wang, X. Wang, A. Suwardi, J. Cao and Y. Chen, A highly flexible form-stable silicone-octadecane PCM composite for heat harvesting, Mater. Today Adv., 2022, 14, 100227 CrossRef CAS.
- M. Sponchioni, U. Capasso Palmiero and D. Moscatelli, Thermo-responsive polymers: Applications of smart materials in drug delivery and tissue engineering, Mater. Sci. Eng., C, 2019, 102, 589 CrossRef CAS PubMed.
- J. Liu, L. Jiang, S. He, J. Zhang and W. Shao, Recent progress in PNIPAM-based multi-responsive actuators: A mini-review, Chem. Eng. J., 2022, 433, 133496 CrossRef CAS.
- Y.-L. Liu and T.-W. Chuo, Self-healing polymers based on thermally reversible Diels–Alder chemistry, Polym. Chem., 2013, 4(7), 2194 RSC.
- C. Zeng, H. Seino, J. Ren, K. Hatanaka and N. Yoshie, Self-healing bio-based furan polymers cross-linked with various bis-maleimides, Polymer, 2013, 54(20), 5351 CrossRef CAS.
- C. M. Nimmo, S. C. Owen and M. S. Shoichet, Diels−Alder Click Cross-Linked Hyaluronic Acid Hydrogels for Tissue Engineering, Biomacromolecules, 2011, 12(3), 824 CrossRef CAS PubMed.
- Y. N. Yuksekdag, T. N. Gevrek and A. Sanyal, Diels-Alder “Clickable” Polymer Brushes: A Versatile Catalyst-Free Conjugation Platform, ACS Macro Lett., 2017, 6(4), 415 CrossRef CAS PubMed.
- O. Roling, K. De Bruycker, B. Vonhoren, L. Stricker, M. Korsgen, H. F. Arlinghaus, B. J. Ravoo and F. E. Du Prez, Rewritable Polymer Brush Micropatterns Grafted by Triazolinedione Click Chemistry, Angew. Chem., Int. Ed., 2015, 54(44), 13126 CrossRef CAS PubMed.
- X. Feng, J. Sun, M. Ouyang, F. Wang, X. He, L. Lu and H. Peng, Characterization of penetration induced thermal runaway propagation process within a large format lithium ion battery module, J. Power Sources, 2015, 275, 261 CrossRef CAS.
- B. Dunn, H. Kamath and J.-M. Tarascon, Electrical Energy Storage for the Grid: A Battery of Choices, Science, 2011, 334(6058), 928 CrossRef CAS PubMed.
- N.-S. Choi, Z. Chen, S. A. Freunberger, X. Ji, Y.-K. Sun, K. Amine, G. Yushin, L. F. Nazar, J. Cho and P. G. Bruce, Challenges Facing Lithium Batteries and Electrical Double-Layer Capacitors, Angew. Chem., Int. Ed., 2012, 51(40), 9994 CrossRef CAS PubMed.
- Y. Tang, J. Deng, W. Li, O. I. Malyi, Y. Zhang, X. Zhou, S. Pan, J. Wei, Y. Cai and Z. Chen,
et al., Water-Soluble Sericin Protein Enabling Stable Solid–Electrolyte Interphase for Fast Charging High Voltage Battery Electrode, Adv. Mater., 2017, 29(33), 1701828 CrossRef PubMed.
- Y. Shi, C. Lee, X. Tan, L. Yang, Q. Zhu, X. Loh, J. Xu and Q. Yan, Atomic-Level Metal Electrodeposition: Synthetic Strategies, Applications, and Catalytic Mechanism in Electrochemical Energy Conversion, Small Struct., 2022, 3(3), 2100185 CrossRef CAS.
- B.-E. Jia, A. Q. Thang, C. Yan, C. Liu, C. Lv, Q. Zhu, J. Xu, J. Chen, H. Pan and Q. Yan, Rechargeable Aqueous Aluminum-Ion Battery: Progress and Outlook, Small, 2022, 2107773 CrossRef CAS PubMed.
- V. P. Bui, H. Z. Liu, Y. Y. Low, T. Tang, Q. Zhu, K. W. Shah, E. Shidoji, Y. M. Lim and W. S. Koh, Evaluation of building glass performance metrics for the tropical climate, Energy Build., 2017, 157, 195 CrossRef.
- S.-L. Qiao, M. Mamuti, H.-W. An and H. Wang, Thermoresponsive Polymer Assemblies: From Molecular Design to Theranostics Application, Prog. Polym. Sci., 2022, 131, 101578 CrossRef CAS.
- S. Bharadwaj, B.-J. Niebuur, K. Nothdurft, W. Richtering, N. F. A. van der Vegt and C. M. Papadakis, Cononsolvency of thermoresponsive polymers: where we are now and where we are going, Soft Matter, 2022, 18(15), 2884 RSC.
- R. Hoogenboom and H. Schlaad, Thermoresponsive poly(2-oxazoline)s, polypeptoids, and polypeptides, Polym. Chem., 2017, 8(1), 24 RSC.
- H. Yang, Z. Liu, B. K. Chandran, J. Deng, J. Yu, D. Qi, W. Li, Y. Tang, C. Zhang and X. Chen, Self-Protection of Electrochemical Storage Devices via a Thermal Reversible Sol–Gel Transition, Adv. Mater., 2015, 27(37), 5593 CrossRef CAS PubMed.
- T. Tang, A. K. K. Kyaw, Q. Zhu and J. W. Xu, Water-dispersible conducting polyazulene and its application in thermoelectrics, Chem. Commun., 2020, 56(65), 9388 RSC.
- Q. Zhu, E. Yildirim, X. Z. Wang, A. K. K. Kyaw, T. Tang, X. Y. D. Soo, Z. M. Wong, G. Wu, S. W. Yang and J. W. Xu, Effect of substituents in sulfoxides on the enhancement of thermoelectric properties of PEDOT:PSS: experimental and modelling evidence, Mol. Syst. Des. Eng., 2020, 5(5), 976 RSC.
- L. Chen, C. Zhao, J. Huang, J. Zhou and M. Liu, Enormous-stiffness-changing polymer networks by glass transition mediated microphase separation, Nat. Commun., 2022, 13(1), 6821 CrossRef CAS PubMed.
- H. Guo, N. Sanson, D. Hourdet and A. Marcellan, Thermoresponsive Toughening with Crack Bifurcation in Phase-Separated Hydrogels under Isochoric Conditions, Adv. Mater., 2016, 28(28), 5857 CrossRef CAS PubMed.
- Q. L. Zhu, C. Du, Y. Dai, M. Daab, M. Matejdes, J. Breu, W. Hong, Q. Zheng and Z. L. Wu, Light-steered locomotion of muscle-like hydrogel by self-coordinated shape change and friction modulation, Nat. Commun., 2020, 11(1), 5166 CrossRef CAS PubMed.
- P. Schattling, F. D. Jochum and P. Theato, Multi-stimuli responsive polymers – the all-in-one talents, Polym. Chem., 2014, 5(1), 25 RSC.
- A. Abdollahi, H. Roghani-Mamaqani, B. Razavi and M. Salami-Kalajahi, The light-controlling of temperature-responsivity in stimuli-responsive polymers, Polym. Chem., 2019, 10(42), 5686 RSC.
- Q. M. Zhang, W. Wang, Y.-Q. Su, E. J. M. Hensen and M. J. Serpe, Biological Imaging and Sensing with Multiresponsive Microgels, Chem. Mater., 2016, 28(1), 259 CrossRef CAS.
- P. Q. Nhien, W.-L. Chou, T. T. K. Cuc, T. M. Khang, C.-H. Wu, N. Thirumalaivasan, B. T. B. Hue, J. I. Wu, S.-P. Wu and H.-C. Lin, Multi-Stimuli Responsive FRET Processes of Bifluorophoric AIEgens in an Amphiphilic Copolymer and Its Application to Cyanide Detection in Aqueous Media, ACS Appl. Mater. Interfaces, 2020, 12(9), 10959 CrossRef CAS PubMed.
- X.-M. Jiang, X.-J. Huang, S.-S. Song, X.-Q. Ma, Y.-M. Zhang, H. Yao, T.-B. Wei and Q. Lin, Tri-pillar[5]arene-based multi-stimuli-responsive supramolecular polymers for fluorescence detection and separation of Hg2+, Polym. Chem., 2018, 9(37), 4625 RSC.
- X. M. Sim, C. G. Wang, X. Liu and A. Goto, Multistimuli Responsive Reversible Cross-Linking-Decross-Linking of Concentrated Polymer Brushes, ACS Appl. Mater. Interfaces, 2020, 12(25), 28711 CrossRef CAS PubMed.
- E. Yidirim, Q. Zhu, G. Wu, T. L. Tan, J. W. Xu and S. W. Yang, Self-Organization of PEDOT:PSS Induced by Green and Water-Soluble Organic Molecules, J. Phys. Chem. C, 2019, 123(15), 9745 CrossRef.
- M. H. Chua, K. L. O. Chin, S. J. Ang, X. Y. D. Soo, Z. M. Png, Q. Zhu and J. Xu, Aggregation Induced Emission (AIE)-Active Poly (acrylates) for Electrofluorochromic Detection of Nitroaromatic Compounds, ChemPhotoChem, 2022, 6(11), e202200168 CrossRef CAS.
- P. J. Ong, Z. M. Png, X. Y. D. Soo, X. Wang, A. Suwardi, M. H. Chua, J. W. Xu and Q. Zhu, Surface modification of microencapsulated phase change materials with nanostructures for enhancement of their thermal conductivity, Mater. Chem. Phys., 2022, 277, 125438 CrossRef CAS.
- J. Hatai, C. Hirschhäuser, J. Niemeyer and C. Schmuck, Multi-Stimuli-Responsive Supramolecular Polymers Based on Noncovalent and Dynamic Covalent Bonds, ACS Appl. Mater. Interfaces, 2020, 12(2), 2107 CrossRef CAS PubMed.
- X. Y. D. Soo, Z. M. Png, X. Z. Wang, M. H. Chua, P. J. Ong, S. X. Wang, Z. B. Li, D. Z. Chi, J. W. Xu and X. J. Loh,
et al., Rapid UV-Curable Form-Stable Polyethylene-Glycol-Based Phase Change Material, ACS Appl. Polym. Mater., 2022, 4(4), 2747 CrossRef CAS.
- J. Xiong, K. Wang, Z. Yao, B. Zou, J. Xu and X. H. Bu, Multi-Stimuli-Responsive Fluorescence Switching from a Pyridine-Functionalized Tetraphenylethene AIEgen, ACS Appl. Mater. Interfaces, 2018, 10(6), 5819 CrossRef CAS PubMed.
- T. Yamamoto, S. Yagyu and Y. Tezuka, Light- and Heat-Triggered Reversible Linear-Cyclic Topological Conversion of Telechelic Polymers
with Anthryl End Groups, J. Am. Chem. Soc., 2016, 138(11), 3904 CrossRef CAS PubMed.
- B. T. Gebeyehu, S.-Y. Huang, A.-W. Lee, J.-K. Chen, J.-Y. Lai, D.-J. Lee and C.-C. Cheng, Dual Stimuli-Responsive Nucleobase-Functionalized Polymeric Systems as Efficient Tools for Manipulating Micellar Self-Assembly Behavior, Macromolecules, 2018, 51(3), 1189 CrossRef CAS.
- X. Jiang, R. Li, C. Feng, G. Lu and X. Huang, Triple-stimuli-responsive ferrocene-containing homopolymers by RAFT polymerization, Polym. Chem., 2017, 8(18), 2773 RSC.
Footnote |
† These authors contributed equally. |
|
This journal is © The Royal Society of Chemistry 2023 |
Click here to see how this site uses Cookies. View our privacy policy here.