DOI:
10.1039/D3NA00313B
(Paper)
Nanoscale Adv., 2023,
5, 4149-4157
Phytofabrication of silver nanoparticles using Averrhoa bilimbi leaf extract for anticancer activity†
Received
9th May 2023
, Accepted 4th July 2023
First published on 19th July 2023
Abstract
Averrhoa bilimbi leaf extract was successfully utilized as a reducing agent to synthesize silver nanoparticles (AgNPs) in the laboratory. The phytochemicals in the extract helped keep the silver nanoparticles stable and slowed them down. Different methods, such as UV-visible, FT-IR spectroscopies, XRD, and SEM analyses, were used to characterize the size, shape, and morphology of the nanoparticles, and the results showed that the synthesized nanoparticles were spherical and monodispersed. FTIR spectrum streaching vibrations shown stabillization of silver nanoparticles by green extract. On the other hand, these nanoparticles were labelled as Averrhoa bilimbi (AB) extract silver nanoparticles (AB-AgNPs). The biological synthesis process was proven to enhance the efficacy of the synthesized silver nanoparticles. The effectiveness of AB-AgNPs in fighting cancer could be enhanced specifically for lung cancer (A549 cell line) and breast cancer (MCF7 cell line) by optimizing the necessary conditions. The IC50 value for A549 cells was 49.52 g mL−1, while that for MCF7 cells was 78.40 g mL−1. The effect of AgNPs on both cell lines was assessed using an MTT assay, which showed a dose-dependent cytotoxicity effect. The biosynthesized AB-AgNPs hold great potential as anticancer agents. Their synthesis using Averrhoa bilimbi leaf extract as a reducing agent was proven to be successful, resulting in spherical and monodispersed nanoparticles that exhibit effective cytotoxicity against cancer cells.
1 Introduction
In medicine, nanoparticles (NPs) are classified into three main categories based on their compositions, chemical properties, and functions: metallic, non-metallic, and metal composite nanoparticles.1–5 The unique properties of nanoparticles have led to their widespread use in many high-tech industries, particularly in medicine and technology.6–8 The commonly used reducing agents in synthesizing NPs include sodium borohydride, hydrazine, and citrate. However, these chemicals can have negative environmental impacts and can be toxic to humans and wildlife. Capping agents, on the other hand, are used to stabilize the NPs and prevent them from aggregating.9,10 Commonly used capping agents include surfactants, polymers, and proteins.11–14
The use of dissolvable plant extracts has revealed promising potential in the eco-friendly synthesis of nanoparticles (NPs) owing to their dual action as both reducing and capping agents.15–18 These extracts are composed of a diverse array of natural compounds, including flavonoids, phenolic acids, and terpenoids, all of which exhibit notable reducing and capping properties. In the synthesis of silver nanoparticles (AgNPs), for instance, a natural reducing agent, such as green tea, ginger, or garlic extract, is combined with silver nitrate. Through this process, the extract serves to both reduce the silver ions to AgNPs and stabilize them by acting as a capping agent. Different plants can synthesize NPs with different properties, allowing for a wide range of applications in medicine, electronics, and catalysis.19–23
Developing the green synthesis of metallic nanoparticles is a crucial research area, along with their application studies.4,24–26 In the case of silver nanoparticles, various methods, such as chemical, photochemical, electrochemical, radiation, and biological syntheses, can be adopted. The emerging field of phyto-nanotechnology offers a sustainable, eco-friendly, and profitable approach for nanoparticle synthesis, with numerous advantages in medical and other high-tech applications.3,7,27,28 The use of natural reducing agents derived from plants or microorganisms, along with green chemistry principles, provides a promising solution for protecting the environment while advancing technology.29,30
AgNPs are commonly utilized as signal enhancers, optical receptors, intercalating materials of batteries, polarizing filters, catalysts, sensors, bio-labelling materials, anticancer, antioxidants, and antimicrobial agents. In the fight against cancer, AgNPs have been shown to reduce toxicity, enhance surface plasmon resonance, and expand electrical resistance. AgNPs possess properties that make them ideal for use as agents against cancer, inflammation, and microbes, as well as in water treatment.
In this context, silver nanoparticles have emerged as a promising therapeutic option due to their ability to target and accumulate at a higher concentration within a tumour site. Additionally, the size of the nanoparticles can be carefully controlled during synthesis to optimize their accumulation at the targeted site. One of the significant advantages of using silver nanoparticles as an anticancer drug is their high cytotoxicity to cancer cell lines, especially for smaller-sized particles. Smaller-sized silver nanoparticles have been shown to exhibit a higher level of cytotoxicity than larger particles.
Furthermore, these nanoparticles have unique chemotherapeutic properties, such as inducing apoptosis-dependent programmed cell death, even in p53-deficient cancer cells. This is a significant advantage compared to conventional cancer drugs that cannot induce cell death in such cells.31 Recent studies have also revealed that silver nanoparticles in the size range of 5–35 nm can induce apoptosis through the mitochondria and targeted drug delivery mechanisms, further highlighting their potential as a therapeutic option for cancer treatment. By leveraging the properties of silver nanoparticles, it may be possible to design effective and targeted cancer treatments with minimal toxicity to healthy cells. The present study aimed to confirm the efficacy of eco-friendly phyto-synthesis for silver nanoparticles (AgNPs) using Artemisia tschernieviana extract (ATE). The AgNPs derived from A. tschernieviana extract exhibited strong cytotoxicity against HT29 colon cancer cells, promoting enhanced apoptosis. These findings indicate the potential of phyto-synthesized AgNPs for anticancer applications. Moreover, Cr-doped ZnO nanostructures demonstrated a significant blue-shift in UV emission and suppressed deep level emission. The band gap was widened with Cr doping due to size reduction and doping effects. Additionally, the synthesized nanostructures exhibited promise for bacterial eradication.32,33
The present study used a bio-reduction method using Averrhoa bilimbi (AB) extract to synthesize AgNPs. This method is simple and convenient, and the synthesized nanoparticles were stable and within the nanometre range. Although several studies have been conducted on the green synthesis of AgNPs using leaf extract, there is a scarcity of studies on the potential anticancer and antibacterial activity of AgNPs synthesized using wild and indigenous species. AB belongs to the oxaloacetates family, and it is a herb, shrub, or small tree that is prevalent in the tropics and subtropics. In India, around 12 species have been reported, and beverages made from the leaves have reportedly been used as an antibacterial and antiscorbutic agents, and for anti-inflammation of the rectum and diabetes. A paste of the leaves is also used in the treatment of skin eruptions, itches, bites of poisonous creatures, coughs, colds, and syphilis.
The study of AgNPs has opened new horizons for the use of nanoparticles in various fields. The green synthesis of AgNPs using plant extracts can potentially reduce the environmental impact of traditional synthetic methods. The present study on the bio-reduction method using AB extract to synthesize AgNPs provides a simpler and convenient method for synthesizing stable AgNPs within the nanometre range. Further studies using wild and indigenous species could provide valuable information on the potential anticancer and antibacterial activity of AgNPs.
2. Experimental
2.1 Materials
Silver nitrite (AgNO3) and acetone were obtained from Sigma-Aldrich (AR grade). A Soxhlet apparatus was used for the extraction, as well as double-distilled water. Other equipment included a digital centrifuge machine, well microtiter plate, MCF-7 cell line, microscope, CO2 incubator, and gyratory shaker.
2.2 Preparation of aqueous extract from the leaves of AB
The leaves of Averrhoa bilimbi were collected from the Navanagar area of Hubballi, Karnataka, India, and were carefully washed before being dried in the shade. Once completely dry, they were finely powdered using a mortar and pestle, resulting in about 50 g of dry powder. The Soxhlet extractor was cleaned thoroughly and dried before the powder was placed in the thimble. In the next step, a round-bottom flask containing 100 mL of acetone was heated to boiling point and decanted, and the extraction was carried out at a temperature of 80 °C. The extract was allowed to flow from the thimble to the round-bottom flask in a process that required at least 20 cycles. The resulting extract was green in colour, indicating the presence of phytochemicals. The AB extract was then filtered using Whatman No. 1 filter paper to remove any impurities and then stored at lower temperature to maintain potency. This meticulous process ensured that the AB extract was of high quality and ready for use in further research or applications.
2.3 Synthesis of silver nanoparticles (AB-AgNPs)
To synthesize the silver nanoparticles using Averrhoa bilimbi extract, a 1 mM concentrated silver nitrate solution was prepared through a standard laboratory procedure. The AB extract was then mixed with the silver nitrate solution in a 1
:
5 ratio with proper stirring. Upon mixing, there was an immediate change in colour of the mixture from light green to chrome yellow, which eventually turned brown over time. The mixture was then left to remain stable at room temperature for 24 h, after which the AB-AgNPs were obtained in a powdered, dried form for further studies (Fig. 1 and 2). This preliminary observation was the foundation for confirming the synthesized AB-AgNPs using nanometrological tools. It was important to confirm the synthesis of the nanoparticles to ensure that they had the desired properties and characteristics necessary for their potential use in biomedical applications, such as anticancer and antimicrobial applications.
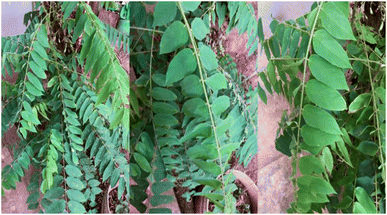 |
| Fig. 1
Averrhoa bilimbi leaves (location Dharwad). | |
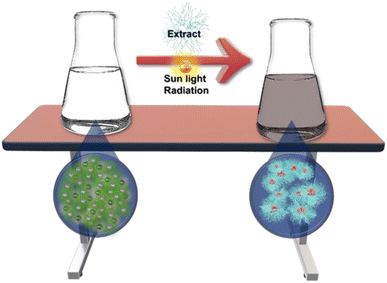 |
| Fig. 2 Schematic representation of the synthesis of AB-AgNPs. | |
3. Results and discussion
In recent years, the use of nanoparticles has become a promising approach in the field of medicine, particularly in cancer treatment. Silver nanoparticles, one of the many varieties of nanoparticles, have drawn interest because of their distinct characteristics and possible uses in the treatment of cancer. This study aimed to investigate the biosynthesis of AB extract silver nanoparticles (AB-AgNPs) and to evaluate their potential effect on cancer cell lines.
3.1 Phytochemical analysis
The leaf extract of AB in water was found to contain several phytochemicals that play a significant role in reducing and capping AgNPs. Qualitative analysis revealed diverse secondary metabolites, which show promise for various biomedical and industrial applications. These findings are described in SI 1 of the ESI.† The biosynthesis of AgNPs from the AB extract involved the reduction of Ag+ ions to Ag0 atoms. The extract's unique combination of phytochemicals can act as reducing agents and stabilizers, preventing the nanoparticles from aggregating and facilitating their uniform dispersion in an aqueous medium. This study highlights the potential of Averrhoa bilimbi leaf extract as a sustainable and eco-friendly alternative to conventional chemical methods for the synthesis of silver nanoparticles. Natural plant extracts can reduce the environmental impact from the synthesis of nanoparticles and provide a cost-effective and scalable approach for synthesizing nanoparticles with desired properties.
3.2 Nanometrology of silver nanoparticles
During the reaction process, UV-visible spectroscopy was utilized to monitor the progress of the synthesis, and the data were recorded using a UV-160V spectrophotometer. This method allowed for the measurement of the absorption spectra of the samples in the ultraviolet and visible regions of the electromagnetic spectrum, providing information about the electronic transitions and chemical nature of the synthesized nanoparticles.
The dried AB-AgNPs sample was subjected to FTIR analysis after synthesis to investigate the presence of the biomolecules involved in the synthesis process. The functional groups and chemical bonds responsible for the synthesis and stability of the nanoparticles were revealed by the FTIR spectra. Various techniques were utilized to characterize the nanoparticles further, including XRD, SEM, EDX, and TEM. SEM and TEM were used to observe the nanoparticles' size, shape, and distribution, while EDX was used to analyze their elemental composition. The crystallographic orientation and lattice spacing of the produced nanoparticles were studied using XRD to determine their crystalline structure. The combination of these analytical techniques allowed for the comprehensive characterization of the AB-AgNPs synthesized using the aqueous leaf extract of Averrhoa bilimbi. These findings provide insights into the mechanisms involved in the green synthesis of AgNPs using natural extracts and their potential applications in various fields, such as medicine, electronics, and catalysis.
3.3 UV-visible spectroscopy analysis
Surface plasmon resonance gives noble metal nanoparticles, like silver, gold, platinum, and copper, distinctive optical characteristics (SPR). Free electrons collectively oscillate in response to certain frequency light radiation, causing nanoparticles to absorb light energy. This phenomenon is only observed in metal nanoparticles because the conduction and valence bands of metals are very close, allowing electrons to move freely between them. As a result, the SPR absorption peak is a distinctive feature of synthesized noble metal nanoparticles. Controlling the size, shape, and morphology of metal nanoparticles is critical in their synthesis. The Mie theory explains the dependence of the SPR absorption peak on various factors, such as the particle size, surrounding dielectric medium, and chemical conditions. As the size of the nanoparticles decreases, the SPR peak shifts towards the shorter wavelength side. This effect has been experimentally and theoretically demonstrated and has significant implications in the design and application of metal nanoparticles.
In recent studies, the SPR absorption peak of noble metal nanoparticles was observed in the visible range at 460 nm, which is a promising result compared to theoretical predictions (Fig. 3). This finding suggests that the synthesis and control of noble metal nanoparticles can be optimized to achieve specific optical properties. Furthermore, the SPR phenomenon offers a unique platform for developing novel optical sensing and imaging applications, including bioimaging and biosensing. The surface plasmon resonance phenomenon exhibited by noble metal nanoparticles is a fascinating field of research that offers significant potential for developing new technologies and applications. The ability to control the size, shape, and morphology of metal nanoparticles, and their optical properties, provides opportunities for designing advanced materials with tailored properties for specific applications.
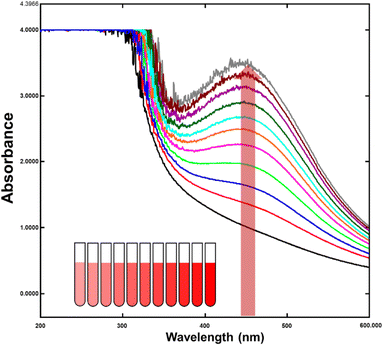 |
| Fig. 3 UV-visible spectrum of AB-AgNPs. | |
3.4 X-ray diffraction analysis
To identify the chemical composition and crystalline nature of the AB-AgNPs, X-ray diffraction (XRD) analysis was conducted. This technique is widely used to investigate the crystal structure of materials, including nanoparticles. The XRD study enabled the identification and estimation of the distinct crystallographic structure of the material founds in the AB extract, as well as the particle sizes of the synthesized AgNPs. The XRD spectra obtained from the AB-AgNPs indicated their crystalline nature, and the oxidation state of the particles was determined as a function of time. The results revealed the presence of silver nanoparticles in the nanometric range (Fig. 4). The average particle size was calculated using the Debye–Scherrer formula based on the prominent peaks observed at 45.1° and 64.7°. The XRD pattern exhibited five main diffraction peaks, which corresponded to the (111), (200), (120), (202), and (311) planes. The existence of these peaks in the AgNPs biosynthesized using AB extract was verified by the TEM images. The XRD peak patterns were considerably impacted by the size of the nanoparticles, highlighting the necessity to regulate the particle size during their synthesis.
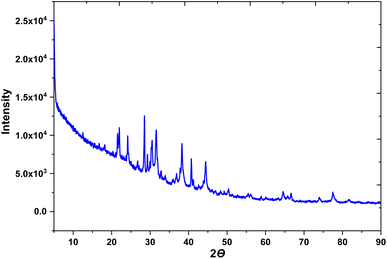 |
| Fig. 4 XRD diffractograms of the AB-AgNPs. | |
FTIR studies were performed and identified various phytochemicals in the AB extract that acted as reducing agents and that were responsible for stabilizing the AgNPs. These phytochemicals played a crucial role in providing the crystalline structure of AgNPs and have been extensively studied in various biosynthesized nanoparticles. XRD analysis is an indispensable technique for determining nanoparticles' chemical composition and crystalline structure. This study provided valuable information regarding the synthesis and properties of the AB-AgNPs, emphasizing the importance of controlling the particle size and the role of the phytochemicals in the nanoparticles' synthesis.
3.5 Fourier transform infrared spectroscopy (FTIR)
FTIR spectroscopy is an important analytical tool in exploring plant extracts' role in reducing silver. The technique involves using infrared radiation to evaluate the surface chemistry of nanoparticles synthesized from plant extracts. The absorption and transmittance values of infrared radiation can be used to understand the identity of a sample and the functional atoms and chemical bonds of the phytochemicals present. Fig. 5 depicts the synthesis of the AB-AgNPs when utilizing plant extracts as reducing agents. The presence of phytoconstituents that function as capping agents was revealed in the FTIR spectra of the AB-AgNPs by the absorption peaks at 3309.15, 2927, 1602, 1075, and 538 cm−1. These peaks were later corroborated at 3444, 1722, 1621, 1388, 1055, and 587 cm−1 wavelengths.
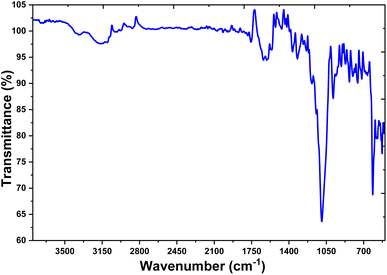 |
| Fig. 5 FTIR spectrum of AB-AgNPs. | |
The “polymeric” –OH stretching mode was represented by the absorption peak at 3444 cm−1, whereas the usual aldehydic group was represented by the peak at 1722 cm−1. The blank peak at 1621 cm−1 corresponded to the amide, while the strong peaks at 1055 cm−1 were related to aliphatic fluoro compound –C
C and –C
F stretches. The peaks at 587 cm−1 indicated the out-of-plane bending of alcohol and –OH. The majority of these peaks corresponded to the phenolic groups of polyphenols, triterpenoids, alkaloids, steroids, carbohydrates, and tannins, all of which were present in sufficient amounts in the leaf extract. These phytochemicals could act as both capping and reducing agents, facilitating the formation of AB-AgNPs. FTIR spectroscopy is thus a simple and appropriate method for determining the plant extracts role in reducing silver nanoparticles in the present study. The absorption bands obtained from the analysis provided valuable information regarding the surface chemistry of the synthesized nanoparticles, which could be used to optimize the synthesis process and enhance the quality of the final product.
3.6 Scanning electron microscopy (SEM) analysis
The morphology of the AB-AgNPs plays crucial in determining their physicochemical properties and potential applications. In this study, we thoroughly examined the morphology of the AB-AgNPs using scanning electron microscopy (SEM). Our analysis revealed that the AB-AgNPs were uniformly distributed and exhibited a spherical shape, with a 3–5 nm size range. These nanoscale dimensions are crucial for their potential applications in nanotechnology and biomedicine. Moreover, we conducted elemental analysis using energy-dispersive X-ray spectroscopy (EDX), which revealed a strong absorption peak of silver at 3 keV. This peak indicated the high concentration of silver, which is the primary constituent of the AB-AgNPs. Fig. 6 further confirmed the uniform distribution of the AB-AgNPs through microscopic analysis by SEM. These synthesized AB-AgNPs exhibited promising properties and the potential to be used in various applications, such as drug delivery, biosensing, and imaging.
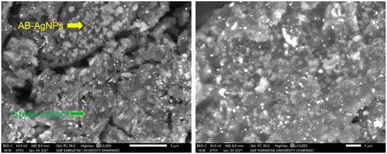 |
| Fig. 6 SEM images of the AB-AgNPs samples. | |
3.7 Transmission electron microscopy (TEM) analysis
Transmission electron microscopy (TEM) is an advanced scientific technique that is widely used to study the morphology and size range of silver nanoparticles (AgNPs). TEM has a high resolving power that allows for the detection of nanoparticles through their interaction with the electron beam and subsequent imaging on a photographic plate. The morphological features of AgNPs can be characterized accurately by TEM analysis, enabling the determination of their size and shape. To perform TEM analysis, a very thin sample is required as electrons need to transmit through it. This tool is useful for studying the topographical structure of nanoparticles and identifying their shape, size, and morphology. In the case of the AB-AgNPs, TEM analysis revealed that they were spherical and crystalline in shape. The size of these nanoparticles was less than 5 nanometres, as determined by imaging with ImageJ software.
The TEM image of the AB-AgNPs showed they had a polycrystalline structure with distinct boundaries. Their crystallinity was observed through the selected area electron diffraction (SAED) pattern and lattice pattern of the AB-AgNPs, as shown in Fig. 7, whereby each grain had different lattice planes oriented in different directions, confirming the polycrystallinity of the synthesized AB-AgNPs. The SAED pattern exhibited various concentric bright-coloured rings, including (111), (200), (220), and (311), which matched with the planes observed for the X-ray diffraction (XRD) analysis, indicating a face-cantered cubic lattice (fcc). TEM is a powerful technique for characterizing nanoparticles' size, shape, and morphology. By providing a high-resolution image of the polycrystalline structure of the AB-AgNPs, TEM has enabled researchers to understand these nanoparticles' properties better. Such insights are essential for developing new nanoparticle applications in various fields, including medicine, electronics, and environmental science.
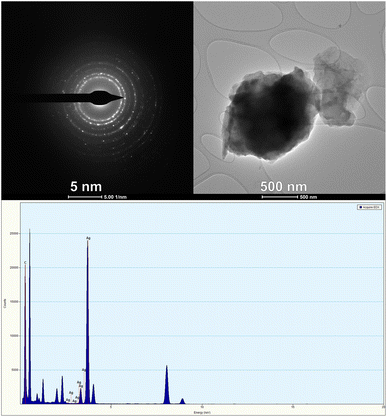 |
| Fig. 7 TEM images of AB-AgNPs and TEM-EDX of the Ag content in AB-AgNPs. | |
3.8 Cytotoxicity analysis against the MCF-7 and A549 cell lines
The study delved into the effects of silver nanoparticles synthesized from Averrhoa bilimbi leaf extract on two cancer cell lines: A549 and MCF7 (Fig. 8). Earlier reports on silver nanoparticles synthesized by Anthemis atropatana reported the particles had an average size of 10–80 nm, and showed efficient IC50 values on the MCF-7 cell line at 20 μg mL−1, while on the HT29 cancer cells line, the observed IC50 value was 63.2. For Rhynchosia suaveolens and Artemisia nilagirica, with particle sizes of 21.22 nm, on MCF7 at the 0.15 μg mL−1 for silver nanoparticles synthesized from Lavandula officinalis, obtained particles size is 100 nm on U87MG cells. Their IC50 value, shows 7.536 μg mL−1.
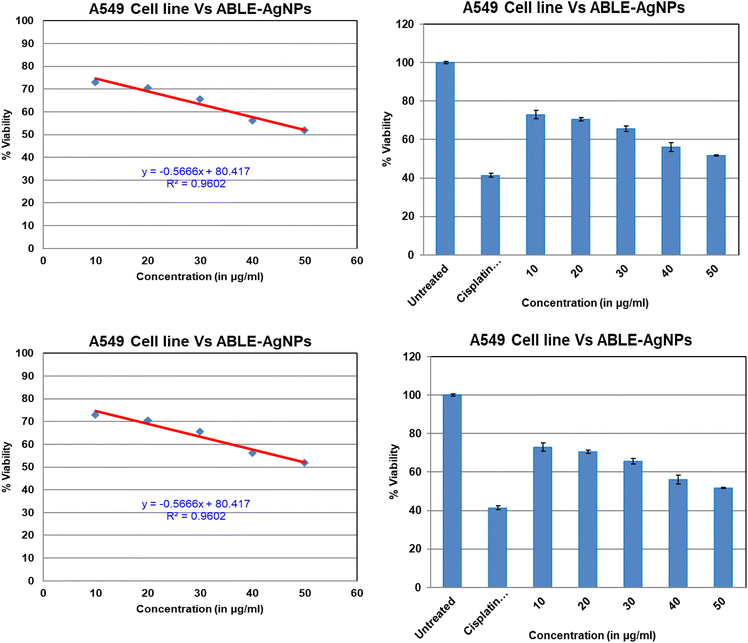 |
| Fig. 8 Variation of the percentage viability vs. concentration of AB-AgNPs. | |
The findings revealed that the nanoparticles exhibited cytotoxic properties towards both cell lines, with MCF-7 cells undergoing significant morphological changes upon exposure. The cell membrane integrity was compromised, impeding cell growth. Distinctive cytoplasmic shrinkage and cell clustering were also evident, which were not observed in normal cells treated with AgNPs. The therapeutic potency of plant-based AgNPs was evaluated against the MCF-7 and A549 cell lines through the MTT assay, which measured the cell viability after continuous treatment with various doses of AB-AgNPs for 24 h. Phase-contrast microscope images captured the cell morphology and density. The results demonstrated that cancer cell growth inhibition was greatly effective with increasing the concentrations of the AgNPs. The inhibitory concentration (IC50) of the phyto-mediated AgNPs was recorded at 78.40 μg mL−1 against MCF7 and at 49.52 μg mL−1 for A549 cells. The cell viability decreased as the concentration of phyto-mediated AgNPs increased.
The study suggests that silver nanoparticles may stimulate reactive oxygen species and affect apoptosis by damaging the cellular components. A unique ability of AgNPs as a chemotherapeutic agent was shown to be apoptosis-dependent regulated cell fatality with the apparent lack of the tumour suppressor p53. This way AgNPs acts as a typical cancer drug that kill p53-deficient cancer cells. Also, the nanoparticles ranging from 5 to 35 nm induced apoptosis through the mitochondria and targeted drug delivery action of the AgNPs. These findings highlight the potential of plant-based AgNPs as a promising candidate for developing anticancer drugs. However, further studies are required to evaluate the efficacy and safety of these nanoparticles before their clinical applications. Overall, this study provides valuable insights into the therapeutic potential of plant-based AgNPs against cancer cells and paves the way for future research in this field.
3.9 Mechanism of the anticancer activity of AB-AgNPs
The mechanism of apoptosis of cancer cells is a complex process achieved through various pathways. One of the most common pathways for the induction of apoptosis is the generation of reactive oxygen species (ROS). The generation of ROS is influenced by the size of the silver nanoparticles used in the process. Fig. 9 shows a three-step signal transduction process that causes cellular apoptosis. The first step involves the reception of a chemical signal through binding of the AB-AgNPs with the receptor protein (p53), which transfers the signal into the cell's nucleus. The p53 protein, a tumour suppressor, regulates cell division and prevents uncontrolled growth. Mutations in p53 are found in around 50% of human tumour cells, whereas normal cells have low levels of p53 protein. The second step involves the activation of other second messenger proteins by the primary receptor proteins. These second messengers further stimulate the message to the nucleus and other cell components. This activation process requires energy from ATP, which activates enzymes that perform metabolic reactions. The series of enzyme activations are collectively known as signal transduction pathways. Finally, the third step involves the cellular response to counteract the activation process, leading to the disturbance of the mitochondrial trans-membrane potential. This leads to the suppression of the respiration process of a cell, ultimately resulting in cell death. The use of silver nanoparticles in inducing the apoptosis of cancer cells is a promising area of research. The specific mechanism of action, as outlined in Fig. 9, provides a targeted approach to treating cancer cells while minimizing damage to healthy cells. However, further research is needed to fully understand the effects of silver nanoparticles on healthy cells and their potential for use in clinical applications.34–36
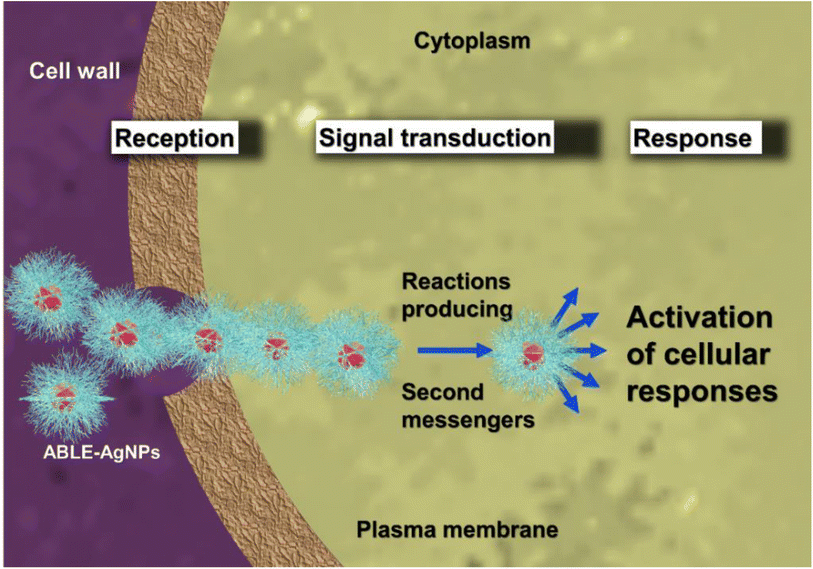 |
| Fig. 9 Schematic representation of the mechanism of ROS generation by AB-AgNPs on cancer cell lines. | |
Recent research has revealed optimistic outcomes in producing silver nanoparticles (AgNPs) through the ecological amalgamation of Mentha arvensis, a herb commonly referred to as “corn mint.” The investigation revealed that the synthesized AgNPs possess the potential to induce cytotoxicity in breast cancer cells, especially by encouraging caspase-9-mediated apoptosis in MCF-7 cells.37
The selective cancer cell death process is based on the percentage of silver nanoparticles released into the cells, also known as targeted drug delivery. The concentration of silver nanoparticles released in normal and cancer cell lines varies at different pH levels. The release percentage is higher at lower pH levels, which confirms that cancer cells are selectively targeted and killed.38–40 The aggregation of AgNPs in cancer cells also increases cellular viscosity, and disturbs significant cellular processes, leading to cellular apoptosis. Caspase 9, an enzyme responsible for initiating programmed cell death, is activated during this process. The use of green synthesis, particularly from plants, offers several benefits over conventional synthesis methods, such as cost-effectiveness, eco-friendliness, and minimal toxicity.9,41,42 This approach also allows for developing targeted drug delivery systems, which would be particularly useful in cancer treatment, where selective cell death is crucial. Further research is needed to fully understand the potential for the green synthesis of AgNPs in cancer treatment. However, these findings provide a promising step towards developing more effective and targeted cancer therapies while minimizing the adverse side effects on healthy cells.43
4. Conclusions
The use of AB extract to synthesize silver nanoparticles is an eco-friendly and safe process. The resulting AB-AgNPs were analyzed using various techniques, including UV-vis, FTIR, XRD, and SEM. The surface plasmon resonance observed at 460 nm and the presence of hydroxyl and carbonyl groups in the phytochemicals of the AB extract, as indicated by the FTIR measurements, were responsible for both reducing and capping the nanoparticles. SEM studies confirmed the formation of nanoclusters with particle sizes ranging from 50 to 90 nm, consistent with the XRD results that showed a face-centred cubic (fcc) structure, as confirmed by the standard JCPDS data. The AB-AgNPs were evaluated for their potential to suppress the growth of lung cancer (A549 cell line) and breast cancer (MCF7) cell lines under optimized conditions. The lowest concentrations of AB-AgNPs resulted in IC50 values of 49.52 μg mL−1 and 78.40 μg mL−1 against A549 and MCF7 cells, respectively. The cytotoxic effect of AB-AgNPs on both cancer cell lines was dose-dependent and confirmed through MTT assay. Therefore, the biosynthesized AB-AgNPs show promise as potential anticancer agents.
Author contributions
Conceptualization: Leena V. Hublikar and S. V. Ganachari; software: S. V. Ganachari and V. B. Patil; validation: S. V. Ganachari and V. B. Patil; investigation: S. V. Ganachari; resources: Leena V. Hublikar, S. V. Ganachari and V. B. Patil; writing – original draft: Leena V. Hublikar; writing – review & amp; editing: S. V. Ganachari and V. B. Patil; visualization: Leena V. Hublikar, S. V. Ganachari and V. B. Patil; supervision: S. V. Ganachari.
Conflicts of interest
There are no conflicts to declare.
References
- S. Sarli, M. R. Kalani and A. Moradi, Int. J. Nanomed., 2020, 15, 3791–3801 CrossRef CAS PubMed.
- A. Andleeb, A. Andleeb, S. Asghar, G. Zaman, M. Tariq, A. Mehmood, M. Nadeem, C. Hano, J. M. Lorenzo and B. H. Abbasi, Cancers, 2021, 13, 2818 CrossRef CAS PubMed.
- L. Xu, Y.-Y. Wang, J. Huang, C.-Y. Chen, Z.-X. Wang and H. Xie, Theranostics, 2020, 10, 8996–9031 CrossRef CAS PubMed.
- G. Lakshmanan, A. Sathiyaseelan, P. T. Kalaichelvan and K. Murugesan, Karbala Int. J. Mod. Sci., 2018, 4, 61–68 CrossRef.
- M. Matysiak-Kucharek, M. Czajka, B. Jodłowska-Jędrych, K. Sawicki, P. Wojtyła-Buciora, M. Kruszewski and L. Kapka-Skrzypczak, Molecules, 2020, 25, 2375 CrossRef CAS PubMed.
- R. S. Hamida, G. Albasher and M. M. Bin-Meferij, Cancers, 2020, 12, 1–25 CrossRef PubMed.
- M. G. González-Pedroza, L. Argueta-Figueroa, R. García-Contreras, Y. Jiménez-Martínez, E. Martínez-Martínez, S. A. Navarro-Marchal, J. A. Marchal, R. A. Morales-Luckie and H. Boulaiz, Nanomaterials, 2021, 11, 1273 CrossRef PubMed.
- M. Rozalen, M. Sánchez-Polo, M. Fernández-Perales, T. J. Widmann and J. Rivera-Utrilla, RSC Adv., 2020, 10, 10646–10660 RSC.
- Y. Ju, H. Liao, J. J. Richardson, J. Guo and F. Caruso, Chem. Soc. Rev., 2022, 51, 4287–4336 RSC.
- R. K. Sharma, S. Yadav, S. Dutta, H. B. Kale, I. R. Warkad, R. Zbořil, R. S. Varma and M. B. Gawande, Chem. Soc. Rev., 2021, 50, 11293–11380 RSC.
- D. Borah, N. Das, P. Sarmah, K. Ghosh, M. Chandel, J. Rout, P. Pandey, N. N. Ghosh and C. R. Bhattacharjee, Mater. Today Commun., 2023, 34, 105110 CrossRef CAS.
- S. R. Pavan, J. Venkatesan and A. Prabhu, J. Drug Delivery Sci. Technol., 2022, 74, 103525 CrossRef.
- S. Majeed, M. Saravanan, M. Danish, N. A. Zakariya, M. N. M. Ibrahim, E. H. Rizvi, S. un NisaAndrabi, H. Barabadi, Y. K. Mohanta and E. Mostafavi, Talanta, 2023, 253, 124026 CrossRef CAS.
- C. S. Madhu, K. S. Balaji, J. Shankar, S. N. S. Gowda and A. C. Sharada, J. Drug Delivery Sci. Technol., 2022, 72, 103329 CrossRef CAS.
- L. V. Hublikar, S. V. Ganachari, V. B. Patil, S. Nandi and A. Honnad, Prog. Biomater., 2023, 12, 155–169 CrossRef CAS PubMed.
- L. V. Hublikar, S. V. Ganachari and V. B. Patil, Environ. Sci. Pollut. Res., 2023, 30, 66994–67007 CrossRef CAS PubMed.
-
S. V. Ganachari, J. S. Yaradoddi, S. B. Somappa, P. Mogre, R. P. Tapaskar, B. Salimath, A. Venkataraman and V. J. Viswanath, in Handbook of Ecomaterials, 2019, vol. 4, pp. 2681–2698 Search PubMed.
-
S. V. Ganachari, N. R. Banapurmath, B. Salimath, J. S. Yaradoddi, A. S. Shettar, A. M. Hunashyal, A. Venkataraman, P. Patil, H. Shoba and G. B. Hiremath, in Handbook of Ecomaterials, 2019, vol. 1, pp. 83–103 Search PubMed.
- H. S. Ahmad, M. Ateeb, S. Noreen, M. I. Farooq, M. M. F. A. Baig, M. S. Nazar, M. F. Akhtar, K. Ahmad, A. R. Ayub, H. Shoukat, F. Hadi and A. Madni, J. Mol. Struct., 2023, 1282, 135196 CrossRef CAS.
- M. Majeed, K. R. Hakeem and R. U. Rehman, Chemosphere, 2022, 288, 132527 CrossRef CAS PubMed.
- M. A. El-Naka, A. El-Dissouky, G. Y. Ali, S. Ebrahim and A. Shokry, Talanta, 2023, 253, 123908 CrossRef CAS PubMed.
- N. N. Farshori, M. M. Al-Oqail, E. S. Al-Sheddi, S. M. Al-Massarani, Q. Saquib, M. A. Siddiqui, R. Wahab and A. A. Al-Khedhairy, J. Drug Delivery Sci. Technol., 2022, 70, 103260 CrossRef CAS.
- P. Hanachi, Z. Gharari, H. Sadeghinia and T. R. Walker, J. Mol. Struct., 2022, 1265, 133325 CrossRef CAS.
- R. Bhat, R. Deshpande, S. V. Ganachari, D. S. Huh and A. Venkataraman, Bioinorg. Chem. Appl., 2011, 2011, 650979 Search PubMed.
- S. V. Ganachari, R. Bhat, R. Deshpande and A. Venkataraman, Bionanoscience, 2012, 2, 316–321 CrossRef.
- V. M. Ankegowda, S. P. Kollur, S. K. Prasad, S. Pradeep, C. Dhramashekara, A. S. Jain, A. Prasad, C. Srinivasa, P. B. S. Setty, S. M. Gopinath, P. S. Rajendra, A. H. Bahkali, A. Syed and C. Shivamallu, Molecules, 2020, 25, 5042 CrossRef CAS PubMed.
- E. M. Halawani, A. M. Hassan and S. M. F. G. El-Rab, Int. J. Nanomed., 2020, 15, 1889–1901 CrossRef CAS PubMed.
- J. Joseph, K. Z. Khor, E. J. Moses, V. Lim, M. Y. Aziz and N. A. Samad, Int. J. Nanomed., 2021, 16, 3599–3612 CrossRef CAS PubMed.
- O. H. Abdelhafez, J. R. Fahim, R. R. El Masri, M. A. Salem, S. Y. Desoukey, S. Ahmed, M. S. Kamel, S. M. Pimentel-Elardo, J. R. Nodwell and U. R. Abdelmohsen, RSC Adv., 2021, 11, 23654–23663 RSC.
- M. Adnan, M. Patel, M. N. Reddy and E. Alshammari, Sci. Rep., 2018, 8 DOI:10.1038/s41598-018-20237-z.
- L. V. Hublikar, S. V. Ganachari, V. B. Patil, S. Nandi and A. Honnad, Prog. Biomater., 2023, 12, 155–169 CrossRef CAS PubMed.
- H. Khalili, S. A. S. Shandiz and F. Baghbani-Arani, J. Clust. Sci., 2017, 28, 1617–1636 CrossRef CAS.
- A. H. Shah, E. Manikandan, M. B. Ahamed, D. A. Mir and S. A. Mir, J. Lumin., 2014, 145, 944–950 CrossRef CAS.
- M. Rashidipour and R. Heydari, J. Nanostructure Chem., 2014, 4, 112 CrossRef.
- Z. A. Ratan, M. F. Haidere, M. Nurunnabi, S. M. Shahriar, A. J. S. Ahammad, Y. Y. Shim, M. J. T. Reaney and J. Y. Cho, Cancers, 2020, 12, 855 CrossRef CAS PubMed.
- M. Wypij, T. Jędrzejewski, J. Trzcińska-Wencel, M. Ostrowski, M. Rai and P. Golińska, Front. Microbiol., 2021, 12, 632505 CrossRef PubMed.
- K. C. Hembram, R. Kumar, L. Kandha, P. K. Parhi, C. N. Kundu and B. K. Bindhani, Artif. Cells, Nanomed., Biotechnol., 2018, 46, S38–S51 CrossRef PubMed.
- G. Gahlawat and A. R. Choudhury, RSC Adv., 2019, 9, 12944–12967 RSC.
- N. Z. Srećković, Z. P. Nedić, D. Liberti, D. M. Monti, N. R. Mihailović, J. S. K. Stanković, S. Dimitrijević and V. B. Mihailović, RSC Adv., 2021, 11, 35585–35599 RSC.
- J. Jeevanandam, S. F. Kiew, S. Boakye-Ansah, S. Y. Lau, A. Barhoum, M. K. Danquah and J. Rodrigues, Nanoscale, 2022, 14, 2534–2571 RSC.
- A. A. Kajani, A.-K. Bordbar, S. H. Z. Esfahani, A. R. Khosropour and A. Razmjou, RSC Adv., 2014, 4, 61394–61403 RSC.
- A. V. V. V. R. Kiran, G. K. Kumari, P. T. Krishnamurthy and R. R. Khaydarov, Biomater. Sci., 2021, 9, 7667–7704 RSC.
- V. Soshnikova, Y. J. Kim, P. Singh, Y. Huo, J. Markus, S. Ahn, V. Castro-Aceituno, J. Kang, M. Chokkalingam, R. Mathiyalagan and D. C. Yang, Artif. Cells, Nanomed., Biotechnol., 2018, 46, 108–117 CrossRef CAS PubMed.
|
This journal is © The Royal Society of Chemistry 2023 |
Click here to see how this site uses Cookies. View our privacy policy here.