Flavin based supramolecular gel displaying multi-stimuli triggered sol–gel transition†
Received
9th May 2023
, Accepted 16th June 2023
First published on 16th June 2023
Abstract
Herein, we report the design and synthesis of an amphiphilic flavin analogue as a robust low molecular weight gelator involving minimal structural modification. Four flavin analogues were evaluated for their gelation capabilities and the flavin analogue with antipodal positioning of the carboxyl and octyl functionalities was found to be the most efficient gelator with the minimum gelation concentration being as low as 0.03 M. A wide range of solvents were used for gelation studies suggesting its widespread applicability. Morphological, photophysical and rheological characterization studies were performed to fully characterize the nature of the gel. Interestingly, reversible multiple stimuli responsive sol–gel transition was observed with changing pH and redox activity, while metal screening showed specific transition in the presence of ferric ions. The gel was able to differentiate between ferric and ferrous species with well-defined sol–gel transition. The current results potentially offer a redox-active flavin-based material as a low molecular weight gelator for the development of next-generation materials.
1 Introduction
Continued interest in designing and developing novel soft materials with the capability of inducing controlled and reversible response in the presence of external stimuli has led to it being the most sought-after research area.1 While the earlier design relies on long polymeric supports to form supramolecular gels, recent investigation pertaining to low molecular weight gelators (LMWGs) has shown advantages in terms of versatility and controllability over nanoscale supramolecular gel formation and hence potential applicability. Various models have been reported to display photophysical or morphological responsiveness involving dynamic sol–gel transition in response to various stimuli such as light,2 temperature,3 electrical potential,4 magnetic field,5 reductive or oxidative environment,6 pH7 or ions.8 Such variations in the design model and stimuli response are crucial for a range of applications such as chemical sensing,9 display materials,10 biomedical technologies,11 drug delivery,12etc.
In this context, a majority of these low molecular weight gelators (LMWGs) incorporate responsive sites/functionalities such as photoactive, electroactive, or chemically reactive groups within their structural frameworks.13 Incorporation of a redox active scaffold offers simultaneous alterations in charges as well as spin states of an electroactive entity, thereby offering multiple modes for stimuli response including optical, magnetic, and mechanical features.14 In general, molecular scaffolds containing ferrocene15 or tetrathiafulvalene (TTF)4,16 have been frequently used. Surprisingly, the natural redox cofactor viz. flavin moiety, displaying excellent redox activity along with the luminescent nature in various biological phenomena,17 has scarcely been used as a low molecular weight gelator. Previous reports on flavin-based supramolecular gels constitute either an N3 diphenylalanine-conjugated flavopeptide analogue18 or an octadecanoyl esterified riboflavin analogue19 and these were investigated for enhanced catalytic activity involving immobilized flavin onto a gel matrix.
As we were interested in modulating the functional behavior of flavin via subtle chemical modification around the core structural framework,20 we decided to design a novel electroactive gel scaffold based on an isoalloxazine core system. The intrinsic redox feature associated with this isoalloxazine ring17 along with the ease of structural functionalization around the flavin core21 makes this tricyclic isoalloxazine moiety a strong candidate for novel soft material applications. A simplistic molecular model resulting in supramolecular gelation would possibly be built by incorporating an amphiphilic character into the core structural entities.22 Incorporating a polar and/or apolar functionality at the chosen position within the molecular framework (Fig. 1b) would possibly lead to variation in the gelation capability as well as the thermal and mechanical properties of such gel networks.23
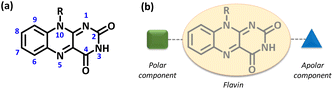 |
| Fig. 1 (a) Numbering scheme for the isoalloxazine moiety. (b) Representative illustration of the necessary structural components for the design of the amphiphilic flavin entity. | |
In order to probe a correlation between the spatial structure and the gelation capacity for such moieties, we decided to synthesize four flavin analogues as shown in Fig. 2, where the polar/apolar functionality was covalently linked at the C7 or N3 position of the isoalloxazine ring.
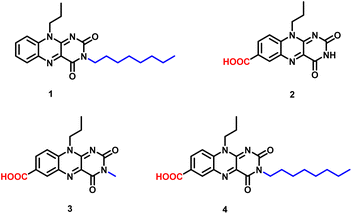 |
| Fig. 2 Chemical structures of flavin analogues investigated for supramolecular gelation. | |
Herein, we report the simple, yet elegant design built around the isoalloxazine core for the formation of a flavin-based supramolecular gel. Systematic inclusion of the polar/apolar functionality around the isoalloxazine core structure was evaluated for their gelation capability to identify the minimalistic chemical modification needed to achieve the gelation behavior. Complete characterization of the flavin analogue displaying better gelation was performed via photophysical, morphological and rheological techniques. Furthermore, stimuli response was evaluated for redox transition of Fe3+/Fe2+ and pH by reversible gel–sol transition. However, the unpredictable nature associated with the construction of these gel networks from these small building blocks barred researchers from rationalizing the design strategy for LMWGs. Hence, understanding the correlation between the spatial structure and the gelation capacity for such moieties is still a challenging task. Preliminary gelation studies suggest that compound 4 results in gel formation with minimal gelation concentration requirement (Fig. 3).
 |
| Fig. 3 Preliminary gelation experiments in DMSO. In (a) and (b), no gel formation was observed with 1 and 2 even at 0.06 M concentration. (c and d) Representation of the formation of gel with 3 and 4 at 0.06 M and 0.03 M concentrations. | |
2 Experimental section
2.1 Materials
Chemicals and reagents used were purchased from commercial sources including Sigma Aldrich, SRL Chemicals Pvt. Ltd. and Avra Synthesis Pvt. Ltd., and were used without purification. The products obtained were characterized by NMR and HRMS (Scheme S1 and Fig. S1–S18 in the ESI†).
2.2 Characterization
The synthetic details used for the synthesis of the flavin analogues are given in the ESI.† Nuclear magnetic resonance spectra were collected using a Bruker DRX-400 spectrometer. 1H NMR spectra were collected at 400 MHz and 13C NMR spectra were collected at 100 MHz. Resonances are reported in parts per million (ppm) and coupling constants, J, are reported in hertz (Hz). High-resolution mass spectra were obtained by the Electron Spray Ionization method (ESI) using Agilent QTOF 6538. UV-Visible spectral measurements were recorded using a JASCO V730 spectrophotometer and fluorescence measurements were performed using a JASCO FP-8300 spectrofluorometer at 25 °C.
Rheological studies were performed using a Kinexus Pro+ Rheometer from Malvern Panalytical. 0.4 mL of the gel was placed on a sample plate, and the test temperature was adjusted to 25 °C while data acquisition was performed. For optical microscopy studies, the xerogel was placed on a glass substrate and visualized under an Olympus microscope. Scanning electron microscopy investigations for the xerogel were carried out using a JEOL microscope (JIB-4700F) at an accelerating voltage of 3 kV.
2.3 Gelation experiments
The gelation experiments were carried out in a 3 mL glass vial, in which the weighted compound was added to the appropriate solvent. The vial was heated in an oil bath until the solid material dissolved completely. The resulting solutions were slowly cooled to room temperature and sonicated until gel formation was confirmed by an inversion test. Microscopy investigations and solid fluorescence measurements were done with the xerogel (prepared by drying the gel material under reduced pressure for 24 hours). For the stimuli response experiments, the corresponding metal salts were used – NaNO3, KNO3, Mg(NO3)2·6H2O, Ca(NO3)2·4H2O, Sr(NO3)2, Ba(NO3)2, Cr(NO3)3·9H2O, FeCl2·4H2O, Fe(NO3)3·9H2O, Co(NO3)2·6H2O, Ni(NO3)2·6H2O, Cu(NO3)2·3H2O, Zn(NO3)2·6H2O, Cd(NO3)2·4H2O and AlCl3.
3 Results and discussion
To understand the structural requirement around the tricyclic flavin core for gel formation, four different flavin analogues were chosen and preliminary studies were performed for understanding the gelation behavior. The amphiphilic character within the flavin core structure was induced by incorporating polar (carboxylic) and non-polar (alkyl) functionalities at the antipodal position with respect to the tricyclic isoalloxazine core. The antipodal positioning of the functional group was envisioned to maximize the gelation efficiency with minimum molecular complexity.24 Taking this consideration into account, structural functionalization at the phenylic and N3 imide positions was done with carboxylic and alkyl groups (Fig. 1). Incorporation of a pH-sensitive carboxylic group along with the redox active nature of the flavin core could possibly offer a potentially multiple stimuli responsive gel scaffold.
A preliminary experiment for investigating the gelation capability of the synthesized flavin analogues (1–4) was performed using DMSO as the common solvent. Monofunctionalized (N3-octyl or C7-carboxylic) flavin analogues (1 and 2) did not show any gelation capability even at 0.06 M concentrations (Fig. 3a and b). This observation is suggestive of the insufficient amphiphilic nature of the flavin analogues, which is possibly required for gel formation.
Interestingly, bi-functionalized flavin analogues (3) involving both the carboxyl and methyl functionalities resulted in successful gelation of DMSO, as shown in the inversion test (Fig. 3c). Notably, flavin analogue 3 also displayed the minimal structural requirement around the flavin core for inducing supramolecular gelation. Further improvement in the gelation capability and the structural strength was achieved by increasing the length of the alkyl group (replacing the methyl group with octyl at the N3 position of flavin).25 As observed, the minimal gelation concentration (MGC) required for model 3 was found to be 0.06 M, while for model 4, it was 0.03 M. The fluorescent nature of the flavin core remains intact as observed by placing the gel scaffolds 3 and 4 under UV light (366 nm), and both the gels exhibit yellowish-green fluorescence emission (Fig. 3c and 3d).
Considering the better gelation capability of 4 among the synthesized flavin analogues (1–4), we decided to explore the gelation behavior of 4 in a wide range of solvents. As shown in Table 1, both polar and non-polar solvents were investigated for gelation studies. A wide range of polar solvents, including a range of alcoholic solvents along with DMSO and MeCN, were found to successfully result in gel formation. This observation is possibly suggestive of the crucial role played by the polar functional groups in stabilizing the gel scaffold via hydrogen bonding interactions. On the other hand, gelation was not observed in an aqueous medium and non-polar solvents such as hexane, toluene, benzene, diethyl ether and chlorinated solvents, possibly due to the insolubility of the flavin analogue in the abovementioned solvents. Interestingly, analogue 4 was also found to form the gel in an ionic liquid (Bmim-PF6) and propylene carbonate, possibly showing its potential application in a wider range of chemical environments.26 The gel transition temperature and the minimum gelation concentration were determined for all the solvents in which gelation was observed. While the highest gelation temperature was observed in ethylene glycol, the lowest MGC was observed in propylene carbonate, possibly suggesting the role of medium viscosity in strengthening the gel structure as well.
Table 1 Solvent gelation studies of 4 in different solvents
Solvent |
State |
T
gel (°C) |
(w/w%, M) |
G = gel formation at 25 °C; S = solution at 25 °C (S); P = precipitation at 25 °C, although a clear solution was observed in the heated sample; I = mainly insoluble at 25 °C and in the heated state, stimuli for gelation: heating followed by cooling or sonication. |
DMSO |
G |
64–66 |
1.09, 0.029 |
Acetonitrile (ACN) |
G |
104–106 |
1.63, 0.03 |
Methanol |
G |
82–88 |
3, 0.048 |
Ethanol |
G |
80–84 |
2.54, 0.068 |
2-Propanol |
G |
88–96 |
3.9, 0.105 |
1-Butanol |
G |
92–94 |
3.12, 0.083 |
2,2,2-Trifluoroethanol |
G |
92–94 |
1.29, 0.0496 |
1,2-Ethanediol |
G |
134–136 |
1.11, 0.03 |
Ethyl cellosolve |
G |
74–78 |
2.4, 0.064 |
1,4-Butanediol |
G |
112–114 |
1.74, 0.0426 |
Benzylalcohol |
G |
60–62 |
6.1, 0.162 |
THF |
G |
62–66 |
3.75, 0.1 |
Dioxane |
G |
68–72 |
3, 0.0787 |
Nitrobenzene |
G |
116–118 |
1.69, 0.049 |
Anisole |
G |
118–120 |
1.66, 0.04 |
1,2-Dimethoxyethane |
G |
68–70 |
2.03, 0.0426 |
Bmim-PF6 |
G |
124–128 |
0.978, 0.047 |
Propylene carbonate |
G |
114–116 |
0.36, 0.0105 |
DMF, pyridine |
S |
— |
— |
Water, acetone, ethylacetate, CHCl3, DCE, CCl4, diethylether, benzene, toluene, n-hexane, and glycerol |
I |
— |
— |
Preliminary absorption and emission studies of the flavin analogues (1–4) were performed to correlate the photophysical behavior with the structural variation. The absorption spectra recorded in DMSO, as shown in Fig. 4a, show the presence of three peaks between the 250 nm and 500 nm wavelength range in all the flavins 1–4, which can be attributed to the characteristic absorption band for the isoalloxazine ring originating from π–π* transitions.27 Interestingly, a subtle shift in both shorter and longer wavelength bands by approximately 13 nm and 6 nm, respectively, was observed for analogues 2–4, when compared with that of 1. A decrease in the intensity of the absorption band at around 331 nm was also observed for analogues 2–4 as compared to 1. This observation can possibly be attributed to the electron-withdrawing nature of the covalently linked carboxyl group.28 As expected, the effect of the covalent carboxyl-functionalization on the photophysical nature of flavin was also evident from the emission spectra. While similar emission spectra were observed for all four flavin analogues with the emission maxima observed at around 526 nm, significant emission quenching was observed for 2–4, when compared with 1 (Fig. 4b).
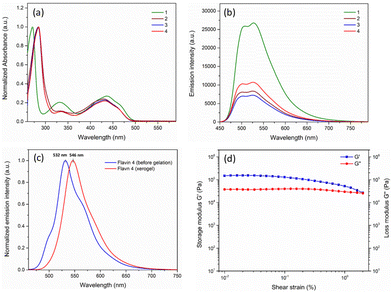 |
| Fig. 4 (a) Solution phase absorption and (b) emission spectra of flavin derivatives 1–4. (c) Representative solid fluorescence spectra for flavin 4: before and after gelation (xerogel). (d) Representation of the rheological behavior (strain sweep) of gel 4 prepared in DMSO. | |
Furthermore, the solid-state emission spectra were recorded before and after gel formation to investigate the effect of gelation on the photophysical behavior for analogue 4 (Fig. 4c). A subtle shift in the emission maxima (∼6 nm) was also observed when compared to the solution phase (526 nm). Notably, a significant bathochromic shift (∼14 nm) was observed for xerogel 4 (emission maxima at 546 nm) as compared to the solid-state emission before gelation (emission maxima at 532 nm). This observation expectedly supports the significant structural rearrangement during the formation of the gel.
To explore the mechanical properties of the modified flavin-based gel, rheological measurement was conducted using 4. As shown in Fig. 4d, a strain sweep experiment was performed to investigate the effect of strain on the storage (G′) and loss moduli (G′′) associated with the gel formed using analogue 4. It was observed that below the critical strain region, the storage modulus and loss modulus were found to be independent of the stress applied. The deformation was found to be negligible, suggesting that the gelatinous structure remains completely intact. Also, the storage modulus (G′) was found to be greater than the loss modulus, which is indicative of the elastic nature of the gel formed.29
Morphological investigation was performed by optical microscopy and scanning electron microscopy to gain insight into the morphological features of the gel formed using analogue 4. A preliminary analysis of gel 4 in DMSO using an optical micrograph, as shown in Fig. 5a, suggest the possible formation of self-assembled fibrillar structures.30 Further studies using the SEM technique reveal the presence of fibre-like morphology. A closer inspection of the fibrous structure shows the entanglement of the fibrous aggregates in a bundle-like arrangement as shown in Fig. 5c and 5d. Notably, these fibrous structures were found to be of different dimensions ranging from a couple of micrometres to tens of micrometres in length. The possible interplay of various non-covalent interactions such as H-bonding, π–π stacking, solvophobic forces and van der Waals interactions might be influencing the overall morphology of the gel scaffold.31 A possible mode of the molecular arrangement leading to gelation might involve strong hydrogen bonding interaction between the carboxylic functionalities in a dimeric arrangement, while the non-polar alkyl groups possibly projected outside interacting with each other via van der Waals forces. The tricyclic isoalloxazine ring might stabilize the overall morphology via stacking interaction between the two adjacent flavin moieties. The propagation of such a supramolecular arrangement might lead to fibrous microstructure formation.
 |
| Fig. 5 (a) Optical microscopy image of xerogel 4 formed in DMSO, while (b) shows an optical image under polarizing light. (c and d) Representation of the morphological features using the SEM image of the xerogel. | |
3.1 Reversible gel–sol transition by pH variation
Being interested in generating multi-stimuli responsive gel, we decided to investigate the influence of different stimuli on the gel structure. As mentioned earlier, the presence of the carboxylic functionality in the core structure offers strong hydrogen bonding interactions, resulting in the formation of a gelatinous scaffold. In this context, changing the chemical environment might disrupt the prevailing hydrogen bonding interaction and hence the gel scaffold. Investigating the pH response of the gel (4) prepared in DMSO (0.05 M) via addition of a base such as sodium hydroxide, sodium carbonate or aqueous ammonia resulted in a gradual change of the gel state to the solution phase (Fig. 6). Interestingly, the subsequent addition of hydrochloric acid was shown to re-insatiate the gel form. However, addition of a weaker acid like acetic acid (up to 10 equivalents) did not affect the gel state. The possibility of protonation/deprotonation of the carboxylic group of the flavin analogue leading to the change in the hydrogen bonding interaction might account for the observed reversible sol–gel transition.
 |
| Fig. 6 Reversible tuning of sol–gel phase transition via addition of a base and an acid to gel 4 in DMSO (0.05 M). | |
3.2 Reversible gel–sol transition by redox variation
Being interested in investigating the metal–flavin interaction for modulating the functional behaviour,20b–e we have further evaluated the effect of metal ions on the gel. A preliminary study with a series of metal ions, as shown in Fig. 7, reveals the high selectivity for the ferric ion, which shows the transition from the gel phase to the solution phase, while no effect was observed in cases where other metal ions were used including the ferrous (Fe2+) ion. The minimal effect of other trivalent ions such as Cr3+ and Al3+ on the gel state suggests a stronger interaction with the ferric ion. Furthermore, we decided to investigate the gel response towards chemical oxidation–reduction transformation involving Fe3+/Fe2+. As shown in Fig. 8, the addition of the Fe3+ salt (1 eq.) to gel 4 formed in methanol resulted in phase transition to sol, possibly suggesting the interaction of Fe3+ with the carboxyl pendant of 4, thereby disrupting the gel network. Interestingly, the subsequent reduction of the ferric ion to the ferrous ion by addition of 2 equivalents of ascorbic acid using a previously reported method32 resulted in gel formation again. The reversible sol–gel transition involving Fe3+/Fe2+ could be attributed to the lack of interaction of relatively softer ferrous ions with harder carboxyl groups.33 This observation was further supported by the addition of stronger metal chelating agents such as tetrasodium EDTA or trisodium citrate salts, which reveal the reversible conversion of the sol phase to the gel phase and vice versa.
 |
| Fig. 7 (a) Inversion test of gel 4 prepared in methanol (0.05 M) with addition of 1 equivalent of metal nitrates (chloride salts were used for Fe2+ and Al3+) and (b) shows the observation under UV light (366 nm). The sequence from left to right represents the added metal ions: (i) no metal, (ii) Na+, (iii) K+, (iv) Mg2+, (v) Ca2+, (vi) Sr2+, (vii) Ba2+, (viii) Cr3+, (ix) Fe2+, (x) Fe3+, (xi) Co2+, (xii) Ni2+, (xiii) Cu2+, (xiv) Zn2+, (xv) Cd2+, and (xvi) Al3+. | |
 |
| Fig. 8 Reversible tuning of gel formation of 4 by chemical oxidation and reduction in methanol. | |
4 Conclusions
In conclusion, we have successfully designed and synthesized a simple low molecular weight gelator based on an amphiphilic flavin analogue. A systematic approach is followed to maximize the gelation efficiency with minimal structural modification. An antipodal positioning of the carboxylic and alkyl functionalities around the flavin core was found to be suitable for the generation of the gel scaffold. The gelation behaviour was evaluated in various solvent media to ascertain its applicability in different chemical environments. Molecular design with the minimum gelation concentration (analogue 4) was further investigated for morphological features, mechanical strength and luminescent behaviour. Screening against various metal ions suggested high selectivity towards ferric ions. Interestingly, a reversible sol to gel transition was also observed with a change in ferrous to ferric ions. The gel was found to be responsive toward multiple chemical stimuli such as pH and redox conversion. This study demonstrates the easy design and applicability of a flavin-based low molecular weight gelator towards functional soft materials.
Conflicts of interest
There are no conflicts to declare.
Acknowledgements
MSSVM thanks MHRD for the scholarship. AKM thanks the Science and Engineering Research Board (SERB) India for the research funding (CRG/2022/006706). Both the authors thank the Indian Institute of Technology Hyderabad for providing the necessary infrastructure and instrumentation facilities.
Notes and references
-
(a) S. Panja and D. J. Adams, Chem. Soc. Rev., 2021, 50, 5165–5200 RSC;
(b) Z. Sun, Q. Huang, T. He, Z. Li, Y. Zhang and L. Yi, ChemPhysChem, 2014, 15, 2421–2430 CrossRef CAS PubMed.
-
(a) H. Yan, Y. Qiu, J. Wang, Q. Jiang, H. Wang, Y. Liao and X. Xie, Langmuir, 2020, 36, 7408–7417 CrossRef CAS PubMed;
(b) Z. Li, G. Wang, Y. Wang and H. Li, Angew. Chem., Int. Ed., 2018, 57, 2194–2198 CrossRef CAS PubMed;
(c) H. Yamaguchi, Y. Kobayashi, R. Kobayashi, Y. Takashima, A. Hashidzume and A. Harada, Nat. Commun., 2012, 3, 1617–1621 Search PubMed;
(d) M. J. Clemente, R. M. Tejedor, P. Romero, J. Fitremann and L. Oriol, New J. Chem., 2015, 39, 4009–4019 RSC.
- S. Xian and M. J. Webber, J. Mater. Chem. B, 2020, 8, 9197–9211 RSC.
- C. Wang, Q. Chen, F. Sun, D. Zhang, G. Zhang, Y. Huang, R. Zhao and D. Zhu, J. Am. Chem. Soc., 2010, 132, 3092–3096 CrossRef CAS PubMed.
- K. Lalitha, Y. S. Prasad, V. Sridharana, C. U. Maheswaria, G. John and S. Nagarajan, RSC Adv., 2015, 5, 77589–77594 RSC.
-
(a) S. Kawano, N. Fujita and S. Shinkai, J. Am. Chem. Soc., 2004, 126(28), 8592–8593 CrossRef CAS PubMed;
(b) F. Peng, G. Li, X. Liu, S. Wu and Z. Tong, J. Am. Chem. Soc., 2008, 130(48), 16166–16167 CrossRef CAS PubMed.
-
(a) W. Deng and D. H. Thompson, Soft Matter, 2010, 6, 1884–1887 RSC;
(b) G. Ghosh, R. Barman, J. Sarkar and S. Ghosh, J. Phys. Chem. B, 2019, 123, 5909–5915 CrossRef CAS PubMed;
(c) M. Externbrink, S. Riebe, C. Schmuck and J. Voskuhl, Soft Matter, 2018, 14, 6166–6170 RSC.
- L. Li, R. Sun, R. Zheng and Y. Huang, Mater. Des., 2021, 205, 109759 CrossRef CAS.
-
(a) S. Panja, A. Panjab and K. Ghosh, Mater. Chem. Front., 2021, 5, 584–602 RSC;
(b) X. Cao, A. Gao, J. Hou and T. Yi, Coord. Chem. Rev., 2021, 434, 213792 CrossRef CAS;
(c) Y. Feng, N. Jiang, D. Zhu, Z. Su and M. R. Bryce, J. Mater. Chem. C, 2020, 8, 11540–11545 RSC.
- Q. Lin, B. Sun, Q.-P. Yang, Y.-P. Fu, X. Zhu, Y.-M. Zhang and T.-B. Wei, Chem. Commun., 2014, 50, 10669–10671 RSC.
-
(a) D. Bairagi, P. Biswas, K. Basu, S. Hazra, D. Hermida-Merino, D. K. Sinha, I. W. Hamley and A. Banerjee, ACS Appl. Bio Mater., 2019, 2, 5235–5244 CrossRef CAS PubMed;
(b) X. Dou, N. Mehwish, C. Zhao, J. Liu, C. Xing and C. Feng, Acc. Chem. Res., 2020, 53, 852–862 CrossRef CAS PubMed.
- Y. Chen, X.-H. Pang and C.-M. Dong, Adv. Funct. Mater., 2010, 20, 579–586 CrossRef CAS.
- X. Sui, X. Feng, M. A. Hempenius and G. J. Vancso, J. Mater. Chem. B, 2013, 1, 1658–1672 RSC.
- T. Fukino, H. Yamagishi and T. Aida, Adv. Mater., 2017, 29, 1603888 CrossRef PubMed.
-
(a) L. Peng, A. Feng, M. Huo and J. Yuan, Chem. Commun., 2014, 50, 13005–13014 RSC;
(b) X. Liu, L. Zhao, F. Liu, D. Astruc and H. Gu, Coord. Chem. Rev., 2020, 419, 213406 CrossRef CAS.
-
(a) Y. Liu, N. Zheng, T. Chen, L. Jina and B. Yin, Org. Biomol. Chem., 2014, 12, 6927–6936 RSC;
(b) X. Yang, G. Zhanga and D. Zhang, J. Mater. Chem., 2012, 22, 38–50 RSC.
-
(a) M. W. Fraaije and A. Mattevi, Trends Biochem. Sci., 2000, 25, 126–132 CrossRef CAS PubMed;
(b) C. T. Walsh, Acc. Chem. Res., 1980, 13, 148–155 CrossRef CAS;
(c) C. T. Walsh, Acc. Chem. Res., 1986, 19, 216–221 CrossRef CAS;
(d) M. J. Clarke, Comments Inorg. Chem., 1984, 3, 133–151 CrossRef CAS;
(e) M. J. Clarke, Rev. Inorg. Chem., 1980, 2, 27–52 CAS;
(f) T. C. Bruice, Acc. Chem. Res., 1980, 13, 256–262 CrossRef CAS.
- M. Cariello, B. Dietrich, L. Thomson, V. Gauci, A. Boyer, S. Sproules, G. Cooke, A. Seddon and D. J. Adams, Chem. – Eur. J., 2022, 28, e202201725 CrossRef CAS PubMed.
- S. Kawamorita, M. Fujiki, Z. Li, T. Kitagawa, Y. Imada and T. Naota, ChemCatChem, 2019, 11, 878–884 CrossRef CAS.
-
(a) M. S. S. V. Mouli and A. K. Mishra, J. Chem. Sci., 2022, 134, 59 CrossRef CAS;
(b) M. S. S. V. Mouli and A. K. Mishra, RSC Adv., 2022, 12, 3990–3995 RSC;
(c) M. S. S. V. Mouli and A. K. Mishra, CrystEngComm, 2022, 24, 2221 RSC;
(d) M. S. S. V. Mouli and A. K. Mishra, ChemistrySelect, 2022, 7, e202202126 Search PubMed;
(e) M. S. S. V. Mouli, S. Katyal and A. K. Mishra, Synlett, 2023, 829–834 CAS;
(f) M. S. S. V. Mouli, H. G. Agrawal, A. Tamrakar, S. R. Tripathy, M. D. Pandey and A. K. Mishra, Luminescence, 2022 DOI:10.1002/bio.4339;
(g) M. S. S. V. Mouli and A. K. Mishra, Dyes Pigm., 2023, 212, 111148 CrossRef CAS;
(h) M. S. S. V. Mouli, D. Mondal, K. Kumari, S. K. Singh and A. K. Mishra, Org. Biomol. Chem., 2023, 21, 3311–3316 RSC.
- A. Kormanyos, M. S. Hossain, G. Ghadimkhani, J. J. Johnson, C. Janaky, N. R. de Tacconi, F. W. Foss, Y. Paz and K. Rajeshwar, Chem. – Eur. J., 2016, 22, 9209–9217 CrossRef CAS PubMed.
-
(a) J. T. van Herpt, J. Areephong, M. C. A. Stuart, W. R. Browne and B. L. Feringa, Chem. – Eur. J., 2014, 20, 1737–1742 CrossRef CAS PubMed;
(b) Q. Jin, L. Zhang, X. Zhu, P. Duan and M. Liu, Chem. – Eur. J., 2012, 18, 4916–4922 CrossRef CAS PubMed;
(c) T. Kar, S. Mukherjee and P. K. Das, New J. Chem., 2014, 38, 1158–1167 RSC.
- N. Mohmeyer and H.-W. Schmidt, A New Class of Low-Molecular-Weight Amphiphilic Gelators, Chem. – Eur. J., 2005, 11, 863–872 CrossRef CAS PubMed.
- T. Kar, S. Debnath, D. Das, A. Shome and P. K. Das, Langmuir, 2009, 25, 8639–8648 CrossRef CAS PubMed.
- H.-K. Yang, H. Zhao, P.-R. Yang and C.-H. Huang, Colloids Surf., A, 2017, 535, 242–250 CrossRef CAS.
-
(a) G. Sinawang, Y. Kobayashi, Y. Zheng, Y. Takashima, A. Harada and H. Yamaguchi, Macromolecules, 2019, 52, 2932–2938 CrossRef CAS;
(b) Y. Ishioka, N. Minakuchi, M. Mizuhata and T. Maruyama, Soft Matter, 2014, 10, 965–971 RSC;
(c) F. Placin, J.-P. Desvergne and J.-C. Lassègues, Chem. Mater., 2001, 13, 117–121 CrossRef CAS.
-
(a) N. Dozova, F. Lacombat, C. Bou-Nader, D. Hamdane and P. Plaza, Phys. Chem. Chem. Phys., 2019, 21, 8743–8756 RSC;
(b) J. N. Iuliano, C. R. Hall, D. Green, G. A. Jones, A. Lukacs, B. Illarionov, A. Bacher, M. Fischer, J. B. French, P. J. Tonge and S. R. Meech, J. Phys. Chem. B, 2020, 124, 7152–7165 CrossRef CAS PubMed;
(c) J. Brazard, A. Usman, F. Lacombat, C. Ley, M. M. Martin and P. Plaza, J. Phys. Chem. A, 2011, 115, 3251–3262 CrossRef CAS PubMed.
-
(a) T. C. Bruice, T. W. Chan, T. P. Taulane, I. Yokoe, D. L. Elliot, R. F. Williams and M. Novak, J. Am. Chem. Soc., 1977, 99, 6713–6720 CrossRef CAS PubMed;
(b) D. M. Răsădean, T. Machida, K. Sada, C. R. Pudney and G. D. Pantoş, Tetrahedron, 2021, 82, 131925 CrossRef.
- A. Dawn and H. Kumari, Chem. – Eur. J., 2018, 24, 762–776 CrossRef CAS PubMed.
- V. A. Mallia and R. G. Weiss, J. Phys. Org. Chem., 2014, 27, 310–315 CrossRef CAS.
- N. M. Sangeetha and U. Maitra, Chem. Soc. Rev., 2005, 34, 821–836 RSC.
-
(a) H. Lee, J. Park, S. Y. Han, S. Han, W. Youn, H. Choi, G. Yun and I. S. Choi, Chem. Commun., 2020, 56, 13748–13751 RSC;
(b) L. Guo, Y. Liu, R. Kong, G. Chen, Z. Liu, F. Qu, L. Xia and W. Tan, Anal. Chem., 2019, 91, 12453–12460 CrossRef CAS PubMed.
- F. Peng, G. Li, X. Liu, S. Wu and Z. Tong, J. Am. Chem. Soc., 2008, 130, 16166–16167 CrossRef CAS PubMed.
|
This journal is © The Royal Society of Chemistry 2023 |
Click here to see how this site uses Cookies. View our privacy policy here.