DOI:
10.1039/D2QI01873J
(Research Article)
Inorg. Chem. Front., 2023,
10, 305-315
A water-tuned reversible spin transition with the largest hysteresis loop in 3D Hofmann frameworks pillared by flexible ligands†
Received
30th August 2022
, Accepted 7th November 2022
First published on 7th November 2022
Abstract
Using rigid linear ligands as pillars for constructing 3D Hofmann-type frameworks is an important strategy to synthesize spin-crossover (SCO) materials with a large hysteresis loop. However, application of flexible pillars for building 3D Hofmann SCO networks remains a big challenge. Herein, by judiciously choosing a known ligand, N,N′-bis(4-picolinoyl)hydrazine (bph) as a flexible pillar, two novel isostructural 3D Hofmann SCO frameworks [Fe(bph)M(CN)4]·4.5H2O (1·4.5H2O-c, M = Pt2+; 2·4.5H2O-c, M = Pd2+; c = crystal) have been successfully synthesized. Both 1·4.5H2O-c and 2·4.5H2O-c exhibited an abrupt spin transition with a hysteresis loop of 60 and 30 K, respectively, representing the largest hysteresis width found for the 3D Hofmann SCO frameworks built from flexible pillars until now. In contrast, the dehydrated complexes 1 and 2 obtained from the dehydration of 1·4.5H2O-c and 2·4.5H2O-c, respectively, only showed a gradual spin transition without any hysteresis loop. Notably, both 1·and 2 can be transformed again into powdery 1·4.5H2O-p and 2·4.5H2O-p (p = powder) on exposure to water. However, the rehydrated 1·4.5H2O-p and 2·4.5H2O-p merely indicated incomplete two-step SCO behaviors with small hysteresis loops but higher transition temperatures compared with 1·4.5H2O-c and 2·4.5H2O-c. X-ray crystallographic studies at 296 and 100 K revealed that the rare large hysteresis loops observed in 1·4.5H2O-c and 2·4.5H2O-c may be ascribed to the strong cooperativity in the SCO process owing to the rich hydrogen bond interactions between the flexible bph ligands and lattice water molecules.
1. Introduction
Spin-crossover (SCO) materials are appealing bistable molecules with a reversible switch between the high-spin (HS) and low-spin (LS) states of the 3d4–3d7 transition metal complexes stimulated by temperature, pressure, or light irradiation.1–4 Among the complexes, iron(II) SCO materials are one of the most notable systems.5–7 Until now, diverse spin-transition modes (gradual, abrupt, hysteretic, multi-step and incomplete) have been found in iron(II) SCO complexes,6 and the transition modes can be finely tuned by judicious selection and delicate modification of ligands.8,9 A typical and interesting example is porous 3D Hofmann-type SCO frameworks consisting of 2D cyanide-bridged Fe2+–M2+/M+ (M = Ni2+, Pd2+, Pt2+, Ag+, Au+) layers pillared by all kinds of linear ligands.10–36 Simultaneously, the accessible voids in the 3D Hofmann frameworks can also encapsulate different guest molecules which will provide a huge opportunity for investigating the host–guest interactions in order to explore guest-dependent SCO behavior. Therefore, guest-dependent 3D Hofmann SCO frameworks are actually a new generation of multifunctional materials involving SCO and porosity and have attracted much attention over the past two decades.10,36 For example, in 2001, Prof. Real et al. reported the first 3D Hofmann SCO frameworks [Fe(pz)M(CN)4]·nH2O (pz = pyrazine; M = Ni2+, Pt2+ (n = 2), Pd2+ (n = 2.5)) with a hysteresis loop of 20 and 33 K for [Fe(pz)Pt(CN)4]·2H2O and [Fe(pz)Pd(CN)4]·2.5H2O, respectively.10 Moreover, Real's subsequent research has demonstrated that the dehydrated complex [Fe(pz)Pt(CN)4] exhibits a complete SCO behavior at room temperature (RT) with a square-shaped hysteresis loop of 24 K.37 Using a long linear ligand bis(4-pyridyl)acetylene (bpac) as a pillar, a small hysteresis loop of 21 and 3 K, respectively, has been found in two 3D Hofmann SCO frameworks [Fe(bpac)M(CN)4]·0.5bpac·H2O (M = Pt2+ and Pd2+), while a larger hysteresis loop of 49 and 32 K, respectively, was observed in the dehydrated SCO complex [Fe(bpac)M(CN)4]·0.5bpac (M = Pt2+ and Pd2+).12 However, if a longer linear ligand than bpac, 1,4-bis(4-pyridylethynyl)benzene (bpeben), is applied as the pillar, no hysteresis loop can be found in either of the resulting 3D Hofmann SCO frameworks [Fe(bpeben)M(CN)4]·0.5bpeben·nH2O (M = Ni2+, Pd2+, Pt2+; n = 1.5 or 2) or the corresponding dehydrated SCO complexes.14 Consequently, it is still difficult to explain the role of the size of the linear pillars in terms of the spin-transition behaviors in 3D Hofmann SCO frameworks. In addition, the relationships between the spin-transition behaviors and host–guest interactions in porous 3D Hofmann SCO frameworks remain unclear.
To date, most of the pillars used in 3D Hofmann SCO frameworks with a six-coordinate octahedral [FeN6] configuration are rigid bidentate linkers.10–28 Recently, a few flexible ligands have been adopted as linear pillars for constructing 3D Hofmann SCO frameworks.29–36 These flexible pillars include di(4-pyridylthio)methane (dpsme),29 1,2-di(4-pyridyl)ethane (dpa),30 1,4-bis(4-pyridylmethyl)piperazine (bpmp),31 di(4-pyridyl)selenide (dpSe),32N-(4-pyridyl)isonicotinamide (pina),33 1,3-di(4-pyridyl)urea (dpyu),34 1,2-dibromo-1,2-di(4-pyridyl)ethane (dbdpe)35 and N,N′-di(4-pyridyl)oxalamide (dpo) (Scheme 1).36 Particularly interesting is that by employing a flexible dpo ligand as a pillar, Prof. Tong reported the guest-dependent four-step spin-transition behaviors in two 3D Hofmann SCO frameworks, [Fe(dpo){Ag(CN)2}2]·3DMF and [Fe(dpo){Ag(CN)2}2]·0.5MeCN·2DEF (DMF = N,N′-dimethylformamide; DEF = N,N′-diethylformamide).36 Inspired by Prof. Tong's pioneering work, in this paper, we selected a known compound, N,N′-bis(4-picolinoyl)hydrazine (bph), which is an isomer of the dpo ligand, as the flexible pillar for building 3D Hofmann SCO frameworks because the bph ligand can offer the following advantages: (1) it is a suitable length as a linear linker (11.5 Å) for preparing porous networks; (2) the double amide (O
C–NH) groups in the ligand can act both as donors and acceptors of hydrogen bonding; (3) strong host–guest interactions might occur in the 3D Hofmann SCO frameworks pillared by the bph ligand. Surprisingly, as an isomer of the dpo ligand, this simple bph ligand has never been applied for the construction of 3D Hofmann SCO frameworks so far, although some bph-based complexes with Cd2+, Cu2+ and Ca2+ ions have been reported.38,39
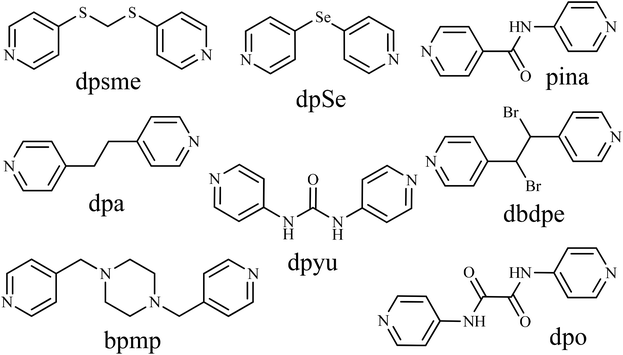 |
| Scheme 1 The flexible pillars used for 3D Hofmann SCO frameworks. | |
Herein, two novel isostructural 3D Hofmann SCO frameworks: [Fe(bph)M(CN)4]·4.5H2O (1·4.5H2O-c, M = Pt2+; 2·4.5H2O-c, M = Pd2+) have been successfully synthesized from the bph pillar (Scheme 2). Both 1·4.5H2O-c and 2·4.5H2O-c showed an abrupt one-step spin transition with a hysteresis loop width of 60 and 30 K, respectively, representing the largest hysteresis width found for 3D Hofmann SCO frameworks built from the flexible pillars until now. In contrast, the dehydrated complexes 1 and 2 obtained from the dehydration of 1·4.5H2O-c and 2·4.5H2O-c, respectively, only exhibited a gradual and incomplete spin transition without any hysteresis loop. Notably, both 1·and 2 can be re-transformed into powdery 1·4.5H2O-p and 2·4.5H2O-p on exposure to water. However, the rehydrated 1·4.5H2O-p and 2·4.5H2O-p merely indicated incomplete two-step SCO behaviors with small hysteresis loops but higher transition temperatures compared with the crystalline 1·4.5H2O-c and 2·4.5H2O-c. The rare large hysteresis loops observed in 1·4.5H2O-c and 2·4.5H2O-c may be ascribed to the strong cooperativity in the SCO process owing to the rich hydrogen bonds involving the flexible bph ligands and lattice water molecules from the host–host, host–guest and guest–guest interactions. Notably, these interactions have clearly been confirmed by variable temperature X-ray crystallographic studies.
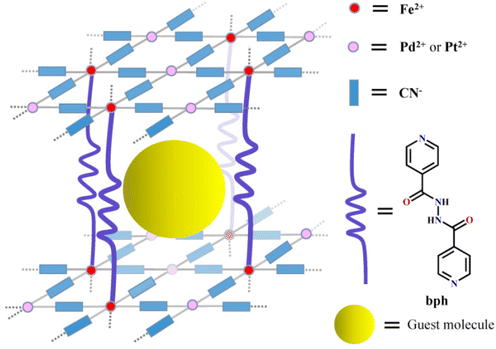 |
| Scheme 2 Design and construction of 3D Hofmann SCO frameworks pillared by flexible bph ligands. | |
2. Experimental
2.1. Materials and methods
All chemicals were of analytical grade and used without further purification. The bph ligand was prepared according to the literature method.40 Elemental analyses (C, H, N) were performed using a Thermo Finnigan Flash 1112A microanalyzer. FT-IR spectra were recorded in the range 4000–400 cm−1 using KBr discs on a Bruker Vector 22 FT-IR spectrophotometer. Thermogravimetric analyses (TGA) were performed on a NETZSCH STA 449C thermal analyser under a nitrogen atmosphere at a heating rate of 10 °C min−1. Powder X-ray diffraction (PXRD) data were collected on a Bruker D8 Advance diffractometer using Cu Kα radiation (λ = 1.5406 Å). Variable temperature (1.8–300 K) magnetic susceptibilities of the SCO complexes were measured on a Quantum Design MPMS3 SQUID magnetometer in the stable mode. Diamagnetic corrections were made using Pascal's constants.
2.2. Synthesis of [Fe(bph)Pt(CN)4]·4.5H2O (1·4.5H2O-c)
K2[Pt(CN)4]·3H2O (0.02 mmol) in an aqueous solution (2 mL) was put in the bottom of a test tube, upon which 2 mL of EtOH/H2O (v/v = 1
:
1) as a buffer layer was carefully added. Then 2 mL ethanol solution of the bph ligand (0.02 mmol) and Fe(ClO4)2·6H2O (0.02 mmol) was slowly placed on top of the buffer layer. The test tube was sealed and kept undisturbed at RT for one month. Light-yellow square crystals of 1·4.5H2O-c suitable for single-crystal X-ray diffraction were obtained in a yield of 60%. FT-IR (KBr, cm−1): 3416 (s), 3201 (s), 3050 (m), 2168 (vs), 1690 (m), 1667 (s), 1552 (m), 1408 (w), 1288 (m), 1063 (w), 845 (w), 695 (w). Anal. calcd for C16H19FeN8O6.5Pt (%): C, 28.33; H, 2.82; N, 16.52; found: C, 28.51; H, 2.63; N, 16.67.
2.3. Synthesis of [Fe(bph)Pd(CN)4]·4.5H2O (2·4.5H2O-c)
The single-crystal of 2·4.5H2O-c was prepared using the same procedure as for 1·4.5H2O-c except for using K2[Pd(CN)4]·3H2O (0.02 mmol) instead of K2[Pt(CN)4]·3H2O. Yield: 47%. FT-IR (KBr cm−1): 3415 (vs), 3208 (vs), 3054 (m), 2172 (vs), 1690 (s), 1665 (s), 1556 (m), 1489 (m), 1415 (w), 1230 (w), 1067 (w), 852 (w), 756 (w). Anal. calcd for C16H19FeN8O6.5Pd (%): C, 32.59; H, 3.25; N, 19.00; found: C, 32.47; H, 3.11; N, 19.18.
2.4. Syntheses of the dehydrated complexes 1 and 2
The dehydrated complexes 1 and 2 were obtained as orange powders (Fig. S1†) by heating 1·4.5H2O-c and 2·4.5H2O-c, respectively, at 423 K for 2 hours. Yield of 1: 100%. FT-IR (KBr, cm−1): 3210 (s), 3051 (m), 2165 (vs), 1690 (s), 1633 (s), 1535 (s), 1475 (m), 1288 (m), 1063 (w), 851 (w), 761 (w). Anal. calcd for C16H10FeN8O2Pt: C, 32.18; H, 1.69; N, 18.76(%). Found: C, 32.34; H, 1.52; N, 18.57(%). Yield of 2: 100%. FT-IR (KBr, cm−1): 3221 (s), 3067 (m), 2167 (vs), 1695 (m), 1670 (s), 1555 (m), 1512 (m), 1410 (w), 1280 (m), 844 (w), 697 (w). Anal. calcd for C16H10FeN8O2Pd: C, 37.79; H, 1.98; N, 22.03(%). Found: C, 37.54; H, 1.85; N, 22.26(%).
2.5. Syntheses of the rehydrated 1·4.5H2O-p and 2·4.5H2O-p
The rehydrated 1·4.5H2O-p and 2·4.5H2O-p were produced as yellow powders (Fig. S1†) by exposure of the dehydrated complexes 1 and 2 to water for two days. Yield of 1·4.5H2O-p: 95%. FT-IR (KBr, cm−1): 3415 (s), 3212 (s), 3054 (m), 2169 (vs), 1689 (m), 1658 (m), 1555 (w), 1489 (w), 1287 (w), 1118 (m), 713 (w), 620 (m). Anal. calcd for C16H19FeN8O6.5Pt: C, 28.33; H, 2.82; N, 16.52(%); found: C, 28.58; H, 2.54; N, 16.73(%). Yield of 2·4.5H2O-p: 93%. FT-IR (KBr, cm−1): 3412 (s), 3211 (s), 3066 (m), 2171 (vs), 1688 (m), 1656 (m), 1556 (w), 1492 (w), 1285 (m), 1121 (m), 714 (w), 620 (m). Anal. calcd for C16H19FeN8O6.5Pd: C, 32.59; H, 3.25; N, 19.00(%); found: C, 32.34; H, 3.07; N, 19.21(%).
2.6. X-ray crystallography
Crystallographic data for 1·4.5H2O-c and 2·4.5H2O-c were collected on a Bruker Smart APEX II CCD diffractometer with graphite-monochromated Mo Kα radiation (λ = 0.71073 Å) at 296 and 100 K. All absorption corrections were performed using the SADABS programs. The structures were solved by direct methods and refined on F2 by full matrix least squares using SHELXTL.41 All non-hydrogen atoms were located from the Fourier maps and refined anisotropically. All hydrogen atoms were placed in calculated positions and assigned isotropic displacement parameters riding on their parent atoms. In the refinements of 1·4.5H2O-c and 2·4.5H2O-c at 296 K, the SQUEEZE routine42 in PLATON was used to include the contribution of disordered water molecules in the structural factors in the least-squares refinement. The solvent-accessible voids with a volume of 183 Å3 for 1·4.5H2O-c and 190 Å3 for 2·4.5H2O-c were identified with electron counts of 30 and 33, respectively, which reasonably corresponded to three water molecules with electron counts of 30 in the unit cell of 1·4.5H2O-c and 2·4.5H2O-c. For 1·4.5H2O-c, the water molecules were all disordered and refined with an occupancy of 0.216(9) for O1w and 0.034(19) for O1wA, and 0.0545(17) for O2w and 0.0705(17) for O2wA. For 2·4.5H2O-c, the O1w water molecule was disordered over two sites and refined with an occupancy of 0.210(8) for O1w and 0.040(8) for O1wA. At 100 K, all the lattice water molecules in the pores of 1·4.5H2O-c and 2·4.5H2O-c were definitely determined. For 1·4.5H2O-c, the water molecules were disordered and refined with an occupancy of 0.30 for O1w and 0.20 for O1wA, 0.159(10) for O2w and 0.091(10) for O2wA, 0.125 for both O3w and O3wA, and 0.0515(18) for O4w and 0.0735(18) for O4wA. For 2·4.5H2O-c, the water molecules were also disordered and refined with an occupancy of 0.644(12) for O1w and 0.356(2) for O1wA, 0.25 for O2w, O2wA, O3w and O3wA, and 0.125 for both O4w and O4wA. The crystal data and refinement parameters are summarized in Table 1, and selected bond lengths and angles are given in Table S1 in the ESI.† Hydrogen-bonding parameters are listed in Table S2.† The crystal data have been deposited in the CCDC with numbers 2142824 (296 K) and 2214856 (100 K) for 1·4.5H2O-c and 2142818 (296 K) and 2214857 (100 K) for 2·4.5H2O-c.†
Table 1 Crystallographic data for 1·4.5H2O-c and 2·4.5H2O-c at 296 and 100 K
Complexes |
1·4.5H2O-c |
2·4.5H2O-c |
Empirical formula |
C16H19FeN8O6.5Pt |
C16H19FeN8O6.5Pd |
Formula weight |
678.29 |
589.64 |
T (K) |
296 |
100 |
296 |
100 |
Crystal system |
Monoclinic |
Space group |
P2/m |
P2/m |
P2/m |
P2/c |
a (Å) |
6.9787(5) |
6.9607(16) |
6.9937(7) |
15.154(3) |
b (Å) |
7.5997(6) |
7.6127(17) |
7.6446(8) |
7.4133(15) |
c (Å) |
13.8438(10) |
13.858(3) |
13.9154(14) |
13.691(3) |
β (°) |
91.419(2) |
91.585(7) |
90.997(3) |
113.248(6) |
V (Å3) |
734.00(9) |
734.0(3) |
743.86(13) |
1413.2(5) |
Z
|
4 |
4 |
4 |
2 |
D
c (g cm−3) |
1.412 |
1.519 |
1.196 |
1.379 |
μ (mm−1) |
5.280 |
5.293 |
1.118 |
1.191 |
F (000) |
297 |
320 |
265 |
584 |
Crystal size (mm3) |
0.09 × 0.07 × 0.06 |
0.09 × 0.07 × 0.06 |
0.09 × 0.08 × 0.07 |
0.09 × 0.08 × 0.07 |
θ Range (°) |
2.920–25.003 |
2.928–25.002 |
2.664–27.608 |
2.926–25.004 |
Reflections collected |
5254 |
4467 |
6116 |
8593 |
Independent reflections |
1402 [Rint = 0.0302] |
1397 [Rint = 0.0470] |
1849 [Rint = 0.0498] |
2477 [Rint = 0.0434] |
Reflections observed [I > 2σ(I)] |
1401 |
1397 |
1651 |
1668 |
Data/restraints/parameters |
1402/37/116 |
1397/54/146 |
1849/30/108 |
2477/60/202 |
Goodness-of-fit on F2 |
1.154 |
1.073 |
1.104 |
1.089 |
R
1/wR2 [I > 2σ(I)] |
0.0302/0.0815 |
0.0307/0.0802 |
0.0538/0.1513 |
0.0511/0.1516 |
R
1/wR2 (all data) |
0.0303/0.0818 |
0.0307/0.0802 |
0.0591/0.1570 |
0.0742/0.1651 |
Max., min. Δρ (e Å−3) |
3.027, −0.817 |
0.910, −0.901 |
1.526, −1.445 |
1.234, −1.120 |
3. Results and discussion
3.1. Syntheses of 1·4.5H2O-c, 2·4.5H2O-c, 1, 2, 1·4.5H2O-p and 2·4.5H2O-p and their reversible transformation
Light-yellow square-shaped single crystals of 1·4.5H2O-c and 2·4.5H2O-c were prepared using slow liquid-to-liquid diffusion methods by layering an ethanolic solution of the bph ligand and Fe(ClO4)2·6H2O onto an aqueous solution of K2[M(CN)4]·3H2O (M = Pt2+ and Pd2+), respectively. 1·4.5H2O-c and 2·4.5H2O-c were obtained in a yield of 60 and 47%, respectively, and confirmed by single-crystal X-ray diffraction analysis. When the crystals of 1·4.5H2O-c and 2·4.5H2O-c, respectively, were heated at 423 K for 2 hours, the dehydrated complexes 1 and 2 can be quantitatively produced as orange powders. Remarkably, both 1·and 2 can be transformed into 1·4.5H2O-p and 2·4.5H2O-p as yellow powders on exposure to moisture for two days, while the latter can undergo dehydration again on heating at 423 K for two hours to regenerate 1·and 2, revealing that the transformation between the 1·4.5H2O-p and 2·4.5H2O-p and their corresponding dehydrated complexes is completely reversible (Fig. S1†). Moreover, this transformation has been fully demonstrated by elemental analysis, FT-IR, TGA and PXRD studies (Fig. S2–S7†).
The FT-IR spectra of 1·4.5H2O-c and 1, and 2·4.5H2O-c and 2 are shown in Fig. S2 and S3,† respectively. A strong broad peak at 3416 cm−1 is ascribed to the O–H vibrations of the lattice water molecules in 1·4.5H2O-c (Fig. S2a†), while the corresponding peak disappears in the dehydrated complex 1 (Fig. S2b†). A similar phenomenon is also observed in 2·4.5H2O-c and 2 (Fig. S3†). In addition, the characteristic peak at 2168, 2165, 2172 and 2167 cm−1 can be assigned to the vibrations of the –C
N group in 1·4.5H2O-c, 1, 2·4.5H2O-c and 2, respectively. An obvious peak at 3201, 3210, 3208 and 3221 cm−1 due to the N–H vibrations of the bph ligand can be found in 1·4.5H2O-c, 1, 2·4.5H2O-c and 2, respectively, whereas the C
O vibrations of the ligand are seen at 1690, 1690, 1690 and 1695 cm−1 for 1·4.5H2O-c, 1, 2·4.5H2O-c and 2, respectively. The FT-IR spectra of the rehydrated 1·4.5H2O-p and 2·4.5H2O-p are shown in Fig. S4.† A strong broad peak at 3415 and 3412 cm−1 due to the O–H vibrations of H2O can be observed in 1·4.5H2O-p and 2·4.5H2O-p, respectively, revealing that the guest water molecules can be refilled in the pores.
The TGA curves of 1·4.5H2O-c and 1, and 2·4.5H2O-c and 2 are depicted in Fig. S5,† whereas those of the corresponding rehydrated 1 and 2 are shown in Fig. S6.† The first weight loss of 11.6% from RT to 202 °C is ascribed to the removal of 4.5 lattice water molecules (calcd 12.0%) in 1·4.5H2O-c, while in the corresponding temperature range almost no loss of mass occurred for 1 (Fig. S5a†), confirming the complete dehydration in 1. The framework of 1·4.5H2O-c starts to collapse above 250 °C when further heated in contrast to 1 with a decomposition temperature of the framework at 230 °C. Similar TGA results are also found in 2·4.5H2O-c and 2 (Fig. S5b†). As shown in Fig. S6,† upon heating from RT to 205 °C, the rehydrated 1·4.5H2O-p lost a weight of 11.5% owing to the loss of 4.5 H2O molecules (calcd. 12.0%). The framework begins to decompose above 232 °C. The TGA result of the rehydrated 2·4.5H2O-p is almost similar to that of the rehydrated 1·4.5H2O-p.
The PXRD patterns of the as-synthesized 1·4.5H2O-c and 2·4.5H2O-c are exactly identical to those simulated from the single-crystal data of 1·4.5H2O-c and 2·4.5H2O-c, respectively, displaying the high phase purity of the bulk products (Fig. S7†). However, for their dehydrated counterparts 1 and 2, the PXRD peaks obviously broaden except the strongest peak centered at 2θ = 6.4° (Fig. S8 and S9†). It is noteworthy that when 1 and 2, respectively, are exposed to H2O, both the rehydrated 1·4.5H2O-p and 2·4.5H2O-p become basically isostructural to the as-synthesized 1·4.5H2O-c and 2·4.5H2O-c because the most intense PXRD peaks are clearly regenerated for the rehydrated derivatives (Fig. S8 and S9†), clearly confirming that 1·4.5H2O-p and 2·4.5H2O-p and their corresponding dehydrated products 1 and 2 can be reversibly transformed.
3.2. Single crystal structures of 1·4.5H2O-c and 2·4.5H2O-c
X-ray crystallographic studies of 1·4.5H2O-c and 2·4.5H2O-c were performed at 296 and 100 K, respectively. At 296 K, both 1·4.5H2O-c and 2·4.5H2O-c are isomorphous and crystallize in the monoclinic P2/m space group (Table 1). Due to the structural similarity of 1·4.5H2O-c and 2·4.5H2O-c, only the structure of 1·4.5H2O-c is discussed here in detail. The asymmetric unit of 1·4.5H2O-c contains a Fe2+ ion (occupancy factor of 0.25), a Pt2+ ion (occupancy factor of 0.25), a half bph ligand, one CN− anion, and one and a half highly disordered lattice water molecules (occupancy of 0.25 for O1w and 0.125 for O2w) (Fig. S10a†). Each Fe2+ center adopts a distorted octahedral [FeN6] geometry with the equatorial positions occupied by the CN− groups of [Pt(CN)4]2− anions (Fig. S11a†), giving rise to a 2D [Fe{Pt(CN)4}]n layer in the ab plane (Fig. 1a). There is an obvious undulation in the layers due to a big dihedral angle of 34.9° between the [FeN4] plane and the [Pt(CN)4]2− anion (Fig. 1b). The layers are axially pillared by the two pyridyl groups of bph ligands along the c axis to form a 3D Hofmann-type framework (Fig. 1c). Notably, although the two pyridyl rings of the bph ligands in 1·4.5H2O-c are ordered, the double amide (O
C–NH) groups that link both rings are disordered at two sites (Fig. S11a†). The axial Fe–Npy bond distance (2.210(6) Å) is slightly larger than the equatorial Fe–NCN one (2.139(4) Å) (Table S1†). The bond lengths are typical of the Fe2+ ions in the HS state at RT (vide infra).
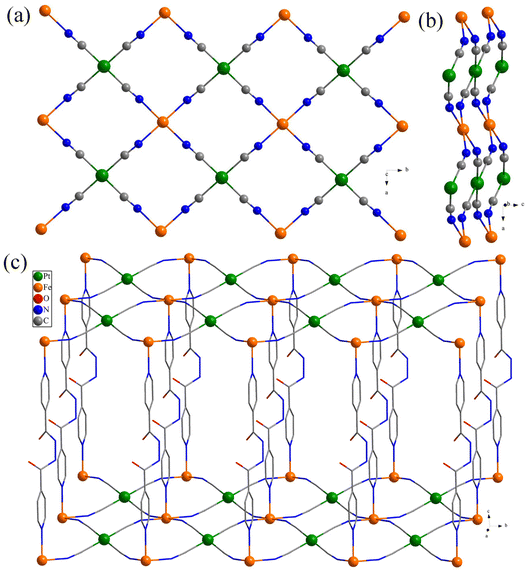 |
| Fig. 1 (a) The view of the 2D [Fe{Pt(CN)4}] layer in the ab plane; (b) the [Fe{Pt(CN)4}] layer showing an undulation along the a axis; (c) 3D Hofmann framework of 1·4.5H2O-c. Guest H2O molecules, disordered and all H atoms are omitted for clarity. | |
At 100 K, the space group and the Fe–N bond distances and angles are the same as those at 296 K without a phase transition for 1·4.5H2O-c. However, the space group of 2·4.5H2O-c at 100 K changes to monoclinic P2/c with a phase transition (Table S1†). Therefore, it is worthwhile to further discuss the low temperature structure of 2·4.5H2O-c. At 100 K, the asymmetric unit of 2·4.5H2O-c contains one 1/2-occupied Fe2+ ion, one 1/2-occupied Pd2+ ion, a half bph ligand, two CN− anions and 2.25 lattice water molecules (Fig. S10d†). Each Fe2+ center shows a distorted octahedral [FeN6] geometry with the equatorial positions occupied by the CN− groups of [Pd(CN)4]2− anions to form a 2D [Fe{Pd(CN)4}]n layer (Fig. S11d†). The adjacent 2D layers are further connected by the bph ligands to the remaining iron(II) sites axially to produce a 3D Hofmann-type framework. Notably, the dihedral angle (23.6°) between the [FeN4] plane and the [Pd(CN)4]2− anion is significantly reduced in contrast to that (35.1°) at 296 K. The two amide (O
C–NH) groups of the bph ligand in 2·4.5H2O-c are ordered at 100 K. In addition, both the axial Fe–Npy and the equatorial Fe–NCN bond lengths decrease to 1.989(4) and 1.938(4)/1.941(4) Å, respectively (Table S1†), revealing that the Fe2+ ion is in the LS state at 100 K (vide infra).
There are permanent 1D channels along the a axis in 1·4.5H2O-c at 296 K. These channels are occupied by lattice water molecules. Consequently, rich intermolecular hydrogen bonds among the host–host, host–guest and guest–guest interactions can be found in 1·4.5H2O-c (Fig. S12a†). The interactions include (1) a weak intermolecular C–H⋯O
C hydrogen bond between the pyridyl and carbonyl groups of the bph ligands belonging to the host–host interplay (C5–H5⋯O1i = 3.351(7) Å, symmetry code: (i) 1 − x, 1 − y, −z); (2) two strong intermolecular N–H⋯OW and OW–H⋯O
C hydrogen bonds involving the lattice water molecules and the hydrazide group of the bph ligands from the host–guest interplay (N3–H3⋯O1Wii = 2.757(7) Å, O1W–H1WB⋯O1i = 2.987(5) Å, symmetry code: (ii) 1 + x, y, z); (3) strong intermolecular OW–H⋯OW hydrogen bonds from the guest–guest interplays between the lattice water molecules (O1W–H1WA⋯O2WA = 3.357(4) Å, O1WA–H1WD⋯O2WA = 2.603(9) Å, and O2W–H2WA⋯O1Wii = 2.629(6) Å) (Table S2†). All these interactions generate hydrogen-bonding networks in the 1D channels (Fig. S12b†). It is the H-bonding networks that greatly prevent the 3D framework of 1·4.5H2O-c from forming the interpenetrated structure even if the flexible bph ligand is enough long. In contrast, both [Fe(dpo){Ag(CN)2}2]·3DMF and [Fe(dpo){Ag(CN)2}2]·0.5MeCN·2DEF show a doubly interpenetrated 3D framework,36 although the dpo and bph isomers have almost the same length. More importantly, the presence of extensive hydrogen-bonding networks in the 1D channels can not only further stabilize the 3D framework of 1·4.5H2O-c but also result in a strong cooperativity between the SCO-active Fe2+ centers, which will be very beneficial for modulating the spin transition properties of 1·4.5H2O-c (vide infra). Notably, the host–host, host–guest and guest–guest interactions also exist in 1·4.5H2O-c at 100 K (Fig. S14a†) and 2·4.5H2O-c at 296 (Fig. S13†) and 100 K (Fig. S14b†).
3.3. Magnetic properties of 1·4.5H2O-c, 2·4.5H2O-c, 1, 2, and the rehydrated 1·4.5H2O-p and 2·4.5H2O-p
The χMT values versus T plots (where χM is the molar magnetic susceptibility and T is the temperature) for 1·4.5H2O-c and 2·4.5H2O-c and their dehydrated products (1 and 2) are shown in Fig. 2 and 3, respectively. At 300 K, 1·4.5H2O-c exhibits a χMT value of 3.79 cm3 K mol−1, which is consistent with an octahedral Fe2+ ion in the HS state.33,36 The χMT value is almost constant down to 110 K, and then an abrupt drop occurs to reach a value of 0.84 cm3 K mol−1 at 70 K, which indicates an incomplete one-step SCO behavior with approximately 77% Fe2+ ion in the HS state transforming into the LS state. Subsequently, a slight χMT decrease is observed until 20 K with a value of 0.72 cm3 K mol−1. The decrease of the χMT product below 20 K takes place due to the zero-field splitting of the Fe2+ ion remaining in the HS state and/or antiferromagnetic interactions.31,32 The thermal dependence of the χMT value in the warming mode does not match that of the cooling mode. The critical temperature of 1·4.5H2O-c is T↓1/2 = 90 K for the cooling mode, and T↑1/2 = 150 K for the warming mode, defining a square-shaped hysteresis loop with a width of 60 K (Fig. 2a), which represents the largest hysteresis width found for the 3D Hofmann SCO frameworks built from the flexible pillars up to now (Table 2).29,32,34 Moreover, this large hysteretic SCO behavior (ΔT = 62 K) is also confirmed by the second magnetic measurements (Fig. S15a†). The abrupt thermal spin transition and the rare large hysteresis loop observed in 1·4.5H2O-c illustrated that there is a strong cooperativity between the SCO-active Fe2+ ions,29,32 which is in good agreement with the results of X-ray crystallography.
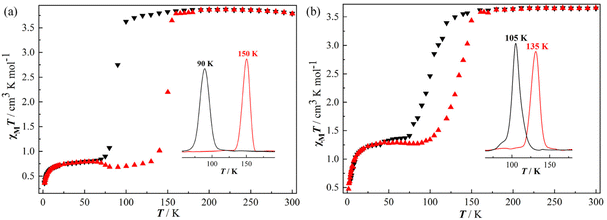 |
| Fig. 2 Variable temperature magnetic susceptibility (χMT) for 1·4.5H2O-c (a) and 2·4.5H2O-c (b) (black: cooling; red: heating; inset: ∂(χMT)/∂T showing the T1/2 values). | |
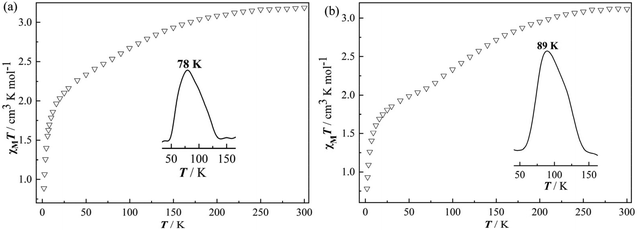 |
| Fig. 3 Variable temperature magnetic susceptibility (χMT) for 1 (a) and 2 (b); inset: ∂(χMT)/∂T showing the T1/2 values. | |
Table 2 Critical temperature (T1/2) and hysteresis width (ΔT) of some 3D Hofmann SCO frameworks [FeLM(CN)4]·G and [FeL{M′(CN)2}2]·G with flexible pillars (M = Pt, Pd; M′ = Ag, Au; L = pillar ligand; G = guest molecule)
L |
M |
G |
T
↓1/2 (K) |
T
↑1/2 (K) |
ΔT (K) |
Interpenetration |
Ref. |
The data were obtained from the second magnetic measurements.
|
bph |
Pt |
4.5H2O |
90 (88) |
150 |
60(62)a |
No |
This work |
bph |
Pd |
4.5H2O |
105 |
135(136) |
30(31)a |
No |
This work |
dpsme |
Pt |
2/3dpsme·xEtOH·yH2O |
138/127/115 |
154/148/134 |
16/21/19 |
No |
29
|
pina |
Au |
5MeOH |
199/191/144/137 |
199/193/160/154 |
0/2/16/17 |
2-Fold |
33
|
dpSe |
Pt |
|
120 |
130 |
10 |
No |
32
|
pina |
Au |
|
225 |
234 |
9 |
2-Fold |
33
|
dpyu |
Pt |
9H2O |
181 |
190 |
9 |
No |
34
|
pina |
Ag |
5MeOH |
215/179 |
220/185 |
5/6 |
2-Fold |
33
|
dpo |
Ag |
0.5MeCN·2DEF |
235/226/200/189 |
237/230/205/193 |
2/4/5/4 |
2-Fold |
36
|
dpo |
Ag |
3DMF |
203/191/160/139 |
203/191/164/143 |
0/0/4/4 |
2-Fold |
36
|
dpa |
Pt |
dpa·1.6H2O |
240/212/197 |
240/216/200 |
0/4/3 |
— |
30
|
pina |
Ag |
|
204 |
207 |
3 |
2-Fold |
33
|
dpa |
Pt |
dpa |
210/178/162 |
210/181/164 |
0/3/2 |
— |
30
|
dpyu |
Pt |
|
292/195 |
292/195 |
0/0 |
No |
34
|
bpmb |
Ag |
0.5DMF·0.5EtOH·2H2O |
232/216 |
232/216 |
0/0 |
2-Fold |
31
|
bpmb |
Au |
DMF·0.5EtOH·H2O |
204/134 |
204/134 |
0/0 |
2-Fold |
31
|
dbdpe |
Pd |
4H2O |
187 |
187 |
0 |
No |
35
|
dbdpe |
Pt |
4H2O |
185 |
185 |
0 |
No |
35
|
The palladium analogue, 2·4.5H2O-c exhibits a χMT value of 3.65 cm3 K mol−1 at 300 K showing that 100% Fe2+ ion is in the HS state (Fig. 2b).33 This value is practically constant until 140 K, and then decreases relatively sharply to reach a value of 1.38 cm3 K mol−1 at 70 K corresponding to the 39%HS/61%LS state. Further cooling of the sample causes a slight drop in χMT with a value of 1.19 cm3 K mol−1 at 20 K. Afterward, the χMT value decreases to 0.44 cm3 K mol−1 at 1.8 K because of the zero-field splitting and/or antiferromagnetic interactions of the HS Fe2+ ion.31,32 The χMT values versus T plots in the heating mode do not match those in the cooling one giving an irregular square-shaped hysteresis loop of 30 K (T↓1/2 = 105 K and T↑1/2 = 135 K) (Fig. 2b),35 which can also be verified by the second magnetic measurements (Fig. S15b†). Thus, 2·4.5H2O-c shows a narrower hysteresis loop and more incomplete HS ↔ LS thermal spin transition compared with 1·4.5H2O-c. This SCO difference may mainly result from the phase transition found in 2·4.5H2O-c when the temperature decreases from RT to 100 K, as confirmed by the X-ray crystallographic studies. In addition, the different ionic radii of the Pt2+ and Pd2+ ions could also result in the SCO difference between 1·4.5H2O-c and 2·4.5H2O-c to some extent.12
As shown in Fig. 3, the dehydrated complexes 1 and 2 display similar changes in the magnetic properties. At 300 K, the χMT values of 1 and 2, 3.18 and 3.12 cm3 K mol−1, respectively, are somewhat lower than those of the corresponding hydrated products. However, upon further cooling, an incomplete and more gradual HS → LS spin transition occurs between 230 and 40 K for both 1 and 2, which are quite different from those observed in the above 1·4.5H2O-c and 2·4.5H2O-c. The χMT achieves a value of 2.26 cm3 K mol−1 for 1 and 1.93 cm3 K mol−1 for 2 at 40 K, corresponding to the 29% HS Fe2+ ion in 1 (T1/2 = 78 K) and 38% in 2 (T1/2 = 89 K) to undergo spin conversion. Furthermore, the χMT curve obtained by the heating mode is exactly identical to that by the cooling one, revealing no hysteresis loop in the conversion of 1 and 2. Compared with 1·4.5H2O-c and 2·4.5H2O-c, both 1 and 2 retain higher percentages of the HS Fe2+ ions at low temperatures, explaining that the spin transitions from the HS to the LS states in 1 and 2 are more difficult to happen owing to the lack of lattice water molecules.
As shown in Fig. 4, both the rehydrated 1·4.5H2O-p and 2·4.5H2O-p exhibit incomplete two-step SCO behaviors with small hysteresis loops but higher transition temperatures compared with 1·4.5H2O-c and 2·4.5H2O-c. At 300 K, the χMT value of 1·4.5H2O-p is close to 3.62 cm3 K mol−1, indicating a typical HS state. The value slightly decreases to 3.38 cm3 K mol−1 at 260 K and then declines gradually. Upon further cooling, the χMT value reaches 1.61 cm3 K mol−1 at 160 K. Further cooling of the sample gives a χMT value of 1.24 cm3 K mol−1 at 20 K. Finally, the χMT value decreases to 0.66 cm3 K mol−1 at 1.8 K because of the zero-field splitting and/or antiferromagnetic interactions of the HS Fe2+ ion.31,32 The 1st derivative of the χMT–T curve suggests a two-step SCO behavior, although their magnetic plateaus are not obvious. The T1/2 values are 263 and 230 K in the cooling mode, and 274 and 245 K in the warming mode. Therefore, two small hysteresis loops (11 and 15 K) are observed in 1·4.5H2O-p (Fig. 4a). A similar SCO behavior is also found in 2·4.5H2O-p but with two smaller hysteresis loops of 4 K (T↓1/2 = 249 K and T↑1/2 = 253 K) and 8 K (T↓1/2 = 215 K and T↑1/2 = 223 K) (Fig. 4b).
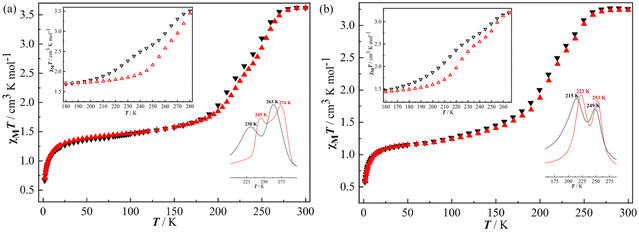 |
| Fig. 4 Variable temperature magnetic susceptibility (χMT) for rehydrated 1·4.5H2O-p (a) and 2·4.5H2O-p (b); inset: ∂(χMT)/∂T showing the T1/2 values and hysteresis loops. | |
3.4. Magneto-structural correlation
Although the bph and dpo isomers have a similar length, their resulting 3D Hofmann SCO frameworks, 1·4.5H2O-c and [Fe(dpo){Ag(CN)2}2]·3DMF show entirely different SCO modes, i.e. one-step abrupt spin transition with a large hysteresis loop (60 K) for 1·4.5H2O-c and a four-step spin-transition with small hysteresis loops (4 K) for [Fe(dpo){Ag(CN)2}2]·3DMF (Table 2). The main differences in their SCO properties may be caused by the different structures. Firstly, [Fe(dpo){Ag(CN)2}2]·3DMF possesses a 2-fold interpenetration 3D framework while 1·4.5H2O-c does not. Secondly, only relatively weak hydrogen-bonding interactions between the dpo ligands and DMF molecules (N2–H2⋯O4 = 2.834(19) Å and N3–H3⋯O3 = 2.881(19) Å) are observed in [Fe(dpo){Ag(CN)2}2]·3DMF whereas strong intermolecular hydrogen bonds involving the bph ligands and H2O molecules (N3–H3⋯O1Wii = 2.757(7) Å) have been found in 1·4.5H2O-c (Table S2†). Finally, the relatively rigid [Pt(CN)4]2− units in 1·4.5H2O-c in contrast to the [Ag(CN)2]− anions in [Fe(dpo){Ag(CN)2}2]·3DMF will be favorable for improving the cooperativity of the SCO-active Fe2+ ions in 1·4.5H2O-c to a certain extent. In addition, by carefully analyzing the structural data for 3D Hofmann SCO frameworks with the flexible pillars (Table 2), it is interesting to note that the 2-fold interpenetrated frameworks with the bpmb,31 pina33 and dpo36 pillars often exhibit guest dependent multi-step SCO behaviors while the non-interpenetrated frameworks with dpSe,32 dpyu34 and dbdpe35 pillars generally indicate one-step SCO behaviors. A rare exception is the 3D Hofmann SCO framework [Fe(dpsme)Pt(CN)4]·2/3dpsme·xEtOH·yH2O,29 which can display the complete three-step SCO behavior with a ca. 20 K hysteresis loop but does not show any interpenetrated structure due to the large non-coordinated dpsme ligand occupying the channels. Consequently, the SCO behavior of 1·4.5H2O-c is slightly similar to that observed in the 3D Hofmann non-interpenetrated framework [Fe(dpyu){Pt(CN)4}]·9H2O which exhibits a one-step incomplete spin transition with a hysteresis loop of 9 K,34 although the bph ligand containing a dihydrazide unit and the dpyu ligand with a urea backbone are obviously different and there are different numbers of lattice water molecules. However, at this stage it is very difficult to draw a simple magneto-structural correlation among the structures, ligands, guest molecules and spin transition behaviors for the 3D Hofmann SCO frameworks built from the flexible pillars due to the limited available crystal structural data.30
Notably, compared with the 3D Hofmann SCO frameworks with the rigid pillars, the 60 K hysteresis of 1·4.5H2O-c is also the top 4 (Table S3†). The hysteresis width of 1·4.5H2O-c is only lower than those (95 and 73 K) reported for two SCO complexes, [Fe(bpe)2{Ag(CN)2}2] (bpe = 1,2-bis(4-pyridyl)ethylene)43 and [Fe(dpb){Au(CN)2}2]·0.7naphthalene (dpb = 1,4-di(4-pyridyl)benzene),19 respectively, and comparable to that (64 K) of [Fe(pz)Pt(CN)4]·0.5(CS(NH2)2).44 The rare SCO properties of 1·4.5H2O-c can be ascribed to the strong cooperativity of the SCO-active Fe2+ ions due to the host–host, host–guest and guest–guest interactions. Unfortunately, the low-temperature crystal data below 100 K were not available to us, thus, it is impossible to further study the magneto-structural correlation of 1·4.5H2O-c at the current stage.
After dehydration of 1·4.5H2O-c, the resulting dehydrated complex 1 completely lost crystallinity, so it is impossible for us to establish a magneto-structural correlation for 1. However, the gradual and incomplete spin transition without any hysteresis loop observed in 1 may be tentatively attributed to the weak cooperativity of the SCO-active Fe2+ ions because of the absence of host–guest interactions resulting from the removal of the lattice water molecules. This phenomenon may provide disproof for the SCO behavior of 1·4.5H2O-c, that is, the host–guest interactions between the dihydrazide group of the bph ligands and lattice water molecules may be an indispensible factor for enhancement of the cooperativity in 1·4.5H2O-c.
It is worthwhile to note that although 1·4.5H2O-c and 2·4.5H2O-c are isostructural, the hysteresis width (60 K) of 1·4.5H2O-c is two times larger than that (30 K) of 2·4.5H2O-c. By carefully comparing the crystal structures at 296 K, it is found that the equatorial Fe–NCN bond length (2.139(4) Å), the ∠Fe–N
C angle (157.78(4)°) and Fe⋯Fe distances (10.318(2)/15.657(2) Å) in 1·4.5H2O-c are all smaller than the corresponding values (2.148(3) Å; 159.42(3)°; 10.361(2)/15.682(2) Å) in 2·4.5H2O-c (Table S1†). In addition, there are four kinds of intermolecular OW–H⋯O hydrogen bonds in 1·4.5H2O-c but only three in 2·4.5H2O-c (Table S2†). Furthermore, the extensive hydrogen-bonding networks existing in the 1D channels of 1·4.5H2O-c (Fig. S12b†) are obviously different from those of 2·4.5H2O-c (Fig. S13b†). These results reveal that there is a stronger cooperativity of the SCO-active Fe2+ ions in 1·4.5H2O-c in contrast to 2·4.5H2O-c, which may contribute to producing a larger hysteresis loop in 1·4.5H2O-c. Most importantly, variable-temperature single-crystal data revealed that there was a phase transition from the monoclinic P2/m space group at 296 K to the P2/c one at 100 K for 2·4.5H2O-c, while no phase transition was observed for 1·4.5H2O-c. This unique feature may result in the different SCO behavior in 2·4.5H2O-c.
4. Conclusion
Using a flexible bispyridyl ligand (bph), we have synthesized two new 3D Hofmann-type SCO frameworks [Fe(bph)M(CN)4]·4.5H2O (M = Pt2+ for 1·4.5H2O-c and Pd2+ for 2·4.5H2O-c). Both 1·4.5H2O-c and 2·4.5H2O-c indicate abrupt one-step SCO behaviors with a hysteresis loop of 60 and 30 K, respectively. To our knowledge, this is the largest hysteresis width reported for 3D Hofmann SCO frameworks with flexible pillars until now. However, the corresponding dehydrated complexes 1 and 2 merely undergo gradual spin transitions without hysteresis. The strong cooperativity resulting from the extensive hydrogen-bonding networks among the host–host, host–guest and guest–guest interactions should be responsible for the rare SCO behaviors of 1·4.5H2O-c and 2·4.5H2O-c. Interestingly, both the dehydrated complexes 1 and 2 and their rehydrated 1·4.5H2O-p and 2·4.5H2O-p can be reversibly transformed by simply heating/rehydrating, together with an apparent change of color. Moreover, both 1·4.5H2O-p and 2·4.5H2O-p merely exhibit incomplete two-step SCO behaviors with small hysteresis loops but higher transition temperatures compared with 1·4.5H2O-c and 2·4.5H2O-c. This work reveals that the hydration/dehydration of guest water molecules can greatly affect the SCO behaviors, and a rational choice of flexible pillar ligands would be an important strategy to design and construct new 3D Hofmann-type SCO materials.
Conflicts of interest
There are no conflicts to declare.
Acknowledgements
We greatly appreciate the financial support from the National Natural Science Foundation of China (No. 21476115) and the Open Foundation of the State Key Laboratory of Coordination Chemistry, Nanjing University (SKLCC2113). Z. F. acknowledges the support from the Postgraduate Research & Practice Innovation Program of Jiangsu Province (KYCX21_1177).
References
- O. Kahn and C. J. Martinez, Spin-transition polymers: from molecular materials toward memory devices, Science, 1998, 279, 44–48 CrossRef CAS.
- M. A. Halcrow, Structure: function relationships in molecular spin-crossover complexes, Chem. Soc. Rev., 2011, 40, 4119–4142 RSC.
- D.-R. Zhu, L. Qi, H.-M. Cheng, X. Shen and W. Lu, Fe(II) spin crossover molecule-based materials, Prog. Chem., 2009, 21, 1187–1198 CAS.
- T. Shiga, R. Saiki, L. Akiyama, R. Kumai, D. Natke, F. Renz, J. M. Cameron, G. N. Newton and H. Oshio, A brønsted-ligand-based iron complex as a molecular switch with five accessible states, Angew. Chem., Int. Ed., 2019, 58, 5658–5662 CrossRef CAS.
- D.-R. Zhu, Y. Xu, Z. Yu, Z.-J. Guo, H. Sang, T. Liu and X.-Z. You, A novel bis(trans-thiocyanate)iron(II) spin-transition molecular material with bidentate triaryltriazole ligands and its bis(cis-thiocyanate)iron(II) high-spin isomer, Chem. Mater., 2002, 14, 838–843 CrossRef CAS.
- G.-P. Shen, L. Qi, L. Wang, Y. Xu, J.-J. Jiang, D.-R. Zhu, X.-Q. Liu and X.-Z. You, Spin-crossover in a trans-[FeL2(NCS)2] family (L = triaryltriazole): remote substituent effects on spin transition modes and temperature, Dalton Trans., 2013, 42, 10144–10152 RSC.
- Y.-H. Luo, M. Nihei, G.-J. Wen, B.-W. Sun and H. Oshio, Ambient-temperature spin-state switching achieved by protonation of the amino group in [Fe(H2Bpz2)2(bipy-NH2)], Inorg. Chem., 2016, 55, 8147–8152 CrossRef CAS.
- Z.-P. Ni, J.-L. Liu, M. N. Hoque, W. Liu, J.-Y. Li, Y.-C. Chen and M.-L. Tong, Recent advances in guest effects on spin-crossover behavior in Hofmann-type metal-organic frameworks, Coord. Chem. Rev., 2017, 335, 28–43 CrossRef CAS.
- T. Shiga, Y. Sato, M. Tachibana, H. Sato, T. Matsumoto, H. Sagayama, R. Kumai, Y. Murakami, G. N. Newton and H. Oshio, Carboxylic acid functionalized spin-crossover iron(II) grids for tunable switching and hybrid electrode fabrication, Inorg. Chem., 2018, 57, 14013–14017 CrossRef CAS PubMed.
- V. Niel, J. M. Martinez-Agudo, M. C. Muñoz, A. B. Gaspar and J. A. Real, Cooperative spin crossover behavior in cyanide-bridged Fe(II)-M(II) bimetallic 3D Hofmann-like networks (M = Ni, Pd, and Pt), Inorg. Chem., 2001, 40, 3838–3839 CrossRef CAS PubMed.
- G. Agustí, S. Cobo, A. B. Gaspar, G. Molnár, N. O. Moussa, P. A. Szilágyi, V. Pálfi, C. Vieu, M. C. Muñoz, J. A. Real and A. Bousseksou, Thermal and light-induced spin crossover phenomena in new 3D Hofmann-like microporous metal-organic frameworks produced as bulk materials and nanopatterned thin films, Chem. Mater., 2008, 20, 6721–6732 CrossRef.
- C. Bartual-Murgui, N. A. Ortega-Villar, H. J. Shepherd, M. C. Muñoz, L. Salmon, G. Molnár, A. Bousseksou and J. A. Real, Enhanced porosity in a new 3D Hofmann-like network exhibiting humidity sensitive cooperative spin transitions at room temperature, J. Mater. Chem., 2011, 21, 7217–7222 RSC.
- F. J. Muñoz-Lara, A. B. Gaspar, M. C. Muñoz, M. Arai, S. Kitagawa, M. Ohba and J. A. Real, Sequestering aromatic molecules with a spin-crossover FeII microporous coordination polymer, Chem. – Eur. J., 2012, 18, 8013–8018 CrossRef PubMed.
- F. J. Muñoz-Lara, A. B. Gaspar, M. C. Muñoz, V. Ksenofontov and J. A. Real, Novel iron(II) microporous spin-crossover coordination polymers with enhanced pore size, Inorg. Chem., 2013, 52, 3–5 CrossRef.
- L. Piñeiro-López, M. Seredyuk, M. C. Muñoz and J. A. Real, Two- and one-step cooperative spin transitions in Hofmann-like clathrates with enhanced loading capacity, Chem. Commun., 2014, 50, 1833–1835 RSC.
- J. E. Clements, J. R. Price, S. M. Neville and C. J. Kepert, Perturbation of spin crossover behavior by covalent post-synthetic modification of a porous metal-organic framework, Angew. Chem., Int. Ed., 2014, 53, 10164–10168 CrossRef CAS.
- H.-T. Xu, Z.-L. Xu and O. Sato, Water-switching of spin crossover in a gold cluster supramolecular system: from metal-organic frameworks to catenane, Microporous Mesoporous Mater., 2014, 197, 72–76 CrossRef CAS.
- J.-Y. Li, Y.-C. Chen, Z.-M. Zhang, W. Liu, Z.-P. Ni and M.-L. Tong, Tuning the spin-crossover behaviour of a hydrogen-accepting porous coordination polymer by hydrogen-donating Guests, Chem. – Eur. J., 2015, 21, 1645–1651 CrossRef CAS PubMed.
- J.-Y. Li, C.-T. He, Y.-C. Chen, Z.-M. Zhang, W. Liu, Z.-P. Ni and M.-L. Tong, Tunable cooperativity in a spin-crossover Hoffman-like metal-organic framework material by aromatic guests, J. Mater. Chem. C, 2015, 3, 7830–7835 RSC.
- J. E. Clements, J. R. Price, S. M. Neville and C. J. Kepert, Hysteretic four-step spin crossover within a three-dimensional porous Hofmann-like material, Angew. Chem., Int. Ed., 2016, 55, 15105–15109 CrossRef CAS PubMed.
- K. Hosoya, S. Nishikiori, M. Takahashi and T. Kitazawa, Spin-crossover behavior of Hofmann-type-like complex Fe(4,4′-bipyridine)Ni(CN)4·nH2O depending on guest species, Magnetochemistry, 2016, 2, 8 CrossRef.
- W. Liu, Y.-Y. Peng, S.-G. Wu, Y.-C. Chen, M. N. Hoque, Z.-P. Ni, X.-M. Chen and M.-L. Tong, Guest-switchable multi-step spin transitions in an amine-functionalized metal-organic framework, Angew. Chem., Int. Ed., 2017, 56, 14982–14986 CrossRef CAS.
- F.-L. Liu, D. Li, L.-J. Su and J. Tao, Reversible three equal-step spin crossover in an iron(II) Hofmann-type metal-organic framework, Dalton Trans., 2018, 47, 1407–1411 RSC.
- Y. Meng, Y.-J. Dong, Z. Yan, Y.-C. Chen, X.-W. Song, Q.-W. Li, C.-L. Zhang, Z.-P. Ni and M.-L. Tong, A new porous three-dimensional iron(II) coordination polymer with solvent-induced reversible spin-crossover behavior, Cryst. Growth Des., 2018, 18, 5214–5219 CrossRef CAS.
- C.-J. Zhang, K.-T. Lian, G.-Z. Huang, S. Bala, Z.-P. Ni and M.-L. Tong, Hysteretic four-step spin-crossover in a 3D Hofmann-type metal-organic framework with aromatic guest, Chem. Commun., 2019, 55, 11033–11036 RSC.
- M. Meneses-Sánchez, L. Piñeiro-López, T. Delgado, C. Bartual-Murgui, M. C. Muñoz, C. P. Chakraborty and J. A. Real, Extrinsic vs. intrinsic luminescence and their interplay with spin crossover in 3D Hofmann-type coordination polymers, J. Mater. Chem. C, 2020, 8, 1623–1633 RSC.
- K.-T. Lian, W.-W. Wu, G.-Z. Huang, Y. Liu, S.-G. Wu, Z.-P. Ni and M.-L. Tong, Reversible step spin crossover modulation via water absorption and dehydration in a 3D Hofmann-type framework, Inorg. Chem. Front., 2021, 8, 4334–4340 RSC.
- K.-P. Xie, Z.-Y. Ruan, B.-H. Lyu, X.-X. Chen, X.-W. Zhang, G.-Z. Huang, Y.-C. Chen, Z.-P. Ni and M.-L. Tong, Guest-driven light-induced spin change in an azobenzene loaded metal-organic framework, Angew. Chem., Int. Ed., 2021, 60, 27144–27150 CrossRef CAS.
- N. F. Sciortino, K. R. Scherl-Gruenwald, G. Chastanet, G. J. Halder, K. W. Chapman, J.
F. Létard and C. J. Kepert, Hysteretic three-step spin crossover in a thermo- and photochromic 3D pillared Hofmann-type metal-organic framework, Angew. Chem., Int. Ed., 2012, 51, 10154–10158 CrossRef CAS PubMed.
- R. Ohtani, M. Arai, A. Hori, M. Takata, S. Kitao, M. Seto, S. Kitagawa and M. Ohba, Modulation of spin-crossover behavior in an elongated and flexible Hofmann-type porous coordination polymer, J. Inorg. Organomet. Polym., 2013, 23, 104–110 CrossRef CAS.
- J.-Y. Li, Z.-P. Ni, Z. Yan, Z.-M. Zhang, Y.-C. Chen, W. Liu and M.-L. Tong, Cyanide-bridged bimetallic 3D Hoffman-like coordination polymers with tunable magnetic behaviour, CrystEngComm, 2014, 16, 6444–6449 RSC.
- N. F. Sciortino, S. M. Neville, J. F. Létard, B. Moubaraki, K. S. Murray and C. J. Kepert, Thermal- and light-induced spin-crossover bistability in a disrupted Hofmann-type 3D framework, Inorg. Chem., 2014, 53, 7886–7893 CrossRef CAS PubMed.
- F. J. Valverde-Muñoz, C. Bartual-Murgui, L. Piñeiro-López, M. C. Muñoz and J. A. Real, Influence of host-guest and host-host interactions on the spin-crossover 3D Hofmann-type clathrates {FeII(pina)[MI(CN)2]2}·xMeOH (MI = Ag, Au), Inorg. Chem., 2019, 58, 10038–10046 CrossRef PubMed.
- D. J. Mondal, S. Roy, J. Yadav, M. Zeller and S. Konar, Solvent-induced reversible spin-crossover in a 3D Hofmann-type coordination polymer and unusual enhancement of the lattice cooperativity at the desolvated state, Inorg. Chem., 2020, 59, 13024–13028 CrossRef CAS.
- Y. Li, Q.-R. Kong, Y. Guo and Z. Tang, Thermal hysteresis induced by external pressure in a 3D Hofmann-type SCO-MOF, Dalton Trans., 2021, 50, 1384–1389 RSC.
- S.-G. Wu, S. Bala, Z.-Y. Ruan, G.-Z. Huang, Z.-P. Ni and M.-L. Tong, Four-step spin-crossover in an oxamide-decorated metal-organic framework, Chin. Chem. Lett., 2022, 33, 1381–1384 CrossRef CAS.
- S. Bonhommeau, G. Molnár, A. Galet, A. Zwick, J. A. Real, J. J. McGarvey and A. Bousseksou, One shot laser pulse induced reversible spin transition in the spin-crossover complex [Fe(C4H4N2){Pt(CN)4}] at room temperature, Angew. Chem., 2005, 117, 4137–4141 CrossRef.
- M. Du, Z.-H. Zhang, X.-G. Wang and X.-J. Zhao, A 3-D (3,5)-connected CdII coordination framework with unique twofold interpenetrating (4·62)(4·66·83) network topology, Inorg. Chim. Acta, 2009, 362, 1358–1360 CrossRef CAS.
- N. M. Krymova, V. E. Ivanov and N. A. Ostankevich, Complexes of copper(II) and calcium with N,N‘-diisonicotinoylhydrazine, Pharm. Chem. J., 1982, 16, 114–117 CrossRef.
- M. Du, Z.-H. Zhang, X.-G. Wang, H.-F. Wu and Q. Wang, Flexible building blocks of N,N‘-bis(picolinoyl)hydrazine for hydrogen-bonding directed cocrystallization: structural diversity, concomitant polymorphs, and synthon prediction, Cryst. Growth Des., 2006, 6, 1867–1875 CrossRef CAS.
- G. Sheldrick, Crystal structure refinement with SHELXL, Acta Crystallogr., Sect. C: Struct. Chem., 2015, 71, 3–8 Search PubMed.
- A. L. Spek, PLATON SQUEEZE: a tool for the calculation of the disordered solvent contribution to the calculated structure factors, Acta Crystallogr., Sect. C: Struct. Chem., 2015, 71, 9–18 CrossRef CAS PubMed.
- V. Niel, M. C. Muñoz, A. B. Gaspar, A. Galet, G. Levchenko and J. A. Real, Thermal-, pressure-, and light-induced spin transition in novel cyanide-bridged FeII-AgI bimetallic compounds with three-dimensional interpenetrating double structures {FeIILx[Ag(CN)2]2}·G, Chem. – Eur. J., 2002, 8, 2446–2453 CrossRef CAS.
- F. J. M. Lara, A. B. Gaspar, D. Aravena, E. Ruiz, M. C. Muñoz, M. Ohba, R. Ohtani, S. Kitagawa and J. A. Real, Enhanced bistability by guest inclusion in Fe(II) spin crossover porous coordination polymers, Chem. Commun., 2012, 48, 4686–4688 RSC.
Footnote |
† Electronic supplementary information (ESI) available: The reversible transformation between the dehydrated and rehydrated complexes, FT-IR spectra, TGA curves, PXRD patterns, molecular structures, bond distances and angles, and hydrogen bond interactions. CCDC 2142824 (1·4.5H2O-c, 296 K), 2214856 (1·4.5H2O-c, 100 K), 2142818 (2·4.5H2O-c, 296 K) and 2214857 (2·4.5H2O-c, 100 K). For ESI and crystallographic data in CIF or other electronic format see DOI: https://doi.org/10.1039/d2qi01873j |
|
This journal is © the Partner Organisations 2023 |
Click here to see how this site uses Cookies. View our privacy policy here.