DOI:
10.1039/D2QI01902G
(Research Article)
Inorg. Chem. Front., 2023,
10, 325-334
Molten-salt-induced phosphorus vacancy defect engineering of heterostructured cobalt phosphides for efficient overall water splitting†
Received
2nd September 2022
, Accepted 8th November 2022
First published on 17th November 2022
Abstract
Cobalt phosphides (CoPx) as a representative of transition metal phosphide (TMP) catalysts show great potential for highly-efficient electrocatalytic water splitting for H2 production. However, the current synthetic strategies of CoPx are complex and time-consuming. Herein, a novel, facile and fast molten salt strategy for one-step synthesis of CoPx (CoP/Co2P) was first proposed. Moreover, the molten salt's strong polarization force endows CoPx with abundant phosphorus vacancy (PV) defects. The synthesized CoPx show impressive catalytic activities towards both the hydrogen evolution reaction (HER) and oxygen evolution reaction (OER) under alkaline conditions. This enables the overall water splitting to reach the current densities of 10 and 100 mA cm−2 driven by only 1.75 and 1.90 V voltage, respectively. Mechanism investigation reveals that PV defects lead to the optimization of the electronic structure, decreased energy barriers of intermediates’ formation, and enhanced electronic conductivity, all of which boost the electrocatalytic activity. This study provides a paradigm of using molten salt for the synthesis of advanced TMPs, which not only simplifies the synthetic procedures, but also provides insight into defect engineering that involves the use of molten salt medium to obtain a better activity.
1. Introduction
Renewable-energy-driven electrochemical water splitting provides one promising approach towards green H2 production.1 This process requires efficient electrocatalysts to overcome the energy barriers. Materials based on noble metals such as Pt and Ru/Ir are known as the state-of-the-art catalysts for the hydrogen evolution reaction (HER) and oxygen evolution reaction (OER), respectively; nevertheless, the scarcity and high cost hinder their large-scale applications.2,3 In this context, tremendous efforts have been devoted to developing noble-metal-free electrocatalysts with high performance.
Transition metal phosphides (TMPs, M includes Fe, Co, Ni, Cu, Mo, W, etc.) catalyzing energy conversion and storage reactions (e.g. hydrogenation, rechargeable batteries, electrocatalytic water splitting, etc.) have drawn growing attention in recent years due to their Earth abundance, unique hydrogenase-like structure, and excellent catalytic activity.4–7 Some tactics including nanostructural engineering, heteroatom doping and heterostructural engineering have been proposed to further enhance TMPs’ performance.8 Here, a novel molten salt strategy was proposed to synthesize a TMP catalyst of cobalt phosphides (CoPx) and simultaneously carry out phosphorus vacancy (PV) defect engineering for efficient water splitting. Defect engineering capable of modulating the electronic structure and thus influencing the chemisorption and catalytic process is one important strategy to improve catalysts’ activity.9 Though defect engineering has been extensively studied in transition metal oxides (TMOs),10,11 transition metal dichalcogenides (TMDs),12,13 and defective graphene supports,14 relevant research on TMPs is limited. In transition metal compounds, intrinsic defects were classified into anionic and cationic defects on the basis of the vacancy type, and both of them will cause local electron redistribution.15 PV defects belong to the class of anionic defects, but it requires more research efforts to reveal their regulation and impact on the electronic structure of TMPs. Moreover, compared to the various mature methods for defect engineering of TMOs and TMDs, the existing methods to regulate PV defects over TMPs are only limited to NaBH4 reduction,16 plasma etching,17 and oxygen doping.18 More distinct and in-depth research studies on PV defect regulation and how it promotes the catalytic process are highly expected.
For the first time, we proposed a molten-salt-mediated phosphidation (MSMP) strategy to synthesize cobalt phosphide (CoPx, CoP/Co2P) in only one step, and meanwhile achieve an enrichment of PV defects in CoPx, resulting in a substantially modulated electronic structure and an improvement in electrocatalytic activity. Molten salt is a phase changing material and represents a significant class of fluids for high-temperature applications.19 Recent studies showed that molten salt can serve as a medium for the synthesis of advanced functional materials, such as single atom catalysts (SACs),20,21 semiconductors,22 and two dimensional (2D) MXenes23 because of its unique liquid feature, strong polarization force and space confinement effect. Given that liquids have the merits of optimal heat control, fast mass transfer, and robust dynamics,21 material synthesis in a liquid environment would be more favorable than that in a gas or solid medium.
Using the MSMP strategy, CoPx nanorods were obtained by directly heating CoSO4 and NaH2PO2 in molten LiCl–KCl. CoSO4 was first converted into cobalt (Co) oxides and then reacted with NaH2PO2-derived PH3 to form CoPx. This one-step synthesis strategy greatly simplifies the fabrication procedure of CoPx. In previous studies, CoPx synthesis required at least two steps: (i) pre-treating inorganic Co salts to synthesize Co oxides, hydroxides and metal organic frameworks (MOFs) that are usually used as Co precursors and (ii) the phosphidation of the above Co precursors (Table S8, ESI†). The CoPx nanorods synthesized in molten salt possess abundant PV defects compared to the control and exhibit a boosted catalytic activity towards overall water splitting. Density functional theory (DFT) calculations were conducted to reveal the underlying promotion mechanism of PV defects towards catalytic activity. Formation of nickel phosphides (NiPx, including NiP, Ni2P and NiP2) and ferric phosphide (Fe2P) with the molten salt strategy confirms that the reported methodology can be extended to other TMPs’ preparation. This study provides a new opportunity for facile, fast, and one-step synthesis of efficient TMPs. Furthermore, the presented MSMP method extends the strategies for defect engineering, which may inspire the synthesis of more advanced materials in the future.
2. Experimental section
Chemicals
All chemicals used in this work were purchased from commercial sources and used without any further treatment. KCl (99.5%) was provided by Aladdin; LiCl·H2O (≥97.0%) was provided by XL Scientific; CoSO4·7H2O (99.5%) was provided by MACKLIN; NaH2PO2 (99.0%) was provided by RHAWN. H2IrCl6·xH2O was provided by Aladdin. Carbon fiber paper (CFP) was purchased from TORAY Company of Japan and employed as the catalytic support. Nafion solution (5 wt%) was provided by Alfa Aesar. All aqueous solutions were prepared using Milli-Q ultrapure water (18 MΩ cm).
Synthetic procedures
In this work, the synthesis of CoPx was based on a novel and one-step MSMP strategy. Firstly, KCl (1.41 g), LiCl·H2O (1.12 g), NaH2PO2 (1.00 g) and CoSO4·7H2O (0.53 g) (the molar ratio of phosphorus (P) to Co is approximately 5
:
1) were mixed and ground into a homogeneous mixture in an agate mortar. Afterwards, the mixture was transferred into a ceramic boat and subjected to annealing treatment in a tube furnace under a N2 flow (100 mL min−1) with a temperature programming of 10 °C min−1. The mixture was kept at desired temperatures for 2 h. Thereafter, the resulting products were washed with deionized water (DI H2O) and hydrochloric acid (HCl, 100 mL, 1 M) with the aid of bath sonication to remove the frozen salt (LiCl-KCl) and to possibly form cobalt oxides. The products were separated from the solvent by centrifugation at 8000 rpm. Finally, the products were washed with DI H2O again to remove the impurities and residual HCl and then dried in a vacuum oven at 60 °C overnight. To study the effect of heating temperature on the catalytic performance, the heating temperature was set as 350, 400 and 500 °C, with the corresponding products denoted as MS-CoPx-350, MS-CoPx-400 and MS-CoPx-500, respectively. For further comparison, the samples were prepared by directly annealing the mixture of NaH2PO2 and CoSO4·7H2O without LiCl-KCl at 350, 400 and 500 °C and denoted as CoPx-350, CoPx-400 and CoPx-500, respectively. Purification procedures of CoPx-350, CoPx-400 and CoPx-500 were the same as that of MS-CoPx-350, MS-CoPx-400 and MS-CoPx-500. MS-NiPx-350, NiPx-350, MS-FePx-350 and FePx-350 were also synthesized and purified with the same procedures as that used for CoPx.
3. Results and discussion
Microscopy characterization to reveal the structural features
Using the MSMP strategy (Fig. 1a), NaH2PO2 and CoSO4·7H2O were mixed with LiCl and KCl directly, and then heated under a N2 flow to give rise to the growth of CoPx. The samples prepared at 350, 400, and 500 °C in molten salt were denoted as MS-CoPx-350, MS-CoPx-400, and MS-CoPx-500, respectively; while the control samples prepared without molten salt were denoted as CoPx-350, CoPx-400, and CoPx-500, respectively (Fig. S1†). All samples were first characterized by scanning electron microscopy (SEM) to reveal their surface morphology. MS-CoPx-350 shows a nanorod-like structure with a diameter of ∼100 nm (Fig. 1b), while CoPx-350 is shown to be amorphous (Fig. S2†). The nanorod formation of MS-CoPx-350 can be ascribed to the appropriate synthetic temperature and template effect of molten LiCl-KCl.24 The nanorod structure is supposed to provide more catalytically active sites and facilitate charge and mass transfer during the electrochemical reaction, hence generally showing a higher catalytic activity than amorphous ones.25,26
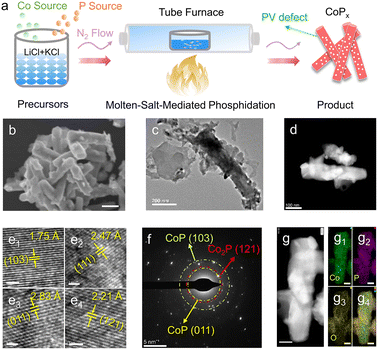 |
| Fig. 1 Synthesis route and structural characterization of MS-CoPx-350. (a) Schematic illustration of the MSMP strategy; (b) SEM graph with a scale bar of 200 nm; (c) TEM image with a scale bar of 200 nm; (d) HAADF-STEM image with a scale bar of 100 nm; (e1–e4) HRTEM images with a scale bar of 1 nm; (f) SAED patterns with a scale bar of 5 nm−1; (g and g1–g4) EDS mappings with a scale bar of 50 nm. | |
To further understand the structural features, transmission electron microscopy (TEM) was carried out to characterize MS-CoPx-350 (Fig. 1c). Small nanoparticles and flakes were observed to adhere on or drift away from the nanorods, which further enhances the exposure of active sites. The high-angle annular dark-field scanning transmission electron microscopy (HAADF-STEM) image of MS-CoPx-350 (Fig. 1d) further shows a nanorod structure. The high resolution TEM (HRTEM) images (Fig. 1e1–e4) show clear lattice fringes. Multiple lattices were exposed: lattice spacings of 1.75 Å, 2.47 Å and 2.83 Å correspond to the CoP's (103), (111) and (011) planes, respectively; a lattice spacing of 2.21 Å is assigned to the Co2P's (121) plane. Selected area electron diffraction (SAED) by TEM (Fig. 1f) reveals several bright rings that arise from CoP (103), CoP (011) and Co2P (121), as marked by green, yellow and red circles, respectively. This agrees well with the HRTEM data. TEM data of the comparison samples are shown in Fig. S3;† evidence for the polycrystalline feature of MS-CoPx-350 is shown in Fig. S4 and S5.† The energy dispersive spectroscopy (EDS) mapping images of MS-CoPx-350 (Fig. 1g and g1–g4) suggest that the dispersions of O, P and Co are even and thereby reveal the actual chemical makeup of CoPx nanorods. By comparing the SEM-EDS results (Fig. S6, S7 and Table S1†), one can note that the CoPx nanorods synthesized in molten salt demonstrate phosphidation to a higher extent. For example, MS-CoPx-350 shows a higher P atomic ratio of 35.76% than CoPx-350 (27.76%). The molten salt medium provides a space confinement effect, which on the one hand promotes the high dispersion of reactant precursors,27 and on the other hand inhibits the fast blowing away of PH3 by a N2 flow. The lower phosphidation of MS-CoPx-400 and MS-CoPx-500 than that of MS-CoPx-350 is because higher temperature led to a faster decomposition of NaH2PO2 and subsequently a faster loss of PH3. Overall, the SEM and TEM data demonstrate the successful preparation of nanorod-like CoPx hybrids with multiple planes’ exposure by the MSMP strategy. Previously, CoPx fabrication required two or more steps, because Co precursors (oxides, hydroxides, MOFs, etc.) need to be first synthesized by the pre-treatment of inorganic Co salts.28 The novel CoPx synthesis strategy presented here demonstrates great progress in simplifying the fabrication process.
Spectroscopy characterization to verify the introduction of PV defects
Fig. 2a compares the X-ray diffraction (XRD) patterns of all CoPx samples. The diffraction peaks at around 31.6°, 35.2°, 36.3°, 46.1°, 48.1°, 52.3°, 55.9°, and 56.8° were indexed to the crystal planes of (011), (200), (111), (112), (211), (103), (020), and (301) of CoP, respectively (Table S2†). The peaks at around 40.7° and 43.1° correspond to the planes of (121) and (211) of Co2P, respectively. These XRD patterns indicate that the synthesized CoPx is polycrystalline, in accord with the HRTEM and SAED data. MS-CoPx-350 shows a better crystallinity than CoPx-350, and this can be explicated by the enhanced phosphidation due to the space confinement of the molten salt medium. Besides CoP and Co2P, the XRD patterns of CoPx-500 and MS-CoPx-500 also show the presence of Co2O3 (220) and Co3O4 (220), suggesting the in situ conversion from CoSO4 to cobalt oxides. From the XRD results, we further reveal the formation mechanism of CoPx by the MSMP strategy: CoSO4 was first converted to cobalt oxides, which then reacted with PH3 to form CoPx. The enhanced phosphidation extent explains the absence of the Co2O3 (220) and Co3O4 (220) planes in MS-CoPx-350. For the Raman spectra (Fig. 2b), the signals within the rectangular region are assigned to CoP;29 the bands centered at around 470, 510, 605 and 660 cm−1 are attributed to cobalt oxides;30,31 the modes at around 1040 cm−1 are consistent with P–O stretching vibrations in a disordered system.32
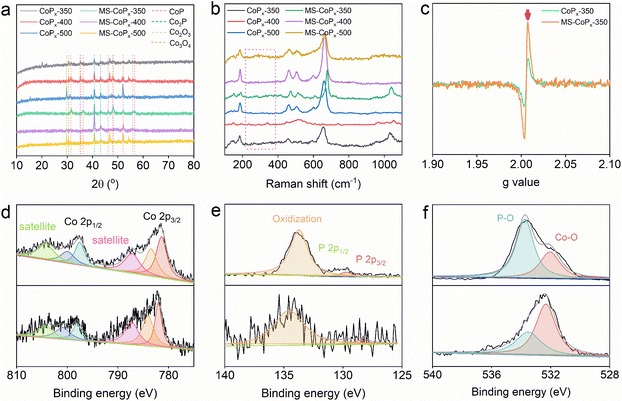 |
| Fig. 2 Spectroscopic characterization of CoPx. (a) XRD patterns; (b) Raman spectra; (c) EPR spectra; (d–f) XPS spectra of MS-CoPx-350 (top) and CoPx-350 (bottom): (d) Co 2p; (e) P 2p and (f) O 1s. The binding energy of XPS spectra was corrected using C 1s 284.6 eV. | |
Electron paramagnetic resonance (EPR) spectroscopy was employed to identify the formation of PV defects (Fig. 2c). The strong signals at g = 2.007 for both CoPx-350 and MS-CoPx-350 indicate the presence of unpaired electrons, which supports the formation of PV.33 We further quantified the PV concentrations by comparing the EPR intensity,34,35 and the results suggest that the PV concentration in MS-CoPx-350 is 2.41 times higher than that in CoPx-350. Akin to the synthetic temperature of 350 °C, MS-CoPx-400 and MS-CoPx-500 have PV concentrations 1.36 and 1.24 times higher than those of CoPx-400 and CoPx-500, respectively (Fig. S8†). These results verified the fact that more PV defects are induced by the molten salt medium. Molten salt generally establishes a robust interaction with the precursor and then provides a strong polarization force.27 In more detail, there is an electronic transmutation between Li (LiCl) and P atoms that obeys the “Zintl-rule”, wherein less electronegative Li donates an electron to more electronegative P, making each P bear a negative charge.36,37 The bonding structure of Li–P tends to weaken Co–P and gives rise to more PV generation. Structural vacancy usually results in electron redistribution and thereby a significant change in the electronic structure.9 Accordingly, X-ray photoelectron spectroscopy (XPS) was applied to reveal the difference in the electronic states between MS-CoPx-350 and CoPx-350 (Fig. 2d–f and Tables S3, S4†). For MS-CoPx-350 (Fig. 2d, top), the Co 2p peaks were deconvoluted into six characteristic peaks within Co 2p3/2 regions at binding energies of 781.39, 783.44, and 787.19 (satellite) eV and Co 2p1/2 regions at binding energies of 797.53, 799.93, and 804.19 (satellite) eV. The peaks centered at 781.39 and 797.53 eV are ascribed to the Co–P bonding of CoPx, while other two peaks at higher binding energies of 783.44 and 799.93 eV are assigned to the oxidized Co species upon contact with air.38 The high-resolution P 2p spectra of MS-CoPx-350 (Fig. 2e, top) can be deconvoluted into two peaks with a doublet centered at 129.73 and 130.36 eV arising from P 2p3/2 and P 2p1/2 of CoPx, respectively. Moreover, a broad peak emerging at 133.72 eV implies the surface oxidation.39 Within the O 1s region of MS-CoPx-350 (Fig. 2f, top), two deconvoluted peaks appeared at 532.05 and 533.74 eV are attributed to the Co–O and P–O species, respectively,40 consistent with the oxidation species formation indicated by Co 2p and P 2p spectra. By comparison to CoPx-350 (Fig. 2d, bottom), we conclude that molten salt induces a visible negative shift in the binding energy (∼0.6–0.9 eV, Table S3†) of the Co 2p spectra. Correspondingly, the P 2p region of MS-CoPx-350 was observed to positively shift when compared to that of CoPx-350 (Fig. 2e, bottom).
This result implies the electron transfer from PV to the Co center. The observed energy shifts also coincide with the EPR results since the unpaired electrons of PV tend to increase the electron density of the Co center. Such modulation of the electronic structure of CoPx is expected to enhance the catalytic performance (see below). XPS characterization of CoPx-400, CoPx-500, MS-CoPx-400, and MS-CoPx-500 (Fig. S9, S10 and Table S3†) further confirmed the increased electron density around the Co center upon introducing the molten salt medium. Altogether, the results strongly supported that the molten salt medium enables defect engineering and modulation of the electronic structure on CoPx.
Electrocatalytic activity under alkaline conditions
The electrocatalytic activity of CoPx towards the HER and OER was evaluated in N2-saturated 1 M KOH solution using a standard three-electrode configuration. Pt/C and IrO2 served as the control for the HER and OER, respectively. As indicated by the HER polarization curves (Fig. 3a), Pt/C shows the highest HER activity with an overpotential of only 22 mV to afford a benchmark current density of 10 mA cm−2. MS-CoPx-350 demonstrates a far lower overpotential (∼190 mV) than CoPx-350 (∼300 mV) at 10 mA cm−2, implying the positive impact of molten salt on the HER activity. This promotion is also observed at other temperatures (MS-CoPx-400, ∼215 mV vs. CoPx-400, ∼243 mV; MS-CoPx-500, ∼273 mV vs. CoPx-500, ∼319 mV) (Fig. S12†). The HER is a multiple-step process and occurs through the Volmer–Heyrovsky or Volmer–Tafel mechanisms.4,8 In the Volmer reaction (adsorption step), a H2O molecule reacts with an electron and generates an adsorbed hydrogen atom (H*) on the electrode surface. Then, the Heyrovsky or Tafel reaction (desorption step) proceeds. Lower H* coverage prefers the Heyrovsky reaction, while higher H* coverage benefits the Tafel reaction. At room temperature, the theoretical Tafel slopes of the Volmer, Heyrovsky and Tafel reactions are 118, 39 and 29 mV dec−1, respectively.4 To explore the HER kinetics and deeply understand the reaction mechanism, the Tafel slope was calculated from the corresponding polarization curves (Fig. 3b). MS-CoPx-350 has a smaller Tafel slope (84 mV dec−1) than CoPx-350 (116 mV dec−1), MS-CoPx-400 (93 mV dec−1) and MS-CoPx-500 (103 mV dec−1), thus revealing a faster HER kinetics. This also indicates that MS-CoPx-350 abides by the Volmer–Heyrovsky HER pathway, wherein the Volmer reaction is the rate-limiting step.41 The superior HER activity of MS-CoPx-350 is further supported by the electrochemical impedance spectra (EIS) (Fig. 3c and Table S5†). MS-CoPx-350 exhibits a reduced charge transfer resistance (Rct) of 187.5 Ω compared with CoPx-350 (436.8 Ω). Lower Rct is beneficial for the combination between H* and electrons, which promotes the HER reaction.42,43 In catalysis, the electrochemical active surface area (ECSA) is proportional to electrochemical double-layer capacitance (Cdl).44 Therefore, Cdl (Fig. S13†) was calculated from the cyclic voltammograms measured with varying scanning rates (Fig. S14†) to evaluate the ECSA. The results show that MS-CoPx-350 has the highest Cdl value of 21.9 mF cm−2, which is roughly 5, 3, and 4 times greater than those of CoPx-350 (4.8 mF cm−2), MS-CoPx-400 (7.5 mF cm−2), and MS-CoPx-500 (5.5 mF cm−2), respectively. This suggests that MS-CoPx-350 can provide more active sites during the reaction process and agrees well with the lowest overpotential it required to reach 10 mA cm−2. All the above results underscore the key role of molten salt in improving the CoPx's HER activity. The HER performance of MS-CoPx-350 is comparable to those of the state-of-the-art CoP- and Co2P-based electrocatalysts (Table S8†). The HER activity of CoPx was also evaluated in 0.5 M H2SO4 (Fig. S15, S16 and Table S6†), and MS-CoPx-350 surpassed other samples owing to its highest phosphidation extent. The excellent HER activity of MS-CoPx-350 under both alkaline and acidic conditions reveals its potential to serve as a pH-universal cathodic electrocatalyst to catalyze water splitting.
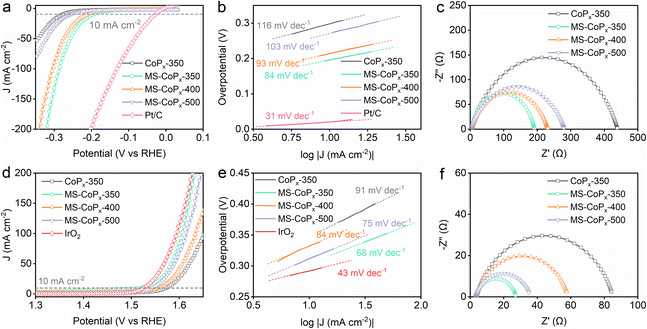 |
| Fig. 3 Electrochemical characterization of CoPx towards the HER and OER in 1 M KOH. (a–c) belong to the HER. (a) Polarization curves; (b) Tafel slopes calculated from the corresponding polarization curves; (c) EIS spectra obtained with an overpotential of 100 mV and fitted with the equivalent circuit models shown in Fig. S17.† (d–f) belong to the OER. (d) Polarization curves of CoPx; (e) Tafel slopes calculated from the corresponding polarization curves; (f) EIS spectra obtained with an overpotential of 300 mV. The HER and OER polarization curves were acquired with a scan rate of 5 mV s−1 (80% IR). | |
For OER measurements, MS-CoPx-350 shows a similar electrocatalytic activity (∼300 mV overpotential) to the control of IrO2 (∼290 mV overpotential) at 10 mA cm−2, which is obviously higher than that of CoPx-350 (∼351 mV overpotential) (Fig. 3d). With the assistance of molten salt, the catalysts synthesized at other temperatures also have lower overpotentials of ∼334 mV (MS-CoPx-400) and ∼310 mV (MS-CoPx-500) than CoPx-350 without molten salt. The corresponding Tafel plots (Fig. 3e) show the smallest slope of 68 mV dec−1 over MS-CoPx-350 among all samples (CoPx-350, 91 mV dec−1; MS-CoPx-400, 84 mV dec−1; MS-CoPx-500, 75 mV dec−1), suggesting its highest OER kinetics. MS-CoPx-350 also has the lowest Rct value (24.19 Ω) compared to CoPx-350 (81.36 Ω), MS-CoPx-400 (54.83 Ω) and MS-CoPx-500 (32.5 Ω) under the same overpotential of 300 mV (Fig. 3f and Table S7†). All results suggest that the synthesis within the molten salt medium enhanced the alkaline OER activity of CoPx. The increase of the molten salt temperature to 400 and 500 °C did not improve the OER activity very obviously (Fig. S12†), corresponding to the reduction of phosphidation at these higher temperatures (Tables S1 and S4†), which further proves that both the HER and OER are dependent on the phosphidation extent. Notably, the as-prepared MS-CoPx-350 surpasses most of the reported OER catalysts and locates among the most efficient ones (Table S8†).
In addition to CoPx, we further extended the reported molten salt strategy to the synthesis of other TMPs. XRD characterization (Fig. S18†) suggests the successful preparation of NiPx including NiP, Ni2P, and NiP2. However, besides Fe2P, there are a lot of impurities in the synthesized FePx compounds such as NaH2PO4, Fe3(PO4)2 and some ferric oxides, indicating the insufficient phosphidation and by-product generation.
Electrochemical measurements of NiPx and FePx were carried out under the same conditions as those for CoPx (Fig. S19 and Table S9†). MS-NiPx-350 shows overpotentials of 220 and 280 mV towards the HER and OER, respectively, which are similar to those of MS-CoPx-350. By comparison, due to the impurities and insufficient phosphidation, MS-FePx-350 shows much poorer catalytic activities towards the HER (414 mV) and OER (440 mV) when compared to MS-NiPx-350. Although we did not obtain pure FePx here, the presence of Fe2P also supports the occurrence of the phosphidation reaction with molten salt. From the FePx case, we further confirm that more research efforts are needed in improving the purity of specific TMPs or even controlling the crystal exposure.
Overall water splitting under alkaline conditions
Given the prominent catalytic activities of MS-CoPx-350 towards both the HER and OER under alkaline conditions, a two-electrode electrolyzer (MS-CoPx-350||MS-CoPx-350) with MS-CoPx-350 serving as both cathodic and anodic catalysts was assembled to carry out overall water splitting in 1 M KOH solution (Fig. 4). Fig. 4a demonstrates the dependence of current generation on applied cell voltage within the indicated electrolyzer, and it shows that ∼1.75 V produces ∼10 mA cm−2. This result coincides well with the HER and OER polarization curves over MS-CoPx-350 (Fig. 4a, inset). The potentiostatic electrolysis examined with varying cell voltages (Fig. 4b) shows that the current density is positively correlated with the applied voltage; in detail, 1.75 V, 1.85 V, and 1.90 V produce current densities of ∼10, ∼50, and ∼100 mA cm−2, respectively. The durability was examined by the chronoamperometry test at 100 mA cm−2 for 24 hours (Fig. 4c). The cell maintained a stable voltage with a negligible increase (<10%) subsequent to 24-hour electrolysis, suggesting that MS-CoPx-350 has an impressive stability. A digital graph of the electrolyzer (1.75 V) shows the generation of H2 and O2 bubbles on the electrodes’ surface (Fig. 4d). CoPx subsequent to 24-hour water electrolysis was further characterized by XPS to explore the changes in catalyst's surface chemistry (Fig. S21 and Table S10†). Both the post-HER and post-OER samples show similar chemical compositions to pristine CoPx, which further verifies the excellent stability. The Co 2p spectra of post-HER and post-OER CoPx experienced a slight negative shift, while the P 2p spectra did not show any visible shift, indicating that Co cite may serve as the active site. Post-HER MS-CoPx-350 was further characterized by XRD and SEM. The good consistence in the XRD patterns (Fig. S22, inset†) indicates that MS-CoPx-350 was not decomposed during the long-term reaction. The SEM images of post-HER MS-CoPx-350 (Fig. S23a and b†) prove that the catalyst maintains its originally rod structure basically. The above results agree well with the excellent catalytic stability. In summary, MS-CoPx-350 is capable of catalyzing the overall water splitting with the catalytic performance comparable to those of other noble-metal-free catalysts (Table S11†), and moreover, it can be easily manufactured.
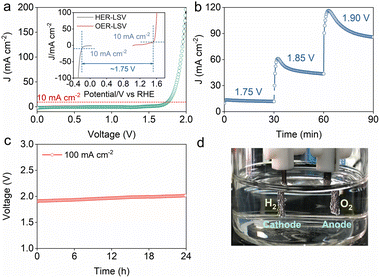 |
| Fig. 4 Overall water splitting by the MS-CoPx-350||MS-CoPx-350 electrolyzer in 1 M KOH solution. (a) Polarization curve of the electrolyzer for overall water splitting, and inset: polarization curves of the HER and OER over MS-CoPx-350; (b) potentiostatic electrolysis by the electrolyzer with varying cell voltages; (c) 24-hour chronopotentiometry test of the electrolyzer at 100 mA cm−2; (d) digital graph of the electrolyzer at 1.75 V cell voltage. | |
DFT study to reveal the promotion mechanism of PV towards electrocatalytic activity
Density functional theory (DFT) calculations were conducted to explore the underlying promotion mechanisms of PV defects towards catalytic activity. CoP (103) and Co2P (121) were selected to model CoP and Co2P, respectively, because, (i) their presence was confirmed by both XRD and TEM; and (ii) they appeared in all samples. The constructed slab models for CoP and Co2P are depicted in Fig. S25.† We firstly evaluated the adsorption behavior of H2O over P and Co sites by calculating the Gibbs free energy diagram (ΔGH2O) (Fig. 5a). The computational results show that the Co sites of CoP and Co2P give exothermic ΔGH2O values of −0.555 and −0.809 eV, respectively, which are more negative than the P sites of CoP (−0.125 eV) and Co2P (−0.179 eV), indicating a better affinity between Co and H2O. Therefore, Co was suggested to be the active site for both CoP and Co2P. This is in line with the post-electrolysis samples’ XPS characterization (Fig. S21 and Table S10†), where the Co 2p region experiences a visibly negative shift for both the post-HER and post-OER samples, but no obvious change is observed in the P 2p spectra.
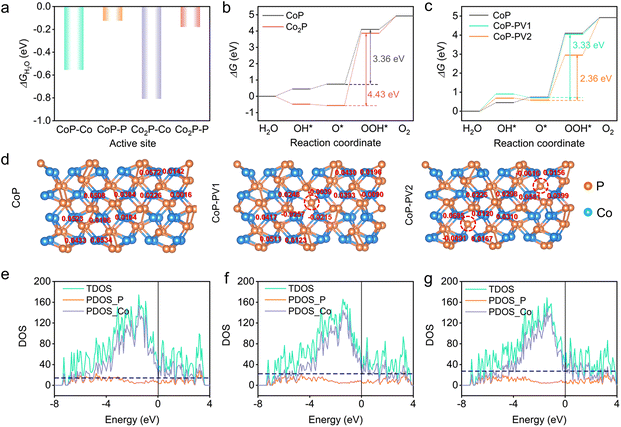 |
| Fig. 5 DFT calculations. (a) Adsorption energy of H2O on CoP and Co2P; (b) free energy diagram of the OER on CoP and Co2P; (c) free energy diagram of the OER on CoP, CoP-PV1 and CoP-PV2; (d) Bader charge states of CoP, CoP-PV1, and CoP-PV2; DOS plots of (e) CoP, (f) CoP-PV1, and (g) CoP-PV2, wherein the Fermi level was set to zero. | |
It is known that the OER is a more challenging half reaction than the HER because of its sluggish kinetics and complex proton-coupled multielectron transfer process.45 Therefore, the OER was employed as the model reaction for DFT calculations. The OER typically consists of four elementary steps under alkaline conditions,46,47 and we calculated the Gibbs free energy of all intermediates (OH*, O* and OOH*) (Fig. S26 and S27†), yielding the theoretical overpotential of the OER over CoP and Co2P (Fig. 5b), respectively. The rate-determining step (RDS) is the formation of OOH* through O* reacting with OH− for both CoP and Co2P, with a ΔGRDS value of 3.36 and 4.43 eV, respectively. Hence, the OER will proceed more favourably on CoP due to its far lower energy barrier. Next, we constructed slab models for CoP with 1 (CoP-PV1) and 2 (CoP-PV2) PV to simulate different PV concentrations (Fig. S28†). The Gibbs free energy diagrams (Fig. 5c) show that CoP-PV2 shows optimal OER performances (ΔGRDS = 2.36 eV) compared to CoP-PV1 (ΔGRDS = 3.33 eV) and CoP (ΔGRDS = 3.36 eV). This result suggests that the formation of more PV is beneficial for enhancing the catalytic activity, accounting for the experimental observations that CoP with more PV defects shows boosted catalytic activity.
The Bader charge was examined to understand the above phenomenon. Upon introduction of PV defects, the electron transfer from PV to nearby Co occurs, as evidenced by the reduced Bader charge of the Co sites (Fig. 5d). This result agrees well with the XPS results, where we can see an obvious negative shift in the Co 2p region when comparing MS-CoPx-350 to CoPx-350. More negative Co sites will promote the dissociation of the H–O bond in OH*, and then facilitate the following generation of O* and OOH*, thus leading to a visibly reduced energy barrier.48 To further reveal the electronic states maintained by CoP, CoP-PV1 and CoP-PV2, partial density of states (PDOS) and total density of states (TDOS) were calculated (Fig. 5e–g). DOS plots show that the conducting charges primarily come from the Co 3d electronic states, as implied by the nearly matched curves of PDOS_Co with those of TDOS. The electronic states close to the Fermi level (set to zero) obey the following order: CoP-PV2 > CoP-PV1 > CoP, indicating that more charge carriers can be introduced via increasing the PV concentration46 and coinciding with the smaller charge transfer resistance of MS-CoPx-350 than that of CoPx-350 (EIS results). The above discussions strongly support that the PV defects benefit the optimization of the electronic structure, and the enhancement of the electronic conductivity, thereby leading to an improvement in the electrochemical activity.
4. Conclusions
This study provides a one-step molten salt strategy to synthesize and simultaneously carry out PV defect engineering over CoPx (CoP/Co2P). In molten salt medium, inorganic Co salt was first converted into cobalt oxides, which then reacted with PH3 generated by thermal decomposition of NaH2PO2 to produce CoPx. Under alkaline conditions, the assembled two-electrode electrolyzer with the bifunctional electrocatalyst CoPx only needed 1.75 and 1.90 V to drive current densities of 10 and 100 mA cm−2 for overall water splitting, respectively. The performance decay was negligible after 24-hour continuous operation, indicating the excellent stability of CoPx. DFT calculations suggested that increasing the PV concentration, induced by molten salt medium, delivered more negatively charged Co sites and more charge carriers, which were beneficial for reducing the energy barriers of reaction intermediates’ formation and enhancing the electronic conductivity, respectively. The successful preparation of NiPx and Fe2P by the molten salt strategy proves that this methodology can be further extended to the synthesis of other mono-, or even bi- and tri-metal TMPs. Moreover, the presented molten salt strategy offers an opportunity to gain more insights into TMPs’ defect engineering and electronic structure manipulation.
Author contributions
Z. L. and L. L. conceived the experiments and wrote the manuscript. Z. L., C. Z., C. W and B. Z. conducted the molten-salt-mediated synthesis and electrochemical characterization of the electrocatalyst. Y. Yang, S. P., Y. Yu and W. C. conducted the TEM, SEM, XRD, XPS, Raman and EPR spectra measurements. Z. L. did the DFT calculations.
Conflicts of interest
There are no conflicts to declare.
Acknowledgements
This work was financially supported by the National Natural Science Foundation of China (22176046), the State Key Laboratory of Urban Water Resource and Environment (Harbin Institute of Technology) (2021TS13), the Shenzhen Science and Technology Program (KQTD20190929172630447 and JCYJ20210324124209025), the Natural Science Foundation of Guangdong Province (2022A1515012016), and the China Postdoctoral Science Foundation (2021M700997).
References
- R. d'Amore-Domenech, Ó. Santiago and T. J. Leo, Multicriteria analysis of seawater electrolysis technologies for green hydrogen production at sea, Renewable Sustainable Energy Rev., 2020, 133, 110166 CrossRef.
- M. Yu, E. Budiyanto and H. Tüysüz, Principles of Water Electrolysis and Recent Progress in Cobalt-, Nickel-, and Iron-Based Oxides for the Oxygen Evolution Reaction, Angew. Chem., Int. Ed., 2022, 61, e202103824 CAS.
- P. Yu, F. Wang, T. A. Shifa, X. Zhan, X. Lou, F. Xia and J. He, Earth abundant materials beyond transition metal dichalcogenides: A focus on electrocatalyzing hydrogen evolution reaction, Nano Energy, 2019, 58, 244–276 CrossRef CAS.
- Y. Shi and B. Zhang, Recent advances in transition metal phosphide nanomaterials: synthesis and applications in hydrogen evolution reaction, Chem. Soc. Rev., 2016, 45, 1529–1541 RSC.
- Y. Shi, M. Li, Y. Yu and B. Zhang, Recent advances in nanostructured transition metal phosphides: synthesis and energy-related applications, Energy Environ. Sci., 2020, 13, 4564–4582 RSC.
- J. Xu, J. Li, D. Xiong, B. Zhang, Y. Liu, K. H. Wu, I. Amorim, W. Li and L. Liu, Trends in activity for the oxygen evolution reaction on transition metal (M=Fe, Co, Ni) phosphide pre-catalysts, Chem. Sci., 2018, 9, 3470–3476 RSC.
- J. Xu, Y. Liu, J. Li, I. Amorim, B. Zhang, D. Xiong, N. Zhang, S. M. Thalluri, J. P. S. Sousa and L. Liu, Hollow cobalt phosphide octahedral pre-catalysts with exceptionally high intrinsic catalytic activity for electro-oxidation of water and methanol, J. Mater. Chem. A, 2018, 6, 20646–20652 RSC.
- J. Zhu, L. Hu, P. Zhao, L. Y. S. Lee and K.-Y. Wong, Recent Advances in Electrocatalytic Hydrogen Evolution Using Nanoparticles, Chem. Rev., 2020, 120, 851–918 CrossRef CAS PubMed.
- Y. Wu, W. Xu, L. Jiao, Y. Tang, Y. Chen, W. Gu and C. Zhu, Defect engineering in nanozymes, Mater. Today, 2021, 52, 327–347 CrossRef.
- F. Cheng, T. Zhang, Y. Zhang, J. Du, X. Han and J. Chen, Enhancing Electrocatalytic Oxygen Reduction on MnO2 with Vacancies, Angew. Chem., Int. Ed., 2013, 52, 2474–2477 CrossRef CAS PubMed.
- J. Kim, X. Yin, K.-C. Tsao, S. Fang and H. Yang, Ca2Mn2O5 as Oxygen-Deficient Perovskite Electrocatalyst for Oxygen Evolution Reaction, J. Am. Chem. Soc., 2014, 136, 14646–14649 CrossRef CAS.
- H. Li, C. Tsai, A. L. Koh, L. Cai, A. W. Contryman, A. H. Fragapane, J. Zhao, H. S. Han, H. C. Manoharan, F. Abild-Pedersen, J. K. Nørskov and X. Zheng, Activating and optimizing MoS2 basal planes for hydrogen evolution through the formation of strained sulphur vacancies, Nat. Mater., 2016, 15, 48–53 CrossRef CAS.
- Y. Yin, J. Han, Y. Zhang, X. Zhang, P. Xu, Q. Yuan, L. Samad, X. Wang, Y. Wang, Z. Zhang, P. Zhang, X. Cao, B. Song and S. Jin, Contributions of Phase, Sulfur Vacancies, and Edges to the Hydrogen Evolution Reaction Catalytic Activity of Porous Molybdenum Disulfide Nanosheets, J. Am. Chem. Soc., 2016, 138, 7965–7972 CrossRef CAS.
- Y. Jia, L. Zhang, A. Du, G. Gao, J. Chen, X. Yan, C. L. Brown and X. Yao, Defect Graphene as a Trifunctional Catalyst for Electrochemical Reactions, Adv. Mater., 2016, 28, 9532–9538 CrossRef CAS PubMed.
- C. Xie, D. Yan, W. Chen, Y. Zou, R. Chen, S. Zang, Y. Wang, X. Yao and S. Wang, Insight into the design of defect electrocatalysts: From electronic structure to adsorption energy, Mater. Today, 2019, 31, 47–68 CrossRef CAS.
- R. Sun, Y. Bai, Z. Bai, L. Peng, M. Luo, M. Qu, Y. Gao, Z. Wang, W. Sun and K. Sun, Phosphorus Vacancies as Effective Polysulfide Promoter for High-Energy-Density Lithium–Sulfur Batteries, Adv. Energy Mater., 2022, 12, 2102739 CrossRef CAS.
- S. Li, Z. Geng, X. Wang, X. Ren, J. Liu, X. Hou, Y. Sun, W. Zhang, K. Huang and S. Feng, Optimizing the surface state of cobalt-iron bimetallic phosphide via regulating phosphorus vacancies, Chem. Commun., 2020, 56, 2602–2605 RSC.
- M. Jin, X. Zhang, M. Han, H. Wang, G. Wang and H. Zhang, Efficient electrochemical N2 fixation by doped-oxygen-induced phosphorus vacancy defects on copper phosphide nanosheets, J. Mater. Chem. A, 2020, 8, 5936–5942 RSC.
- V. M. B. Nunes, C. S. Queirós, M. J. V. Lourenço, F. J. V. Santos and C. A. Nieto de Castro, Molten salts as engineering fluids – A review: Part I. Molten alkali nitrates, Appl. Energy, 2016, 183, 603–611 CrossRef CAS.
- M. Xiao, L. Zhang, B. Luo, M. Lyu, Z. Wang, H. Huang, S. Wang, A. Du and L. Wang, Molten-Salt-Mediated Synthesis of an Atomic Nickel Co-catalyst on TiO2 for Improved Photocatalytic H2 Evolution, Angew. Chem., Int. Ed., 2020, 59, 7230–7234 CrossRef CAS PubMed.
- M. Xiao, B. Luo, M. Konarova, Z. Wang and L. Wang, Molten Salt Synthesis of Atomic Heterogeneous Catalysts: Old Chemistry for Advanced Materials, Eur. J. Inorg. Chem., 2020, 2020, 2942–2949 CrossRef CAS.
- X. Liu, N. Fechler and M. Antonietti, Salt melt synthesis of ceramics, semiconductors and carbon nanostructures, Chem. Soc. Rev., 2013, 42, 8237–8265 RSC.
- Y. Bai, C. Liu, T. Chen, W. Li, S. Zheng, Y. Pi, Y. Luo and H. Pang, MXene-Copper/Cobalt Hybrids via Lewis Acidic Molten Salts Etching for High Performance Symmetric Supercapacitors, Angew. Chem., Int. Ed., 2021, 60, 25318–25322 CrossRef CAS.
- H. Cui, M. Jiao, Y.-N. Chen, Y. Guo, L. Yang, Z. Xie, Z. Zhou and S. Guo, Molten-Salt-Assisted Synthesis of 3D Holey N-Doped Graphene as Bifunctional Electrocatalysts for Rechargeable Zn–Air Batteries, Small Methods, 2018, 2, 1800144 CrossRef.
- B. Zhou, J. Li, X. Zhang and J. Guo, Engineering P-doped Ni3S2-NiS hybrid nanorod arrays for efficient overall water electrolysis, J. Alloys Compd., 2021, 862, 158391 CrossRef CAS.
- L. Zhang, H. Li, B. Yang, N. Han, Y. Wang, Z. Zhang, Y. Zhou, D. Chen and Y. Gao, Promote the electrocatalysis activity of amorphous FeOOH to oxygen evolution reaction by coupling with ZnO nanorod array, J. Solid State Electrochem., 2020, 24, 905–914 CrossRef CAS.
- N. Li, W. Liu, C. Zhu, C. Hao, J. Guo, H. Jing, J. Hu, C. Xin, D. Wu and Y. Shi, Molten salt as ultrastrong polar solvent enables the most straightforward pyrolysis towards highly efficient and stable single-atom electrocatalyst, J. Energy Chem., 2021, 54, 519–527 CrossRef CAS.
- J. Wang, Z. Liu, Y. Zheng, L. Cui, W. Yang and J. Liu, Recent advances in cobalt phosphide based materials for energy-related applications, J. Mater. Chem. A, 2017, 5, 22913–22932 RSC.
- L. Su, X. Cui, T. He, L. Zeng, H. Tian, Y. Song, K. Qi and B. Y. Xia, Surface reconstruction of cobalt phosphide nanosheets by electrochemical activation for enhanced hydrogen evolution in alkaline solution, Chem. Sci., 2019, 10, 2019–2024 RSC.
- J. Tyczkowski, R. Kapica and J. Łojewska, Thin cobalt oxide films for catalysis deposited by plasma-enhanced metal–organic chemical vapor deposition, Thin Solid Films, 2007, 515, 6590–6595 CrossRef CAS.
- V. G. Hadjiev, M. N. Iliev and I. V. Vergilov, The Raman spectra of Co3O4, J. Phys. C: Solid State Phys., 1988, 21, L199–L201 CrossRef.
- F. H. Saadi, A. I. Carim, W. S. Drisdell, S. Gul, J. H. Baricuatro, J. Yano, M. P. Soriaga and N. S. Lewis, Operando Spectroscopic Analysis of CoP Films Electrocatalyzing the Hydrogen-Evolution Reaction, J. Am. Chem. Soc., 2017, 139, 12927–12930 CrossRef CAS PubMed.
- G. Yuan, J. Bai, L. Zhang, X. Chen and L. Ren, The effect of P vacancies on the activity of cobalt phosphide nanorods as oxygen evolution electrocatalyst in alkali, Appl. Catal., B, 2021, 284, 119693 CrossRef CAS.
- S. Nadupalli, S. Repp, S. Weber and E. Erdem, About defect phenomena in ZnO nanocrystals, Nanoscale, 2021, 13, 9160–9171 RSC.
- S. Najib, F. Bakan, N. Abdullayeva, R. Bahariqushchi, S. Kasap, G. Franzo, M. Sankir, N. Demirci Sankir, S. Mirabella and E. Erdem, Tailoring morphology to control defect structures in ZnO electrodes for high-performance supercapacitor devices, Nanoscale, 2020, 12, 16162–16172 RSC.
- J. K. Olson and A. I. Boldyrev, Electronic transmutation: Boron acquiring an extra electron becomes ‘carbon’, Chem. Phys. Lett., 2012, 523, 83–86 CrossRef CAS.
- A. S. Ivanov, A. J. Morris, K. V. Bozhenko, C. J. Pickard and A. I. Boldyrev, Inorganic Double-Helix Structures of Unusually Simple Lithium–Phosphorus Species, Angew. Chem., Int. Ed., 2012, 51, 8330–8333 CrossRef CAS.
- L. Yang, R. Liu and L. Jiao, Electronic Redistribution: Construction and Modulation of Interface Engineering on CoP for Enhancing Overall Water Splitting, Adv. Funct. Mater., 2020, 30, 1909618 CrossRef CAS.
- X. Huang, X. Xu, C. Li, D. Wu, D. Cheng and D. Cao, Vertical CoP Nanoarray Wrapped by N,P-Doped Carbon for Hydrogen Evolution Reaction in Both Acidic and Alkaline Conditions, Adv. Energy Mater., 2019, 9, 1803970 CrossRef.
- Y. Ma, G. Zhou, Z. Liu, L. Xu, D. Sun and Y. Tang, Electronic structural regulation of CoP nanorods by the tunable incorporation of oxygen for enhanced electrocatalytic activity during the hydrogen evolution reaction, Nanoscale, 2020, 12, 14733–14738 RSC.
- X. Xiao, C. Engelbrekt, Z. Li and P. Si, Hydrogen evolution at nanoporous gold/tungsten sulfide composite film and its optimization, Electrochim. Acta, 2015, 173, 393–398 CrossRef CAS.
- M. A. Lukowski, A. S. Daniel, F. Meng, A. Forticaux, L. Li and S. Jin, Enhanced Hydrogen Evolution Catalysis from Chemically Exfoliated Metallic MoS2 Nanosheets, J. Am. Chem. Soc., 2013, 135, 10274–10277 CrossRef CAS PubMed.
- Y. Cao, H. Liu, X. Bo and F. Wang, Highly active porous nickel-film electrode via polystyrene microsphere template-assisted composite electrodeposition for hydrogen-evolution reaction in alkaline medium, Sci. China: Chem., 2015, 58, 501–507 CrossRef CAS.
- Y. Huang, Y. Sun, X. Zheng, T. Aoki, B. Pattengale, J. Huang, X. He, W. Bian, S. Younan, N. Williams, J. Hu, J. Ge, N. Pu, X. Yan, X. Pan, L. Zhang, Y. Wei and J. Gu, Atomically engineering activation sites onto metallic 1T-MoS2 catalysts for enhanced electrochemical hydrogen evolution, Nat. Commun., 2019, 10, 982 CrossRef CAS.
- K. Wang, X. Wang, Z. Li, B. Yang, M. Ling, X. Gao, J. Lu, Q. Shi, L. Lei, G. Wu and Y. Hou, Designing 3d dual transition metal electrocatalysts for oxygen evolution reaction in alkaline electrolyte: Beyond oxides, Nano Energy, 2020, 77, 105162 CrossRef CAS.
- H. Xue, A. Meng, H. Zhang, Y. Lin, Z. Li and C. Wang, 3D urchin like V-doped CoP in situ grown on nickel foam as bifunctional electrocatalyst for efficient overall water-splitting, Nano Res., 2021, 14, 4173–4181 CrossRef CAS.
- J. Jiang, F. Sun, S. Zhou, W. Hu, H. Zhang, J. Dong, Z. Jiang, J. Zhao, J. Li, W. Yan and M. Wang, Atomic-level insight into super-efficient electrocatalytic oxygen evolution on iron and vanadium co-doped nickel (oxy)hydroxide, Nat. Commun., 2018, 9, 2885 CrossRef.
- C. Guan, W. Xiao, H. Wu, X. Liu, W. Zang, H. Zhang, J. Ding, Y. P. Feng, S. J. Pennycook and J. Wang, Hollow Mo-doped CoP nanoarrays for efficient overall water splitting, Nano Energy, 2018, 48, 73–80 CrossRef.
Footnote |
† Electronic supplementary information (ESI) available: Experimental details (spectroscopy and structural characterization, electrode preparation, electrochemical measurements, and DFT calculations), XPS, SEM-EDS, TEM, EPR, electrochemical results, and catalytic activity table (comparison with other available CoP electrocatalysts). See DOI: https://doi.org/10.1039/d2qi01902g |
|
This journal is © the Partner Organisations 2023 |
Click here to see how this site uses Cookies. View our privacy policy here.