DOI:
10.1039/D2QI02757G
(Research Article)
Inorg. Chem. Front., 2023,
10, 2014-2021
Rare-earth La-doped VS2−x for electrochemical nitrate reduction to ammonia†
Received
29th December 2022
, Accepted 13th February 2023
First published on 14th February 2023
Abstract
We report rare-earth La-doped VS2−x enriched with S-vacancies (La-VS2−x) towards the electrochemical nitrate reduction to ammonia (NO3RR), which shows a maximum NH3-faradaic efficiency of 96.6% with a corresponding NH3 yield rate of 11.3 mg h−1 cm−2 at −0.6 V vs. RHE. Theoretical computations unveil that La-dopants and S-vacancies synergistically promote NO3− activation, suppress hydrogen evolution and lower the energetic barriers, leading to the enhanced NO3RR activity and selectivity of La-VS2−x.
1. Introduction
NH3, a value-added chemical and also a renewable hydrogen-rich carrier, is vital to modern agriculture and industry.1–3 Electrochemical nitrate reduction to ammonia (NO3RR) represents a fascinating approach to realising both green NH3 generation and wastewater purification.4–6 However, NO3RR effectiveness is still retarded by the complex multi-electron transfer process and easy occurrence of side reactions especially competing for the hydrogen evolution reaction (HER).7–9 Therefore, it is urgently required to explore effective NO3RR electrocatalysts for active and selective NO3−-to-NH3 conversion.10–14
To date, substantial efforts have been devoted to exploring many potential NO3RR catalysts.15–25 Metal chalcogenides have attracted wide attention in electrolysis due to their layered structure and high electrochemical stability.26–28 Among them, VS2 is most appealing due to its metallic nature with high conductivity, facilitating accelerated electron transfer for boosting the catalytic kinetics.29,30 Nevertheless, investigations on VS2-based catalysts for the NO3RR remain largely unexplored due presumably to the poor intrinsic activity of VS2 for NO3− activation. Metal doping is a promising approach to tuning the surface electronic structure of the catalysts to significantly enhance the catalytic activity.31 By virtue of the unique 4f structure and rich redox capability, rare-earth lanthanides emerge as intriguing metal dopants to considerably improve the catalyst activities.32–34 Nevertheless, the use of rare-earth metal dopants to tune the electronic structure and NO3RR activity of the catalysts has not yet been explored.
In this study, we report a rare-earth La-doped VS2−x (La-VS2−x) enriched with S-vacancies (VS) towards the NO3RR. La-VS2−x delivers excellent NO3RR performance with a highest NH3-faradaic efficiency (FENH3) of 96.6% with the corresponding NH3 yield of 11.3 mg h−1 cm−2 at −0.6 V vs. RHE. Detailed experiments combined with theoretical investigations are employed to unravel the catalytic NO3RR mechanism of La-VS2−x.
2. Results and discussion
La-VS2−x (4.8 wt% La) grown on carbon cloth (CC) was fabricated using a simple hydrothermal approach. The XRD pattern of La-VS2−x (Fig. 1a) shows major diffraction peaks assigned to the hexagonal VS2 phase (JCPDS No. 89-1640). The SEM image of La-VS2−x (Fig. 1b) shows numerous nanosheets which grow perpendicularly on the CC substrate, and the nanosheet morphology of La-VS2−x is further verified by the TEM image (Fig. 1c). The elemental mapping images of La-VS2−x (Fig. 1d) reveal a uniform distribution of La elements. The HRTEM image of La-VS2−x (Fig. 1e) shows two d spacings of 0.25 and 0.60 nm, corresponding to the (011) and (001) facets of VS2, respectively. The corresponding inverse fast Fourier transform (IFFT) pattern (Fig. 1f) and lattice line scanning analysis (Fig. 1g) signify the loss of some lattice atoms (dotted circles), indicating the presence of abundant defects/vacancies on La-VS2−x.35 The elemental analysis further reveals a much reduced S/V molar ratio of 1.85 compared to the nominal ratio of VS2 (2), demonstrating the VS-rich nature of La-VS2−x.36
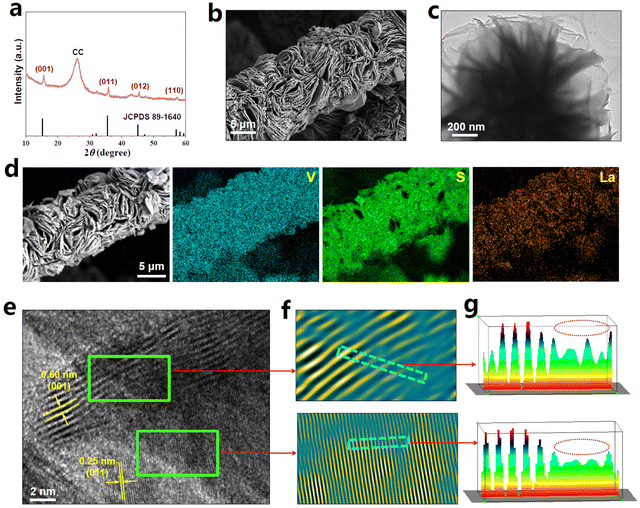 |
| Fig. 1 (a) XRD pattern of La-VS2−x on CC. (b) SEM image of La-VS2−x on CC. (c) TEM image of La-VS2−x. (d) Elemental mapping images of La-VS2−x on CC. (e) HRTEM image of La-VS2−x and (f) the corresponding IFFT pattern and (g) lattice line scanning analyses. | |
As shown in the electron paramagnetic resonance (EPR) spectrum (Fig. 2a), La-VS2−x displays a much enhanced EPR signal compared to pristine VS2, further attesting to the existence of abundant VS on La-VS2−x.36–39 The X-ray absorption near-edge structure (XANES) spectra (Fig. 2b) show that the white line intensity of La-VS2−x is lower than that of the La2O3 reference, suggesting that La-dopants carry a partially positive charge.40 The extended X-ray absorption fine structure (EXAFS) spectra (Fig. 2c) show that La-VS2−x presents a dominant peak at 2.16 Å, assigned to the La–S scattering path. Besides, no La–La (3.96 Å) scattering paths can be detected, confirming that La-dopants are atomically dispersed in La-VS2−x. The evidence for the presence of atomically dispersed La-dopants in La-VS2−x can be further proved by the wavelet transform (WT) plots (Fig. 2d), showing the absence of a La–La signal in La-VS2−x.41–43 The EXAFS fitting results (Fig. 2e, Table S1†) reveal that the La–S coordination is around 5, implying that La-dopants mainly substitute the five-fold coordinated V atoms of VS2−x (Fig. 2f).
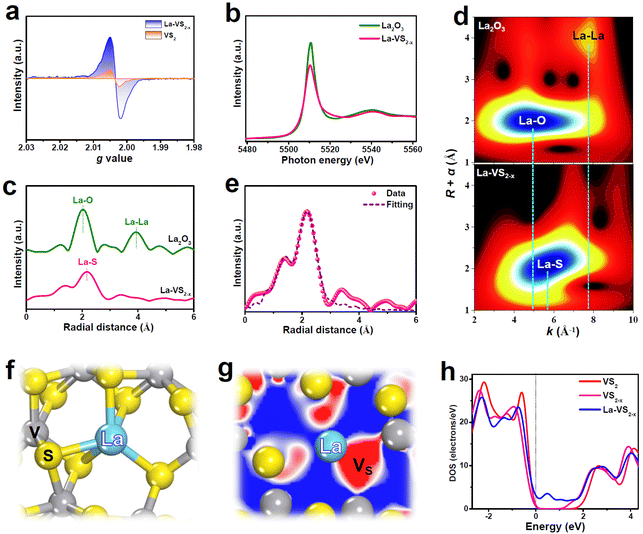 |
| Fig. 2 (a) EPR spectra of VS2 and La-VS2−x. (b) La L3-edge XANES, (c) EXAFS spectra and (d) WT profiles of La-VS2−x and reference La2O3. (e) EXAFS fitting curve of La-VS2−x and (f) the corresponding fitting model. (g) Electron contour map of La-VS2−x (red: charge accumulation, blue: charge depletion). (h) DOS plots of VS2, VS2−x and La-VS2−x. | |
Theoretical calculations are carried out to examine the electronic structure of La-VS2−x. The calculated La-dopant formation energy (ELa, Fig. S1†) reveals a much reduced ELa of La-VS2−x (1.94 eV) compared to VS-free La-VS2 (3.61 eV), suggesting that VS plays a critical role in making the incorporation of La-dopants into VS2−x lattices more thermodynamically feasible.43,44 Electron contour maps reveal abundant electrons accumulated in the VS region (Fig. S2 and S3†), while La-dopant affects little the electron accumulation nature of VS in La-VS2−x (Fig. 2g). These accumulated electrons are apt to be transferred to the antibonding orbital of NO3− for the activation and dissociation of the N
O bond,5,45 facilitating the boosted NO3RR process. The partial densities of states (PDOS) plot of La-VS2−x (Fig. S4†) reveals a significant La/S orbital hybridization, suggesting the strong La–S electronic interactions which allow La-dopants to be atomically dispersed and firmly stabilized in VS2−x, resulting in the high thermodynamic stability of La-VS2−x (Fig. S5†).46 Furthermore, compared to VS2 and VS2−x, La-dopant incorporation makes La-VS2−x exhibit the occupied electron states across the Fermi level (Fig. 2h) and reduced work function (Fig. S6†), thus endowing La-VS2−x with enhanced conductivity to accelerate electron transfer and catalytic kinetics (Fig. S7†).47–51
The electrochemical NO3RR activity of La-VS2−x directly used as the working electrode is evaluated in an H-type cell on the basis of a standard procedure flow chart (Fig. S8†).52–55 The electrolyte used is 0.5 M Na2SO4 with 0.1 M NaNO3 (Fig. S9†). As displayed in Fig. 3a, La-VS2−x exhibits a much higher current density in the presence of NO3−, indicating that La-VS2−x is catalytically effective towards the NO3RR. The NH3 yield rates and FENH3 of La-VS2−x are then quantitatively estimated by the combination of chronoamperometric (Fig. S10†) and colorimetric approaches (Fig. S11–S13†).56–59 As shown in Fig. 3b, La-VS2−x exhibits the highest FENH3 of 96.6% at −0.6 V. The corresponding NH3 yield rate and partial current density at −0.6 V are 11.3 mg h−1 cm−2 and 121.2 mA cm−2 (Fig. S14†), respectively. Such NO3RR performance of La-VS2−x exceeds that of most reported NO3RR catalysts (Fig. 3c, Table S2†). We also investigate the effect of the La-dopant content on the NO3RR performance of La-VS2−x and determine that 4.8 wt% is the optimum La-dopant content (Fig. S15†). Meanwhile, FENH3 is dramatically higher than the FEs of other byproducts shown in Fig. 3d, demonstrating the exceptional selectivity of La-VS2−x for electrocatalytic NO3−-to-NH3 conversion.
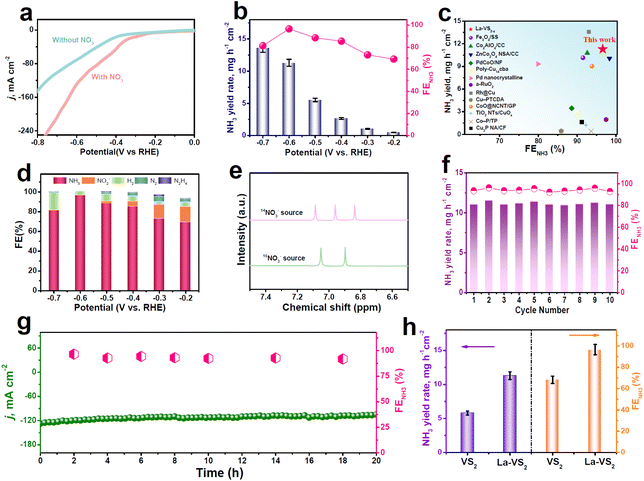 |
| Fig. 3 (a) LSV curves of La-VS2−x with and without the addition of 0.1 M NO3−. (b) NH3 yield rates and FENH3 of La-VS2−x at various potentials. (c) NO3RR performance comparison between La-VS2−x and reported catalysts. (d) FEs of different products after NO3RR electrolysis at various potentials. (e) 1H NMR measurements fed by 14NO3−/15NO3− after NO3RR electrolysis. (f) Cycling and (g) long-term chronoamperometry tests at −0.6 V. (h) Comparison of the NO3RR performance between VS2 and La-VS2−x at −0.6 V. | |
Several control tests are carried out to validate the NH3 origin. It is shown in Fig. S16† that NH3 is barely detected both in the NO3−-free electrolyte and at the open circuit potential (OCP). The N source is further confirmed by isotopic labeling 1H nuclear magnetic resonance (NMR) spectroscopy (Fig. 3e). Visibly, upon using 14NO3− and 15NO3− tracing agents, the resulting NMR spectra show three characteristic signals of 14NH4+ and two signals of 15NH4+, respectively, proving that the detected NH3 originates from the NO3RR.60–62 We further tested the catalytic stability of La-VS2−x for the NO3RR. Fig. 3f depicts no obvious decay in NH3 yield rates and FENH3 during ten consecutive NO3RR cycles, indicating the good cycling durability of La-VS2−x. During the chronopotentiometric test for 20 h of continuous electrolysis (Fig. 3g), negligible variations in current density and corresponding FEHN3 can be observed, suggesting the outstanding long-term stability of La-VS2−x. After the stability tests, La-VS2−x reveals no obvious changes in the morphology, crystal phase and La content (Fig. S17†), confirming the robust structural and compositional stability of La-VS2−x.
We also evaluate the NO3RR activity of pristine VS2 under the same conditions at −0.6 V (Fig. 3h). Obviously, the NO3RR performance of VS2−x is considerably lower than that of La-VS2−x, with FENH3 and the corresponding NH3 yield rate being 1.4 and 1.9 times poorer than those of La-VS2−x, respectively, suggesting that La-dopants have a significant contribution to the NO3RR activity of La-VS2−x. We measured the electrochemical surface areas (ECSAs) of the two catalysts and found that the ECSA-normalized performance of La-VS2−x is still considerably better than that of VS2 (Fig. S18†), indicating the superior intrinsic NO3RR activity of La-VS2−x. The in-depth mechanistic understanding of the enhanced NO3RR of La-VS2−x is elucidated by theoretical investigations.
Since NO3− adsorption is a critical prerequisite for the NO3RR,63 we first examined NO3− adsorption on various catalysts. As shown in Fig. S19,† in contrast to the negligible N
O bond elongation on pristine VS2, the N
O bond of the absorbed NO3− on the VS site of VS2−x is stretched to 1.305 Å, and it is further stretched to 1.365 Å on La-dopant-adjacent VS site of La-VS2−x, indicating that NO3− can be significantly activated on La-VS2−x. This is further corroborated by the differential charge density maps, showing that compared to the cases of VS2 and VS2−x (Fig. S20†), La-VS2−x exhibits a more intense charge transfer with the adsorbed NO3− (Fig. 4a). The corresponding electron location function maps (Fig. 4b) reveal that both the VS-induced unsaturated V atom and the La-dopant (or La-VS site) synergistically donate electrons to the absorbed NO3−,35 resulting in effective NO3− activation on La-VS2−x. Benefitting from the enhanced NO3− activation on the La-VS site, La-VS2−x shows a largely reduced binding free energy of NO3− relative to VS2 and VS2−x (Fig. 4c), thus facilitating the subsequent NO3RR process. Meanwhile, compared to the VS site of VS2−x, the La-VS site of La-VS2−x exhibits a more energy requirement for H2 evolution (Fig. 4d), and thus La-VS2−x can effectively impede the HER to benefit NO3RR selectivity.56
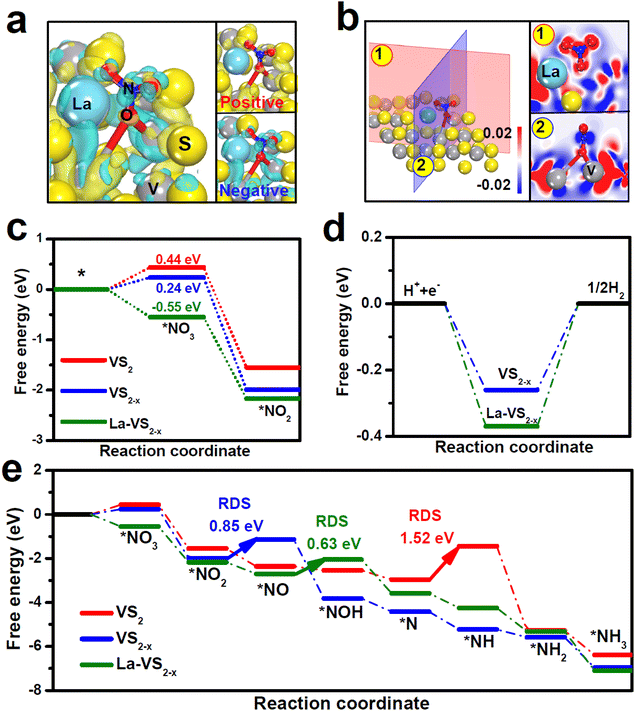 |
| Fig. 4 (a) Differential charge density maps of NO3− adsorption on La-VS2−x (yellow: accumulation, cyan: depletion) and (b) the corresponding electron location function maps (red: accumulation, blue: depletion). (c and d) Binding free energies of (c) NO3− and (d) H on different catalysts. (e) Gibbs free energy diagrams of the NO3RR pathway of VS2, VS2−x and La-VS2−x, respectively. | |
The free energy changes of NO3RR pathways of all considered VS2, VS2−x and La-VS2−x catalysts are further assessed, with their corresponding atom configurations being displayed in Fig. S21–S23.† It is known that the electrocatalytic NO3RR process for NH3 formation comprises the initial deoxidation steps of *NO3 → *NO2 → *NO and the following hydrogenation steps of *NOH → *N → *NH → *NH2 → *NH3.64–66 As shown in Fig. 4e, in comparison with VS2 and VS2−x, La-VS2−x shows the lowest energy barrier of −0.63 eV for its rate-determining step (RDS) of *NO → *NOH, suggesting its favorable energetics to boost the NO3RR process. Therefore, the co-introduction of VS and La-dopants can synergistically promote NO3− activation, retard the HER and lower the reaction energetic barriers, thereby greatly promoting the NO3RR activity and selectivity of La-VS2−x for NO3−-to-NH3 conversion.
3. Conclusion
In summary, La-VS2−x is verified to be a highly active and selective NO3RR catalyst. Theoretical computations reveal that the excellent NO3RR performance of La-VS2−x originates from the synergy of La-dopants and VS to promote NO3− activation, suppress the HER and lower the energetic barriers. This work demonstrates the great potential of rare earth catalysts toward the efficient NO3RR for NH3 electrosynthesis.
Conflicts of interest
There are no conflicts of interest to declare.
Acknowledgements
This work was supported by the Central Government Guides Local Science and Technology Development Project (206Z1003G) and the Longyuan Youth Innovative and Entrepreneurial Talents Project ([2021]17).
References
- J. Liang, Q. Liu, A. A. Alshehri and X. Sun, Recent advances in nanostructured heterogeneous catalysts for N-cycle electrocatalysis, Nano Res. Energy, 2022, 1, e9120010 CrossRef.
- K. Chen, P. Shen, N. Zhang, D. Ma and K. Chu, Electrocatalytic NO reduction to NH3 on Mo2C nanosheets, Inorg. Chem., 2023, 62, 653–658 CrossRef CAS PubMed.
- D. Qi, F. Lv, T. Wei, M. Jin, G. Meng, S. Zhang, Q. Liu, W. Liu, D. Ma, M. S. Hamdy, J. Luo and X. Liu, High-efficiency electrocatalytic NO reduction to NH3 by nanoporous VN, Nano Res. Energy, 2022, 1, e9120022 CrossRef.
- H. Xu, Y. Ma, J. Chen, W.-x. Zhang and J. Yang, Electrocatalytic reduction of nitrate–a step towards a sustainable nitrogen cycle, Chem. Soc. Rev., 2022, 51, 2710–2758 RSC.
- Y. Wang, C. Wang, M. Li, Y. Yu and B. Zhang, Nitrate electroreduction: mechanism insight, in situ characterization, performance evaluation, and challenges, Chem. Soc. Rev., 2021, 50, 6720–6733 RSC.
- P. H. van Langevelde, I. Katsounaros and M. T. Koper, Electrocatalytic nitrate reduction for sustainable ammonia production, Joule, 2021, 5, 290–294 CrossRef.
- N. Zhang, J. Shang, X. Deng, L. Cai, R. Long, Y. Xiong and Y. Chai, Governing interlayer strain in bismuth nanocrystals for efficient ammonia electrosynthesis from nitrate reduction, ACS Nano, 2022, 16, 4795–4804 CrossRef CAS PubMed.
- Y. Wang, H. Li, W. Zhou, X. Zhang, B. Zhang and Y. Yu, Structurally disordered RuO2 nanosheets with rich oxygen vacancies for enhanced nitrate electroreduction to ammonia, Angew. Chem., 2022, 134, e202202604 Search PubMed.
- H. Liu, X. Lang, C. Zhu, J. Timoshenko, M. Rüscher, L. Bai, N. Guijarro, H. Yin, Y. Peng, J. Li, W. Wang, B. Roldan Cuenya and J. Luo, Efficient electrochemical nitrate reduction to ammonia with copper-supported rhodium cluster and single-atom catalysts, Angew. Chem., 2022, 134, e202202556 Search PubMed.
- Q. Gao, H. S. Pillai, Y. Huang, S. Liu, Q. Mu, X. Han, Z. Yan, H. Zhou, Q. He, H. Xin and H. Zhu, Breaking adsorption-energy scaling limitations of electrocatalytic nitrate reduction on intermetallic CuPd nanocubes by machine-learned insights, Nat. Commun., 2022, 13, 2338 CrossRef CAS PubMed.
- X. F. Cheng, J. H. He, H. Q. Ji, H. Y. Zhang, Q. Cao, W. J. Sun, C. L. Yan and J. M. Lu, Coordination symmetry breaking of single-atom catalysts for robust and efficient nitrate electroreduction to ammonia, Adv. Mater., 2022, 34, 2205767 CrossRef CAS PubMed.
- F.-Y. Chen, Z.-Y. Wu, S. Gupta, D. J. Rivera, S. V. Lambeets, S. Pecaut, J. Y. T. Kim, P. Zhu, Y. Z. Finfrock, D. M. Meira, G. King, G. Gao, W. Xu, D. A. Cullen, H. Zhou, Y. Han, D. E. Perea, C. L. Muhich and H. Wang, Efficient conversion of low-concentration nitrate sources into ammonia on a Ru-dispersed Cu nanowire electrocatalyst, Nat. Nanotechnol., 2022, 17, 759–767 CrossRef CAS PubMed.
- Z. Deng, C. Ma, Z. Li, Y. Luo, L. Zhang, S. Sun, Q. Liu, J. Du, Q. Lu, B. Zheng and X. Sun, High-efficiency electrochemical nitrate reduction to ammonia on a Co3O4 nanoarray catalyst with cobalt vacancies, ACS Appl. Mater. Interfaces, 2022, 14, 46595–46602 CrossRef CAS PubMed.
- Z. Deng, C. Ma, X. Fan, Z. Li, Y. Luo, S. Sun, D. Zheng, Q. Liu, J. Du, Q. Lu, B. Zheng and X. Sun, Construction of CoP/TiO2 nanoarray for enhanced electrochemical nitrate reduction to ammonia, Mater. Today Phys., 2022, 28, 100854 CrossRef CAS.
- Y. Xu, Y. Wen, T. Ren, H. Yu, K. Deng, Z. Wang, X. Li, L. Wang and H. Wang, Engineering the surface chemical microenvironment over CuO nanowire arrays by polyaniline modification for efficient ammonia electrosynthesis from nitrate, Appl. Catal., B, 2023, 320, 121981 CrossRef CAS.
- Y. Xu, Y. Sheng, M. Wang, T. Ren, K. Shi, Z. Wang, X. Li, L. Wang and H. Wang, Interface coupling induced built-in electric fields boost electrochemical nitrate reduction to ammonia over CuO@MnO2 core–shell hierarchical nanoarrays, J. Mater. Chem. A, 2022, 10, 16883–16890 RSC.
- T. Ren, Z. Yu, H. Yu, K. Deng, Z. Wang, X. Li, H. Wang, L. Wang and Y. Xu, Interfacial polarization in metal-organic framework reconstructed Cu/Pd/CuOx multi-phase heterostructures for electrocatalytic nitrate reduction to ammonia, Appl. Catal., B, 2022, 318, 121805 CrossRef CAS.
- Y. Zhang, X. Chen, W. Wang, L. Yin and J. C. Crittenden, Electrocatalytic nitrate reduction to ammonia on defective Au1Cu (111) single-atom alloys, Appl. Catal., B, 2022, 310, 121346 CrossRef CAS.
- X. Li, S. Wang, G. Wang, P. Shen, D. Ma and K. Chu, Mo2C for electrocatalytic nitrate reduction to ammonia, Dalton Trans., 2022, 51, 17547–17552 RSC.
- S. Zhang, M. Li, J. Li, Q. Song and X. Liu, High-ammonia selective metal–organic framework–derived Co-doped Fe/Fe2O3 catalysts for electrochemical nitrate reduction, Proc. Natl. Acad. Sci., 2022, 119, e2115504119 CrossRef CAS PubMed.
- W. J. Sun, H. Q. Ji, L. X. Li, H. Y. Zhang, Z. K. Wang, J. H. He and J. M. Lu, Built-in electric field triggered interfacial accumulation effect for efficient nitrate removal at ultra-low concentration and electroreduction to ammonia, Angew. Chem., Int. Ed., 2021, 60, 22933–22939 CrossRef CAS PubMed.
- L. Li, C. Tang, X. Cui, Y. Zheng, X. Wang, H. Xu, S. Zhang, T. Shao, K. Davey and S. Z. Qiao, Efficient nitrogen fixation to ammonia through integration of plasma oxidation with electrocatalytic reduction, Angew. Chem., 2021, 133, 14250–14256 CrossRef.
- P. Gao, Z. H. Xue, S. N. Zhang, D. Xu, G. Y. Zhai, Q. Y. Li, J. S. Chen and X. H. Li, Schottky barrier-induced surface electric field boosts universal reduction of NOx− in water to ammonia, Angew. Chem., 2021, 133, 20879–20884 CrossRef.
- P. Li, Z. Jin, Z. Fang and G. Yu, A single-site iron catalyst with preoccupied active centers that achieves selective ammonia electrosynthesis from nitrate, Energy Environ. Sci., 2021, 14, 3522–3531 RSC.
- Y. Guo, R. Zhang, S. Zhang, Y. Zhao, Q. Yang, Z. Huang, B. Dong and C. Zhi, Pd doping-weakened intermediate adsorption to promote electrocatalytic nitrate reduction on TiO 2 nanoarrays for ammonia production and energy supply with zinc–nitrate batteries, Energy Environ. Sci., 2021, 14, 3938–3944 RSC.
- J. Zhang, T. Wang, D. Pohl, B. Rellinghaus, R. Dong, S. Liu, X. Zhuang and X. Feng, Interface engineering of MoS2/Ni3S2 heterostructures for highly enhanced electrochemical overall-water-splitting activity, Angew. Chem., Int. Ed., 2016, 55, 6702–6707 CrossRef CAS PubMed.
- H. Li, C. Tsai, A. L. Koh, L. Cai, A. W. Contryman, A. H. Fragapane, J. Zhao, H. S. Han, H. C. Manoharan, F. Abild-Pedersen, J. K. Nørskov and X. Zheng, Activating and optimizing MoS2 basal planes for hydrogen evolution through the formation of strained sulphur vacancies, Nat. Mater., 2016, 15, 48–53 CrossRef CAS PubMed.
- Q. Xiang, J. Yu and M. Jaroniec, Synergetic effect of MoS2 and graphene as cocatalysts for enhanced photocatalytic H2 production activity of TiO2 nanoparticles, J. Am. Chem. Soc., 2012, 134, 6575–6578 CrossRef CAS PubMed.
- Q. Li, Y. Guo, Y. Tian, W. Liu and K. Chu, Activating VS2 basal planes for enhanced NRR electrocatalysis: the synergistic role of S-vacancies and B dopants, J. Mater. Chem. A, 2020, 8, 16195–16202 RSC.
- J. Zhang, C. Zhang, Z. Wang, J. Zhu, Z. Wen, X. Zhao, X. Zhang, J. Xu and Z. Lu, Synergistic interlayer and defect engineering in VS2 nanosheets toward efficient electrocatalytic hydrogen evolution reaction, Small, 2018, 14, 1703098 CrossRef PubMed.
- K. Chu, Y. Liu, Y. Li, Y. Guo, Y. Tian and H. Zhang, Multi-functional Mo-doping in MnO2 nanoflowers toward efficient and robust electrocatalytic nitrogen fixation, Appl. Catal., B, 2020, 264, 118525 CrossRef CAS.
- N. Liu, D. Cao, W. Liu, H. Zhang, Y. Zhu, L. Chang, D. Wu and D. Cheng, Constructing La-doped ultrathin Co-based nanostructured electrocatalysts for high-performance water oxidation process, Int. J. Hydrogen Energy, 2022, 47, 14504–14514 CrossRef CAS.
- X. Yang, Y. Ma, Y. Liu, K. Wang, Y. Wang, M. Liu, X. Qiu, W. Li and J. Li, Defect-induced Ce-doped Bi2WO6 for efficient electrocatalytic N2 reduction, ACS Appl. Mater. Interfaces, 2021, 13, 19864–19872 CrossRef CAS PubMed.
- Y. Luo, K. Chen, G. Wang, G. Zhang, N. Zhang and K. Chu, Ce-doped MoS2−x nanoflower arrays for electrocatalytic nitrate reduction to ammonia, Inorg. Chem. Front., 2023 10.1039/d2qi01798a.
- P. Shen, G. Wang, K. Chen, J. Kang, D. Ma and K. Chu, Selenium-vacancy-rich WSe2 for nitrate electroreduction to ammonia, J. Colloid Interface Sci., 2023, 629, 563–570 CrossRef CAS PubMed.
- P. Shen, X. Li, Y. Luo, Y. Guo, X. Zhao and K. Chu, High-efficiency N2 electroreduction enabled by Se-vacancy-rich WSe2−x in water-in-salt electrolytes, ACS Nano, 2022, 16, 7915–7925 CrossRef CAS PubMed.
- X. Li, G. Zhang, P. Shen, X. Zhao and K. Chu, A defect engineered p-block SnS2−x catalyst for efficient electrocatalytic NO reduction to NH3, Inorg. Chem. Front., 2023, 10, 280–287 RSC.
- Y. Luo, P. Shen, X. Li, Y. Guo and K. Chu, Sulfur-deficient Bi2S3−x synergistically coupling Ti3C2Tx-MXene for boosting electrocatalytic N2 reduction, Nano Res., 2022, 15, 3991–3999 CrossRef CAS.
- Y. Luo, Q. Li, Y. Tian, Y. Liu and K. Chu, Amorphization engineered VSe2−x nanosheets with abundant Se-vacancies for enhanced N2 electroreduction, J. Mater. Chem. A, 2022, 10, 1742–1749 RSC.
- L. Zhang, M. Zhou, A. Wang and T. Zhang, Selective hydrogenation over supported metal catalysts: from nanoparticles to single atoms, Chem. Rev., 2019, 120, 683–733 CrossRef PubMed.
- K. Chen, Y. Zhang, J. Xiang, X. Zhao, X. Li and K. Chu, p-block antimony single-atom catalysts for nitric oxide electroreduction to ammonia, ACS Energy Lett., 2023, 8, 1281–1288 CrossRef.
- K. Chen, G. Zhang, X. Li, X. Zhao and K. Chu, Electrochemical NO reduction to NH3 on Cu single atom catalyst, Nano Res., 2023 DOI:10.1007/s12274-023-5384-9.
- K. Chen, J. Wang, J. Kang, X. Lu, X. Zhao and K. Chu, Atomically Fe-doped MoS2−x with Fe-Mo dual sites for efficient electrocatalytic NO reduction to NH3, Appl. Catal., B, 2023, 324, 122241 CrossRef CAS.
- K. Chu, J. Wang, Y. Liu, Q. Li and Y. Guo, Mo-doped SnS2 with rich S-vacancies for highly efficient electrocatalytic N2 reduction: the critical role of Mo-Sn-Sn trimer, J. Mater. Chem. A, 2020, 8, 7117–7124 RSC.
- X. Li, K. Chen, X. Lu, D. Ma and K. Chu, Atomically dispersed Co catalyst for electrocatalytic NO reduction to NH3, Chem. Eng. J., 2023, 454, 140333 CrossRef CAS.
- X. Li, P. Shen, Y. Luo, Y. Li, Y. Guo, H. Zhang and K. Chu, PdFe single-atom alloy metallene for N2 electroreduction, Angew. Chem., 2022, 134, e202205923 Search PubMed.
- P. Shen, X. Li, Y. Luo, N. Zhang, X. Zhao and K. Chu, Ultra-efficient N2 electroreduction achieved over a rhodium single-atom catalyst (Rh1/MnO2) in water-in-salt electrolyte, Appl. Catal., B, 2022, 316, 121651 CrossRef CAS.
- K. Chen, J. Wang, H. Zhang, D. Ma and K. Chu, Self-tandem electrocatalytic NO reduction to NH3 on W single atom catalyst, Nano. Lett., 2023 DOI:10.1021/acs.nanolett.2c04444.
- W. Zhang, M. Jiang, S. Yang, Y. Hu, B. Mu, Z. Tie and Z. Jin,
In situ grown CuOx nanowire forest on copper foam: A 3D hierarchical and freestanding electrocatalyst with enhanced carbonaceous product selectivity in CO2 reduction, Nano Res. Energy, 2022, 1, e9120033 CrossRef.
- L. Zhang, J. Liang, L. Yue, K. Dong, J. Li, D. Zhao, Z. Li, S. Sun, Y. Luo, Q. Liu, G. Cui, A. Ali Alshehri, X. Guo and X. Sun, Benzoate anions-intercalated NiFe-layered double hydroxide nanosheet array with enhanced stability for electrochemical seawater oxidation, Nano Res. Energy, 2022, 1, e9120028 CrossRef.
- F. Guo, M. Zhang, S. Yi, X. Li, R. Xin, M. Yang, B. Liu, H. Chen, H. Li and Y. Liu, Metal-coordinated porous polydopamine nanospheres derived Fe3N-FeCo encapsulated N-doped carbon as a highly efficient electrocatalyst for oxygen reduction reaction, Nano Res. Energy, 2022, 1, e9120027 CrossRef.
- X. Li, P. Shen, X. Li, D. Ma and K. Chu, Sub-nm RuOx clusters on Pd metallene for synergistically enhanced nitrate electroreduction to ammonia, ACS Nano, 2023, 17, 1081–1090 CAS.
- K. Chen, Z. Ma, X. Li, J. Kang, D. Ma and K. Chu, Single-atom Bi alloyed Pd metallene for nitrate electroreduction to ammonia, Adv. Funct. Mater., 2023 DOI:10.1002/adfm.202209890.
- G. Wang, P. Shen, Y. Luo, X. Li, X. Li and K. Chu, A vacancy engineered MnO2−x electrocatalyst promotes electroreduction of nitrate to ammonia, Dalton Trans., 2022, 51, 9206–9212 RSC.
- G. Zhang, X. Li, K. Chen, Y. Guo, D. Ma and K. Chu, Tandem electrocatalytic nitrate reduction to ammonia on MBenes, Angew. Chem., Int. Ed., 2023 DOI:10.1002/anie.202300054.
- N. Zhang, G. Zhang, P. Shen, H. Zhang, D. Ma and K. Chu, Lewis acid Fe-V pairs promote nitrate electroreduction to ammonia, Adv. Funct. Mater., 2023 DOI:10.1002/adfm.202211537.
- Y. Luo, K. Chen, P. Shen, X. Li, X. Li, Y. Li and K. Chu, B-doped MoS2 for nitrate electroreduction to ammonia, J. Colloid Interface Sci., 2023, 629, 950–957 CrossRef CAS PubMed.
- K. Chu, X. Li, Q. Li, Y. Guo and H. Zhang, Synergistic enhancement of electrocatalytic nitrogen reduction over boron nitride quantum dots decorated Nb2CTx-MXene, Small, 2021, 17, 2102363 CrossRef CAS PubMed.
- Y. Cheng, X. Li, P. Shen, Y. Guo and K. Chu, MXene quantum dots/copper heterostructure for synergistically enhanced N2 electroreduction, Energy Environ. Mater., 2023, 6, e12268 CAS.
- Q. Li, P. Shen, Y. Tian, X. Li and K. Chu, Metal-free BN quantum dots/graphitic C3N4 heterostructure for nitrogen reduction reaction, J. Colloid Interface Sci., 2022, 606, 204–212 CrossRef CAS PubMed.
- K. Chu, Y. Luo, P. Shen, X. Li, Q. Li and Y. Guo, Unveiling the synergy of O-vacancy and heterostructure over MoO3−x/MXene for N2 electroreduction to NH3, Adv. Energy Mater., 2022, 12, 2103022 CrossRef CAS.
- X. Li, Y. Luo, Q. Li, Y. Guo and K. Chu, Constructing an electron-rich interface over an Sb/Nb2CTx-MXene heterojunction for enhanced electrocatalytic nitrogen reduction, J. Mater. Chem. A, 2021, 9, 15955–15962 RSC.
- K. Chen, G. Wang, Y. Guo, D. Ma and K. Chu, Iridium single-atom catalyst for highly efficient NO electroreduction to NH3, Nano Res., 2023 DOI:10.1007/s12274-023-5556-7.
- D. Wu, P. Lv, J. Wu, B. He, X. Li, K. Chu, Y. Jia and D. Ma, Catalytic active centers beyond transition metals: atomically dispersed alkaline-earth metals for electroreduction of nitrate to ammonia, J. Mater. Chem. A, 2023, 11, 1817–1828 RSC.
- B. He, P. Lv, D. Wu, X. Li, R. Zhu, K. Chu, D. Ma and Y. Jia, Confinement catalysis of a single atomic vacancy assisted by aliovalent ion doping enabled efficient NO electroreduction to NH3, J. Mater. Chem. A, 2022, 10, 18690–18700 RSC.
- Y. Wang, A. Xu, Z. Wang, L. Huang, J. Li, F. Li, J. Wicks, M. Luo, D.-H. Nam, C.-S. Tan, Y. Ding, J. Wu, Y. Lum, C.-T. Dinh, D. Sinton, G. Zheng and E. H. Sargent, Enhanced nitrate-to-ammonia activity on copper–nickel alloys via tuning of intermediate adsorption, J. Am. Chem. Soc., 2020, 142, 5702–5708 CrossRef CAS PubMed.
|
This journal is © the Partner Organisations 2023 |
Click here to see how this site uses Cookies. View our privacy policy here.