DOI:
10.1039/D3QM00069A
(Review Article)
Mater. Chem. Front., 2023,
7, 2297-2315
Triplet–triplet annihilation mediated photon upconversion solar energy systems
Received
19th January 2023
, Accepted 27th March 2023
First published on 3rd April 2023
Abstract
Solar energy harvesting is among the best solutions for a global transition toward carbon-neutral energy technologies. The existing solar energy harvesting technologies like photovoltaics (PV) and emerging molecular concepts such as solar fuels and molecular solar thermal energy storage (MOST) are rapidly developing. However, to realize their full potential, fundamental solar energy loss channels like photon transmission, recombination, and thermalization need to be addressed. Triplet–triplet annihilation mediated photon upconversion (TTA-UC) is emerging as a way to overcome losses due to the transmission of photons below the PV/chromophore band gap. However, there are several challenges related to the integration of efficient solid-state TTA-UC systems into efficient devices such as: wide band absorption, materials sustainability, and device architecture. In this article, we review existing work, identify and discuss challenges as well as present our perspective toward possible future directions.
Introduction
The solar energy of the sun has been an external power source to earth for 4.5 billion years. Around 3.4 billion years ago, the earth developed a method to store solar energy in chemical bonds through photosynthesis by cyanobacteria and later by plants.1 Since the discovery of fire, humans have been using various forms of stored solar energy like fossil fuels or wood to sustain life.2–4 However, the rise in labour-intensive industrialization of human society has exponentially increased fossil energy consumption,5 eventually causing global warming due to carbon emission being higher than the natural carbon fixation cycle. Further warming of the earth can pose a danger to the survival of various species including humans in the imminent future. Hence, global efforts are increasing for development of sustainable clean energy technologies with a minimum carbon footprint. Carbon-neutral energy technology is also the key agenda of the Paris Agreement,6 2016 on climate change.
Solar energy technologies have emerged as a sustainable alternative to fossil energy albeit with efficiency in the range of 15–20% for commercial crystalline silicon (c-Si) solar cells.7 The maximal possible efficiency of single junction solar cell is described by the Shockley–Queisser (SQ) limit (33% theoretical maximum) arising due to different loss channels such as charge recombination, transmission loss of sub-bandgap photon, and thermalization loss of photons higher than the solar cell band gap.8 Alternatives to c-Si solar cells9 such as dye-sensitized solar cells,10,11 multijunction solar cells,12 and perovskite solar cells13 are emerging fast but still far from commercial utility due to many factors including conventional band gap related loss channels. The transmission loss (33%) can be addressed by integrating a suitable photon upconverter into the solar cell to convert transmitted photons into the solar cell absorption window.14 In this regard, triplet–triplet annihilation mediated photon upconversion (TTA-UC) is emerging as a promising photon upconversion process wherein two low-energy triplet excitons combine to form one high-energy singlet photon that can be infused into solar cells to overcome transmission losses.15,16 Hence, TTA-UC has the potential to push the solar energy conversion limit to 44%.15 Similar to the PV systems, solar fuel systems in general and molecular solar thermal energy storage (MOST) systems in particular also face transmission losses due to the small absorption window of molecular systems used. Practically fabricable and efficient MOST windows and chips could turn out to be a sustainable energy resource for the heating/cooling of houses and thermoelectric generators.17,18 Therefore, integration with TTA-UC materials can enhance the efficiency of the MOST systems by harvesting a larger amount of solar photons.
In this regard, here we have reviewed existing studies on TTA-UC integrated solar energy systems (PV & MOST) and challenges associated with the realization of efficiency enhancement. Specifically, we focus on challenges related to the fabrication of efficient solid-state TTA-UC systems with wide band upconversion, materials sustainability, PV, and MOST systems with a suitable bandgap. Elaborating, for example, (1) lack of solar thermal energy systems and TTA-UC systems with optimal spectral overlap, (2) efficiency of both the systems in the solid/semi-solid state, (3) physical losses due to scattering of the upconverted photons in all directions. We have also presented our perspective for further possible TTA-UC-PV and TTA-UC-MOST device design improvements.
However, these improvements are subject to the efficiency of TTA-UC near the solar irradiance which depends on many factors discussed below.
Triplet–triplet annihilation photon upconversion
TTA-UC is a photophysical process of generating one high-energy singlet photon upon annihilation of two lower-energy triplet excitons.19–21 TTA-UC occurs through a series of triplet energy transfer events in an ensemble of annihilator chromophores doped with triplet sensitizer.22 The triplet sensitizer or donor (D) upon excitation at lower energy move to the photo-emissive triplet state either via intersystem crossing (ISC) through S1 or direct S0–T absorption.19–23 While relaxing to the ground state, the sensitizer excites the annihilator to its triplet state via triplet energy transfer through electron exchange. When two sensitized annihilator triplets encounter, they undergo TTA that results in the formation of a higher energy singlet state through spin inversion and emit the upconverted photon (Fig. 1).19–26 However, the efficacy of obtaining the upconverted photon is subject to many factors like distance, energetics, and specific orientation of the involved molecules. For example, the electron exchange between sensitizer–annihilator or annihilator–annihilator occurs via Dexter energy transfer (DET).27
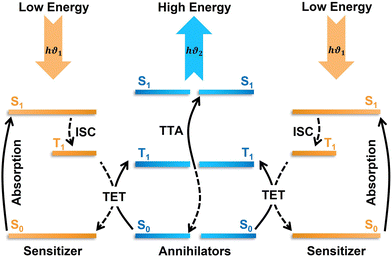 |
| Fig. 1 The scheme of TTA-UC with indicated energy transfer processes. ISC – intersystem crossing, TET – triplet energy transfer, TTA – triplet–triplet annihilation. | |
The rate of DET (kDET) is inversely proportional to the sum of van der Waals radii (L) of the sensitizer and annihilator following eqn (1).27
| 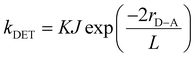 | (1) |
where
J is the normalized spectral overlap integral,
K is an experimental factor that indicates specific orbital overlap corresponding to the instantaneous orientations of a sensitizer and annihilator, and
rD–A is the distance between the sensitizer and annihilator with respect to the
L. For DET,
rD–A should be less than 10 Å since it requires an overlap of the wavefunctions of the highest occupied or lowest unoccupied molecular orbitals (HOMO or LUMO) of the involved molecules. The normalized spectral overlap integral (
J) can be defined by
eqn (2).
| 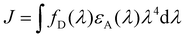 | (2) |
where (
fD) and (
εA) are normalized emission intensity of a donor and normalized extinction coefficient of an annihilator, respectively.
The performance of TTA-UC is evaluated using upconversion quantum yield (ϕUC) that is a function of many other factors related to eqn (3).
| 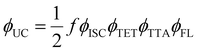 | (3) |
where
ϕISC,
ϕTET,
ϕTTA,
ϕFL denote quantum yields of the intersystem crossing in the sensitizer (ISC), sensitizer to annihilator triplet energy transfer (TET), triplet–triplet annihilation (TTA), and fluorescence of the annihilator (FL). The factor
f denotes the statistical probability of the formation of one emissive singlet state upon annihilation of two triplet excitons, and ½ indicates the emission of one photon upon absorption of two photons, which sets the limit of
ϕUC to be 50%.
Experimentally, ϕUC is calculated via relative method using reference standard28,29 or absolute method using integrating sphere.30 In the relative method, a reference fluorescent dye or upconversion system having an overlapping absorption with the triplet sensitizer of the TTA-UC system under investigation is used as a reference standard using eqn (4).28,29
| 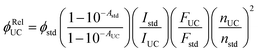 | (4) |
where
ϕUC,
ϕstd,
AUC,
Astd,
IUC,
Istd,
FUC,
Fstd and
nUC,
nstd are quantum yield, absorbance, excitation intensity, integrated UC emission profiles, and refractive index of the UC sample and reference standard, respectively. While the relative method remains suitable for
ϕUC calculation in the solution state, the integrating sphere is preferred for solid-state measurements to avoid thickness-related absorption errors. However, inherent self-absorption of the UC photons and reabsorption of the emitted UC photons reflected by the integrating sphere are secondary loss channels that give errors in the absolute
ϕUC measured in the integrating sphere. Kimizuka and Yanai's group
30 devised a method to address the reabsorption of the reflected UC photons. For this, they calculated the reabsorption probability (
a) in the integrating sphere to correct absolute

calculation according to
eqn (5).
30 | 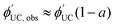 | (5) |
where

is observed UC quantum yield and

is the UC quantum yield corrected for reabsorption probability. The
a is evaluated by
eqn (6).
| 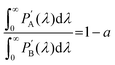 | (6) |
where,

and

are UC photoluminescence spectra in photons per wavelength normalized with integrated phosphorescence spectra of a sensitizer, measured inside and outside of the integrating sphere. The UC quantum yields calculated using this method give similar values to that calculated using a relative method in solution.
30 It was also found true for green to blue bioplastics film
31 thus validating its applicability towards solid-state TTA-UC systems.
The inherent UC photon losses due to the secondary inner filter effect, caused by the reabsorption of UC photons by the sensitizer due to overlapping spectra can be corrected by the conversion factor (Fc) as per eqn (7).32
| 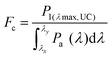 | (7) |
where
PI and
Pa are normalized photoluminescence intensity at maximum UC emission wavelength of annihilator and normalized integrated emission of annihilator at low concentration, respectively. Consequently, the correct
ϕUC can be calculated using
eqn (8).
32 | 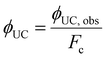 | (8) |
However, it must be noted that only
ϕUC,obs will be used upon TTA-UC integration with the devices. The only way to avoid inherent reabsorption losses is a suitable design of the sensitizer–annihilator pairs with non-overlapping spectra and fabrication of low-thickness samples.
The quantum yield of each energy transfer step within the TTA-UC systems is of significant importance to obtain maximum ϕUC required for efficient integration with solar energy systems. Therefore, we will briefly discuss the state-of-art to obtain a complete picture of the energetic requirements for sensitizer, and annihilator molecules and the progress made to achieve these requirements. Basically, the quantum yield (ϕ) is the number of photons emitted per number of photons absorbed by the system.33 Therefore, when considering device integration of TTA-UC, the sensitizer being a key absorber must possess the following properties (Fig. 2). First, it must have a broad absorption cross-section in addition to a high molar extinction coefficient (ε) to harvest maximum photons. Second, the sensitizer must have a small energy gap between the singlet and triplet states (ΔES–T) for a high rate of spin-forbidden ISC (kISC). Third, because of the bimolecular nature of TTA-UC, the sensitizer must have a long triplet lifetime to meet an annihilator for transferring energy in its lifetime. And fourth, it must have a broad optical transparency window between the bands preceding the key absorption band to avoid inherent reabsorption of the upconverted photons by the sensitizer. For example, the region between the Q-band (S0–S1) and Soret band (S0–S2) of the porphyrins.34
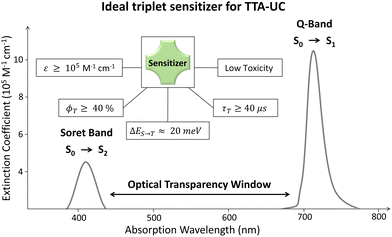 |
| Fig. 2 Illustration of the photophysical properties of an ideal triplet sensitizer for TTA-UC. | |
Most of the triplet sensitizers used in TTA-UC are organometallic complexes (porphyrins, phthalocyanines, or their metal–organic) complexes,35–38 thermally activated delayed fluorescence (TADF)39,40 molecules, and BODIPY dyes35,41 which all have their strengths and challenges. For example, the red/green light-absorbing transition metal porphyrins have a reasonable phosphorescence quantum yield (ϕT = 5% to 50%) and phosphorescence lifetime (τT = 20 μs to 300 μs) but low ε of the Q-band as compared to the Soret band. Metal phthalocyanines on the other hand have a significant ε = 2 × 105 M−1 cm−1 of the Q-band and high ϕT > 40% but low τT < 5 μs.37 The direct S0–T absorbing substituted Os-complexes have emerged as a potential NIR sensitizers with zero energy loss during ISC and have τT = 81 μs, but have low ϕT = 3.1 to 5.4%, high absorption between the Soret and Q-band and a small Stokes shift.35,41 Due to the toxicity of transition metals, halogenated BODIPY dyes with high ε and ϕT have emerged as alternative photosensitizers.35,41 The introduction of heavy atoms increases the ϕISC of BODIPY due to the increased spin–orbit coupling that decreases the ΔES–T to increase the ϕT.41 However, the application of BODIPY in TTA-UC is mainly limited to Vis-Vis TTA-UC and realisation of NIR absorbing BODIPY is needed for practical application in solar energy storage systems. The TADF or multi-resonance TADF (m-TADF) are emerging as another class of promising sensitizers due to small ΔES–T (20–40 meV), but the rate of reverse intersystem crossing (RISC) is a limiting step that should be outperformed for high kISC. However, the TADF have low ε and small optical transparency window that are essential for solar energy harvesting applications. Apart from the organic/metal organic sensitizers, the quantum dots42–44 and metal halide perovskite nanocrystals45 have emerged as promising triplet sensitizers due to high ε and tunable absorption/emission. However, they have many challenges for practical applications such as complicated synthesis, high excitation intensities (W cm−2), the strong back Förster resonance energy transfer (FRET), etc. that affects the triplet energy transfer to annihilator. Hence, an ideal TTA-UC sensitizer must have a low toxicity, ε ≥ 105 M−1 cm−1 of Q-band, broad optical transparency window between Soret band and Q-band (for porphyrins and phthalocyanines), ϕT ≥ 40%, ΔES–T ≈ 20 meV, and τT ≥ 30 μs. Additionally, the sensitizer must have an appropriate molecular orientation (factor K in eqn (1)) or suitable transmitter/mediator for high ϕTET to the annihilator.
The ϕTET between the sensitizer and annihilator molecules is usually regarded as a challenging step in TTA-UC systems. The TET between the molecules is accomplished via DET, that has a distance limit of up to 1 nm and functions according to eqn (1). In the solution state, due to the high molecular diffusion the ϕTET can be enhanced by increasing the annihilator concentration to maximize the probability of sensitizer–annihilator collision. However, the aggregation of polyaromatic dyes at high concentrations can cause emission quenching in addition to primary and secondary inner filter effects.36,46,47
Dimeric and polymeric annihilators of DPA/perylene have emerged as alternatives to limit the concentration factor.48,49 In such systems, triplet energy can migrate intramolecularly in the dimers or polymers to minimize the energy loss due to diffusion and to increase the TTA rates, but have typically been limited to Vis to Vis photon upconversion. Additionally, they face challenges with aggregation quenching in the solid-state which is key for practical applications of TTA-UC. Recently, Congrave et al. found a synthetic route to suppress aggregation-induced fluorescence quenching with a conjugated polymer-strapped derivative of diphenylanthracene (DPA polymer).50 Interestingly the DPA polymer showed a similar fluorescence quantum yield (ϕf ≈ 40%) both in solution and spin coated solid-state films. Thus gave new synthetic directions for an annihilator design for solid-state devices.
The ϕTET can also be increased by attaching a triplet energy transmitter/mediator to the sensitizer. For example, the case with a quantum dot or perovskite nanocrystal-based sensitizers. However, these systems have been also found efficient only in the solution state due to the phase separation of quantum dots in the solid-state.51 Another promising way to increase the ϕTET is a suitable tuning of the annihilator molecular structure so that it has triplet energy levels very close to that of the sensitizers (ΔET ≥ 4kbT).38,52
The ϕTET is generally calculated from quenching of the phosphorescence quantum yield (ϕp) or lifetime (τp) of sensitizer with and without (ϕpo, τpo) annihilator according to eqn (9).
| 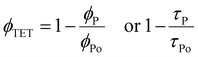 | (9) |
An example of TTA-UC in the solid-state demonstrating an increase in emission of annihilator at the expense of sensitizer's phosphorescence due to TET and subsequent TTA is shown in
Fig. 3.
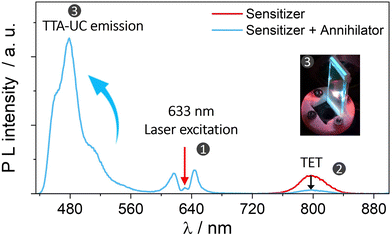 |
| Fig. 3 Plot illustrating UC emission of annihilator (blue line) at the expense of sensitizer's phosphorescence (red line) due to subsequent TET and TTA processes in the solid-state bioplastic film (inset). Part of the figure is reproduced from ref. 32 with permission from the Royal Society of Chemistry. | |
Other than ϕUC, threshold excitation intensity (Ith) of the TTA-UC system is an important parameter for solar energy applications. An efficient system with Ith near the solar irradiance would be more suitable for practical application. Therefore in the coming section, we will discuss Ith concerning solar energy applications.
Upconversion threshold
TTA-UC is a process that depends on the excitation power density in two stages. The first stage is a quadratic dependence of UC emission intensity on excitation power density which marks the non-saturation regime as the concentration of triplet excited molecules is relatively low. In this case, the TTA process may appear rarely as the collision of two excited molecules is required to produce an emissive singlet state. That is why the probability of TTA grows quadratically with excitation power density until almost half of the molecules are excited. The second stage of TTA-UC is a linear growth of UC emission with excitation power density. This regime shows a saturation concentration situation of excited molecules where each additional triplet excited state finds an already existing counterpart to undergo TTA. As it follows, the intersection of quadratic and linear regimes is called the UC threshold (Ith) and equals 38.2%53 of a maximum UC emission intensity or quantum yield. The Ith can be defined as follows:54 | 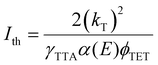 | (10) |
where kT is the triplet decay rate, γTTA is bimolecular annihilation constant, α(E) is sensitizer's absorption at excitation energy, ϕTET is TET quantum yield.
Usually, the Ith is required to be much lower than the solar energy density of a certain region for TTA-UC to operate efficiently. The Ith was previously thought to be equal to 50% of the maximum UC quantum yield (ϕ∞UC), however, recent work by Murakami et al.53 clarified that this point corresponds only to 38.2% of the ϕ∞UC. Also, authors highlighted a new way to determine the Ith and ϕ∞UC accurately by fitting the experimental dependence of ϕUC on excitation intensity (Iex) even if the saturation regime is not achieved as per eqn (11).
| 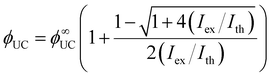 | (11) |
This, in turn, allows evaluation of the statistical probability factor (
f) of the annihilator by using
ϕ∞UC of the system as the
ϕTTA approaches unity, which we will discuss in the next section.
Statistical probability factor
The statistical probability factor (f) is an intrinsic property of an annihilator molecule that refers to the probability of a singlet state generation through the annihilation of two triplets. The annihilation takes place due to the coupling of the wavefunction of two triplet spin states aligned either parallel or antiparallel to each other. It can be simply expressed in Heisenberg's spin only Hamiltonian, eqn (12).55,56where Ŝ1 and Ŝ2 are individual spin operators of the interacting triplets and J is the magnetic exchange parameter. The J contains all of the spatial information of the wave function for coupling through-space and through-bond interactions which determine the ground-state spin preferences. The triplet coupling results in 9 spin-pair eigen states; 1 singlet, 3 triplets, and 5 quintets which are graded corresponding to the spin multiplicities in the ratio of 1
:
3
:
5 according to the Glebsch–Gordan series, respectively (Fig. 4).57 As specified by the spin statistics, the limit of the f is defined as 1/9, but the possibility to recycle a triplet state from quintet or higher energy triplet can enlarge this theoretical value to 1/5.58
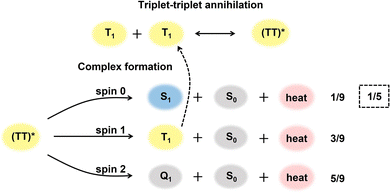 |
| Fig. 4 The scheme of a singlet, triplet, and quintet encounter complex formation via TTA. The statistical probabilities for each encounter are provided. The dashed line represents the triplet state recycling process increasing f to 1/5. | |
However, this is possible only if the coupling of triplets occurs via anti-ferromagnetic coupling (AFC), which reverses the energetic order of spin states against the Hund's rule according to eqn (13).55
| Es = −J(S(S + 1) − 4) | (13) |
where
Es is the energy of the spin-state,
J is the magnetic exchange parameter and
S is the total angular momentum of the annihilation triplets. If
J > 0, similar spins of two triplets interact
via ferromagnetic coupling (FC) making the quintet the lowest energy state, and if
J < 0 opposite spins of two triplets interact
via anti-ferromagnetic coupling (AFC), hence, the quintet becomes the highest energy state followed by triplet and singlet (
Fig. 5a). It can be understood by putting the value of
J = +1 or −1 and
S = 0, 1 and 2 for singlet, triplet and quintet state in
eqn (13). As it can be seen from
Fig. 5b, that post-TTA events discards complete quenching of the photons of higher energy quintet and triplet states. Hence they can participate in TTA coupling to increase the spin statistical probability of singlet formation beyond 1/9
via reverse intersystem crossing (RISC)
59 or spin mixing of singlet–quintet states.
60
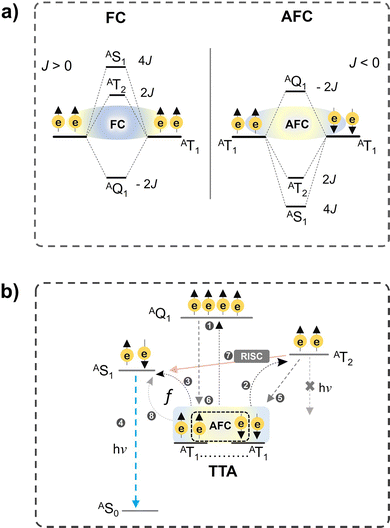 |
| Fig. 5 Illustration of (a) ferromagnetic (FC) and anti-ferromagnetic coupling (AFC) of triplets, and (b) post-TTA events like UC emission and reverse intersystem crossing (RISC), and quintet or triplet recycling to the T1. (b) is reproduced from ref. 21 with permission from the Royal Society of Chemistry. | |
Experimentally, f can be defined according to eqn (14) where it depends on ϕ∞UC and energy transfer processes within the TTA-UC system.36,37,61 Also, the f value depends on bimolecular annihilation constant (γTTA), annihilation radius (R) and diffusion coefficient (D) of annihilator as described by Köhler et al.57 in eqn (15). Therefore, f depends on the molecular structure, energy state distribution, and orientation of the annihilator molecule during triplet coupling.
| 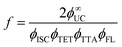 | (14) |
|  | (15) |
For example, the
f of some annihilators (DPA, TIPS-naphthalene,
etc.) have been reported to exceed 1/5
36,49,62,63 as their energy state distribution is suitable for the TTA process (2T
1 > S
1) and hinders other non-radiative decay channels such as singlet-fission (SF) or high energy triplet states (T
n). The 2T
1 energy loss to T
n was theoretically predicted to be the dominating non-radiative decay channel negatively affecting the
f.
58,64 Recently, it was proven with diketopyrrolopyrrole (DPP) emitters that decreasing the energy gap between 2T
1 and T
2 was responsible for the decrease in
f.
61 Hence it is essential to have a large energy gap between 2T1 and T
n energy to have high
f.
Considering the possible importance of f in annihilator design to achieve desired UC quantum yield, we have reviewed the reported f values of the widely used TTA annihilators over the UV-Vis spectral range to find possible trends or structure–property relations (Fig. 6). The f values for some of the annihilators have been calculated by us using the method proposed by Murakami et al.53 from the published data of other parameters considering ϕTTA = 1, when ϕ∞UC becomes invariant with excitation intensity and has been shown as approximate value.
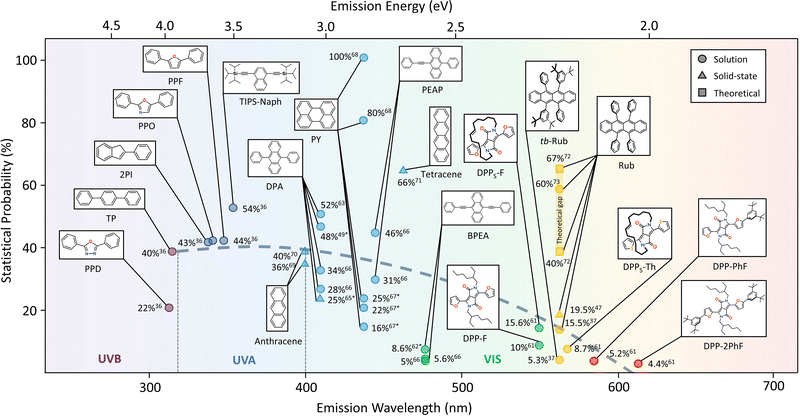 |
| Fig. 6 Plot showing variations in statistical probability factor values for TTA annihilators over UVB-to-red spectral range in solution (circle), solid-state (triangle). The values in square show value calculated theoretically. The dashed line indicates variation of statistical probability factor with an increasing emission wavelength of annihilators. Values marked with asterisk (*) are not directly reported but deduced from the data presented in publications. | |
Interestingly, barring a few exceptions, we found a trend of decrease in f values of TTA annihilators with an increase in branched conjugation from UV to NIR region in solution. For example, an average f ≈ 40% was observed for the UV-B (PPT, TP)36 and UV-A (2PI, PPO, PPF)36 region that decreased to f ≈ 25 to 35% in the blue region (DPA, perylene, PEAP),49,63,65–67f ≈ 10 to 15% in the green region (BPEA, DPPs-F, DPP-F),61,62,66f ≈ 10 to 20% in the yellow region (rubrene, tb-rubrene, DPPs-Th),37,47,61 and f ≈ 5% in the red-region (DPP-PhF, DPP-2PhF).61 An exceptionally high f ≈ 54%36 was observed for TIPS-Naph showing high ϕUC = 17% highlighting the importance of f in the final output of TTA in addition to other energy transfer steps. It was also found true for DPA49,63,65,66 and perylene,46,67,68 although with a lot of variations in f observed by different research groups (Fig. 6). The f value of perylene in particular is a bit disputed since there is a large variation from 16%67 to 100%.68 For example, f = 100%68 seems to be overestimated as the T2 state in perylene tends to be around 3.1 eV46,67 and not at 4.0 eV as reported.68 This means that T2 is not energetically far from 2T1, hence there is an accessible non-radiative loss channel to alter f. Interestingly, f increased with an increase in linear conjugation from anthracene (f = 36–40%)69,70 to tetracene (f = 66%)71 in crystalline state. But there are not any available reported crystalline systems with enhanced linear conjugation to find any trend. Rubrene has been the leading yellow emitting annihilator in TTA-UC. Recenlty, Bossanyi et al.72 modelled f values of rubrene in different molecular orientations and showed that f changes from 40% to 67% from perpendicular to parallel orientation due to spin-mixing of spin-pair wavefunctions.72 The values are close to the experimental value (f = 60%), reported by Schmidt et al.73 But that experiment was carried out using high energy pulsed laser.73 Moreover, the maximum f = 15.5% (solution) and 19.5% (solid-state) has been reported for native rubrene37,47 that decreases further to 5.3% due to tert-butylation in steady-state measurements.37 Hence, the lower f could be one of the main reasons for low TTA-UC quantum yield observed for rubrene based TTA-UC systems.37,47 However, we strongly believe that molecular orientation during inter-triplet coupling plays a key role in deciding the f value72,74 and side chain branching or enhanced conjugation alters most suitable orientation for effective coupling. It was also found true for red-emitting annihilators showing low f values of around 5%.61
The observed trend may also be influenced by the energy gap law75,76 considering the differences in achievable higher triplet states (Tn). For example, in the UV-blue region, the 2T1 is able to access the Tn states leading to higher f values possibly due to the higher Tn–T1 and T1–S0 energy differences compared to the lower energy emission annihilators. The higher Tn–T1 energy differences may impede the internal conversion to T1, therefore leading to more pronounced RISC to S1. This, in turn, might have a positive effect on f. However, in the green to red region, the Tn–T1 and T1–S0 energy differences are smaller and, according to the energy gap law, may facilitate faster decay of triplets affecting the possibility to recycle the Tn states efficiently. As follows, this cause may contribute to the reduced f values. The trend also shows that possible far-red and NIR annihilators may not have a significant f value to harvest photons from below the crystalline silicon band gap to realize high UC quantum yield for practical implementations in the far-red and NIR region if suitable molecular orientation for inter-triplet coupling is not achieved.
Solid-state TTA-UC for solar energy systems
While most efficient TTA-UC systems have been reported in degassed solutions,77–79 practical integration with solar energy systems requires them to function efficiently in the solid-state. But the fabrication of the efficient solid-state TTA-UC faces many challenges: (1) aggregation-induced quenching of singlet/triplet energy of chromophores; (2) triplet quenching by molecular oxygen; (3) small upconversion windows, etc.22,80,81 Additionally, the non-recyclability of petro-polymers used for solid-state fabrication and the toxicity of TTA-UC chromophores is another challenge that hampers the sustainability of solid-state TTA-UC materials.82,83 Many of these challenges have been addressed by employing various solid-state fabrication strategies. For example, by tuning the molecular structure of sensitizer–annihilator, their concentrations, crystallization, glassification, doping in amorphous polymers and bioplastics, etc.22,80 From the application point of view, the solid-state TTA-UC systems can be divided into three categories: (1) near-infrared to Visible (NIR-to-Vis); (2) visible to Visible (Vis-to-Vis) and; (3) visible to ultraviolet (Vis to UV). Under the current scope of this review, we will mainly discuss the most efficient solid-state systems (Table 1) as well as their applications in solar energy systems. Detailed information about the developments in solid-state TTA-UC systems can be found in the papers published elsewhere.21,80,84
Table 1 Most efficient solid-state TTA-UC systems over UV-to-NIR spectral range. Matrix, polymer glass transition temperature (Tg), energy difference of anti-Stokes shift (ΔEUC), UC threshold (Ith) and UC quantum yield (ϕUC) indicated. ΔEUC here is presented as difference between wavelength of excitation and maximum of emission wavelength
Sensitizer–annihilator-emitter |
Matrix |
T
g (°C) |
UC range (nm) |
ΔEUC (eV) |
I
th (W cm−2) |
ϕ
UC (%) |
Near infrared sensitized TTA-UC (λex > 780 nm) |
Os(atpy)(tbbpy)Cl+-rubrene-DBP85 |
PVA film |
80 |
938 to 625 |
0.66 |
125 |
2.05 |
PdTPTAP-rubrene88 |
PVA film |
80 |
810 to 560 |
0.68 |
1.40 |
3.65 |
PdSNC-rubrene-DBP86 |
Amorphous film |
— |
808 to 610 |
0.50 |
— |
0.53 |
Y6-rubrene138 |
Thin bilayer |
— |
850 to 575 |
0.70 |
— |
0.52 |
|
Far-red sensitized TTA-UC (700 nm < λex < 780 nm) |
MoSe2-rubrene-DBP87 |
Thin bilayer |
— |
772 to 610 |
0.43 |
0.26 |
1.1 |
PdPc-rubrene47 |
Polystyrene |
100 |
730 to 575 |
0.46 |
1.40 |
1.2 |
|
Red sensitized TTA-UC (620 nm < λex < 700 nm) |
PdTPBP-TIPS-anthracene32 |
Gelatin |
— |
633 to 478 |
0.64 |
0.095 |
8.8 |
PdTPBP-anthracene89 |
Crystals |
— |
635 to 470 |
0.69 |
0.017 |
5 |
Pd(OBu)8Pc-rubrene90 |
Polyacrylate |
44–48 |
670 to 560 |
0.36 |
— |
15 |
|
Green sensitized TTA-UC (532 nm < λex < 550 nm) |
PdOEP-DPA90 |
Polyacrylate |
48–52 |
543 to 435 |
0.57 |
0.0014 |
16 |
PtOEP-DPA91 |
Polyurethane |
−42 |
532 to 430 |
0.55 |
0.022 |
11 |
PtOEP-DPA139 |
LAPONITE®/PVP |
— |
532 to 435 |
0.57 |
0.033 |
23.8 |
PtOEP-DPA65 |
PMMA |
108 |
532 to 430 |
0.55 |
0.005 |
8 |
PtOEP-ANNP92 |
Crystals |
— |
542 to 434 |
0.57 |
0.0007 |
16 |
PtOEP-C7-sDPA93 |
Crystals |
— |
532 to 434 |
0.53 |
0.005 |
10 |
|
Blue to UV TTA-UC (400 nm < λex < 532 nm) |
Ir(ppy-DBP)-DBP100 |
Polyurethane |
−42 |
450 to 370 |
0.53 |
— |
1.3 |
Most of the efficient NIR and far-red sensitized TTA-UC systems have used rubrene as a key annihilator and dibenzotetraphenylperiflanthene (DBP) as a singlet acceptor/emitter.85–87 Since rubrene satisfies the energetic condition for singlet fission i.e. S1(E) ≥ 2T1(E), the upconverted singlet photon undergo singlet fission before emission, which reduces the overall ϕ∞UC. DBP is used to avoid this loss channel via singlet energy transfer from rubrene to DBP because of its low-lying singlet state compared to rubrene (ΔES ≈ −0.14 eV).43,83 Eventually, DBP emit the upconverted photon of rubrene at low energy in the red-region. In such systems, the best ϕUC = 2.05% has been reported for Os(atpy)(tbbpy)Cl+-rubrene-DBP nanoparticles doped polyvinyl alcohol (PVA) film upon 938 nm excitation.85 Without DBP the best ϕUC = 3.65% has been reported in Pd-tetraphenyltetraanthroporphyrin (PdTPTAP)-rubrene emulsified mesoporous PVA films upon 810 nm excitation.88
For red light sensitized TTA-UC, thermally evaporated rubrene on palladium phthalocyanine (PdPc) doped polystyrene surface (ϕUC = 1.2%)47 and rubrene-DBP spin-coated on an atomically thin two-dimensional (2-D) layer of MoSe2 as sensitizer (ϕUC = 1.1%)87 were found to be the most efficient. Incidentally, most efficient solid-state TTA-UC system have been reported for Vis-to-Vis TTA-UC.32,89–93 It could be due to the combined effect of high statistical probabilities of the annihilators, small anti-Stokes shifts, and suitable orientations of sensitizer–annihilator pairs for TET and later triplet coupling in solid-state. For example, a high ϕUC = 15% in air has been reported for Pd(II)-octa-butoxy phthalocyanine Pd(OBu)8Pc-rubrene nanoemulsion doped polyacrylate films upon red light (λex = 670 nm) excitation.90 This is despite low f value of rubrene. In the same framework the green light (λex = 543 nm) sensitized palladium octaethylporphyrin (PdOEP)-diphenylanthracene (DPA) film showed ϕUC = 16%.90 This is due to the higher molecular diffusion of the chromophores inside the liquid core of nanoemulsions that fosters high TET and effective inter-triplet coupling to populate the singlet state.
However, synthetic polymers as a matrix may not be a sustainable solution for a long period due to non-recyclability. Hence, an alternative recyclable matrix is desired to fabricate an efficient TTA-UC system. Here, recyclable TTA-UC bioplastics, also operating via molecular diffusion mechanism could be a viable solution. For example, TTA-UC bioplastics comprised of chromophores doped viscous surfactant liquids dispersed inside the biopolymer matrix have shown high ϕUC = 8.8% for red to blue,32 and ϕUC = 7.8%31 for green to blue UC in air. Moreover, chromophores used in these bioplastics could be recycled via simple solid–liquid phase separation that enhances their sustainability factor.
Another way to avoid synthetic plastics is the air-stable TTA-UC crystal, which operates via triplet energy migration (TEM). It can be achieved via a suitable molecular design of chromophores and a crystallization approach. For example, molecular engineering of anthracene to 9-(2-naphthyl)-10-[4-(1-naphthyl)phenyl]anthracene (ANNP) and subsequent crystallization with platinum octa-ethyl porphyrin (PtOEP) and annealing yielded high-end results with ϕUC = 16.4%, Ith = 0.7 mW cm−2 in air.92 Similarly, co-crystallization of PtOEP with cyclic diphenylanthracene derivative, C7-sDPAm yielded ϕUC = 10% and Ith = 5 mW cm−2 in air.93 Fabrication of crystalline mono/bilayers of such TTA-UC crystals beneath the solar cells of suitable band gap can certainly enhance their power conversion efficiency (PCE) by manifold. Moreover, such molecular designs also inspire the engineering of annihilators capable of upconverting NIR light to increase the efficient upconversion width of TTA-UC crystals. But the longevity of TTA-UC in crystals needs to be established for practical integration with solar harvesting devices.
The commercial crystalline solar cell (c-Si), has a band gap of ≈1.1 eV,94 that is below the longest energy-converting solid-state TTA-UC system (1.32 eV).85 Therefore, the current TTA-UC systems are not suitable for integration with c-Si. Instead, they are suitable for integration with high band gap solar cells, which are currently at the development stage. However, efforts have been made in the past decade, with several proof-of-concept TTA-UC integrated high band gap PV devices. Therefore, in the next section, we will review these developments concerning the sensitizer–annihilator design, TTA-UC emission band gap, PV band gap, and device design.
The solid-state Vis-to-UV TTA-UC systems are rather more relevant to increase the efficiency of solar fuels, MOST systems95 or solid-state photoswitches.96 The upconversion to UV light can counter the low spectral irradiance of AM 1.5 solar spectrum in the UV region, which can be exploited to enhance the photoconversion quantum yield of photo isomers like norbornadiene, stilbene, dihydroazulene and azobenzene, etc.97,98 Recently, there has been a great surge in research on Vis-to-UV TTA-UC systems due to the discovery of TIPS-naphthalene.79 However, the most efficient systems (ϕUC = 10% to 17%)36,79 are limited to degassed organic solutions that are mainly exploited for photo-catalysis applications.99 However, if such high efficiency Vis to UV TTA-UC could be translated to solid-state it would create opportunities in several applications. Surprisingly, there is a big void in solid-state Vis to UV TTA-UC research. And the most efficient system (ϕUC = 1.2%) was last reported in 2016 in the form of Ir(ppy-DBP)-2,7-di-tert-butylpyrene (DBP) doped polyurethane films.100 Therefore, in the coming section we will also discuss implications of solid-state Vis to UV TTA-UC systems and our perspective for future directions.
TTA-UC driven photovoltaic devices
Photon upconversion can overcome the transmission losses in PV devices, but practically that is needed to happen near the solar irradiance or one sun ≈100 mW cm−2 of AM 1.5 solar spectrum. That is where TTA-UC which functions efficiently around solar irradiance is useful compared to other UC processes like energy transfer upconversion (ETU), excited state absorption (ESA), and photon avalanche (PA), etc. Most of the earliest TTA-UC systems were efficient in the deaerated organic solvents. As a result, they were tested as a proof-of-concept for photocurrent enhancement of high band gap solar cells. The summary of this is provided in Table 2.
Table 2 Photocurrent enhancement in UC solution integrated solar cells. UC emission bandwidth, solar cell bandgap, excitation wavelength (λex), excitation power density (Iex) and short circuit current density due to UC (ΔJsc). Here 1 sun of 670 nm laser ≈3.89 mW cm−2 and 720 nm laser ≈9.81 mW cm−2
Sensitizer–annihilator (UC solution) |
UC emission bandwidth (eV) |
Solar cell |
Bandgap (eV) |
λ
ex (laser) (nm) |
Sun |
ΔJsc (mA cm−2) |
PQ4Pd-rubrene101 |
2.25–2.06 |
a-Si:H |
1.7–1.8 |
670 |
1 |
0.00042 |
PQ4PdNA-rubrene101 |
2.25–2.06 |
a-Si:H |
1.7–1.8 |
720 |
1 |
0.0125 |
PQ4PdNA-rubrene102 |
2.25–2.06 |
a-Si:H |
1.7–1.8 |
720 |
1 |
0.035 |
PQ4PdNA-rubrene103 |
2.25–2.06 |
a-Si:H |
1.7–1.8 |
670 |
1 |
0.0144 |
PQ4PdNA-rubrene103 |
2.25–2.06 |
P3HT : ICBA |
2.1 : 1.72 |
670 |
1 |
0.0028 |
PQ4PdNA-rubrene104 |
2.25–2.06 |
a-Si:H |
1.7–1.8 |
670 |
1 |
0.0075 |
PQ4PdNA-rubrene105 |
2.25–2.06 |
D149/TiO2 |
2.43/3.2 |
670 |
1 |
0.0037 |
PQ4PdNA-rubrene-BPEA105 |
2.25–2.06 |
a-Si:H |
1.7–1.8 |
670 |
1 |
0.036 |
PQ4PdNA-rubrene-BPEA105 |
2.25–2.06 |
D149/TiO2 |
2.43/3.2 |
670 |
1 |
0.068 |
PQ4PdNA-rubrene24 |
2.25–2.06 |
D149/TiO2 |
2.43/3.2 |
670 |
1 |
0.0046 |
Schmidt's research group contributed to the earliest developments of TTA-UC-PV devices with high band gap (1.7 to 3.2 eV) solar cells (a-Si:H) (p–i–n), P3HT:ICBA (OPV), D149/TiO2 (DSSC)24,101–103 and worked on the device design to improve the short circuit current density (Jsc) and upconversion figure of merit (ζ). For this they mainly used TTA-UC solution of PQ4PdNA/rubrene, that was placed behind the solar cells to capture the back-reflected UC photons upon 670 or 720 nm laser excitation. This is because rubrene's UC emission (550–600 nm) corresponds to the band gap of the solar cells or the dye used in the case of dye-sensitized solar cells (DSSCs). The UC-enhanced short circuit current density (ΔJUCSC) of the solar cell was calculated using eqn (16).
|  | (16) |
where EQE is external quantum efficiency, which is the number of charge careers extracted from the solar cell per incident photons;
e is the elementary charge;
fc is the solar concentration factor;
ϕAM1.5G is solar flux in photons per unit area time per wavelength. The
ζ is calculated using
eqn (17) by dividing Δ
JUCsc with a square of
fc.
|  | (17) |
A typical device structure of TTA-UC integrated bifacial p–i–n, a-Si:H solar cell used by Schmidt's group is shown in
Fig. 7a.
101
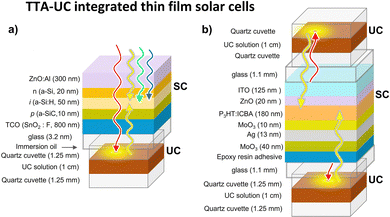 |
| Fig. 7 Illustration of the device structure of TTA-UC solution integrated thin film solar cell, (a) a-Si:H p–i–n solar cell and (b) P3HT:ICBA organic photovoltaic solar cell (OPV). The TTA-UC solution on both sides of OPV indicates that it can be placed either below the ITO cathode or below the Ag anode. Transmitted red light is injected back into the solar cell in the form of upconverted to yellow light. | |
In many papers device performance is expressed as ζ.24,101–103 But for clarity, in this paper, the current enhancement due to TTA-UC integration has been presented as change in short circuit current density only (ΔJsc). Other than changing the solar cells, they also worked on the optical design of the device to reflect a maximum number of UC photons toward the solar cell. For example, the use of silver-coated glass spheres or silver-coated PTFE as a back reflector led to a 3102 to 18104 fold enhancement in ΔJsc (Table 2).101,102,104 Further, they reported around 5-fold enhancement of ΔJsc for p–i–n, a-Si:H by changing the thickness of a-Si:H layer to 150 nm and adding annihilator, 9,10-bis(phenylethynyl)anthracene (BPEA) in PQ4PdNA/rubrene solution to avoid parasitic triplet loss channel (Table 2).105
The solution state TTA-UC integrated solar cells gave useful early proof-of-concept insights for PV applications of TTA-UC. However, practical PV devices require their integration in the solid-state. Moreover, they were based on the absorption of UC photons by the semiconductor or dye to increase the photocurrent. Schmidt and co-workers106 showed that the singlet state of anthracene could also act as an electron donor to the conduction band of semiconductor (TiO2) in the DSSC setup. It is due to the suitable oxidation potential of anthracene. They demonstrated a heterogenous DSSC device design where an annihilator, 4,40-(anthracene-9,10-diylbis(ethyne-2,1-diyl) dibenzoic acid) (BDCA) was adsorbed on the surface of a semiconductor (TiO2) and sensitizer platinum(II) tetrabenzotetraphenylporphyrin (PtTBTPP) was dissolved in the electrolyte solution along with Co2+/CO3+ as a redox mediator. A ΔJUC = 0.11 μA cm−2 of could be achieved for this device upon excitation with AM 1.5 spectrum between 560–640 nm (Fig. 8a).106
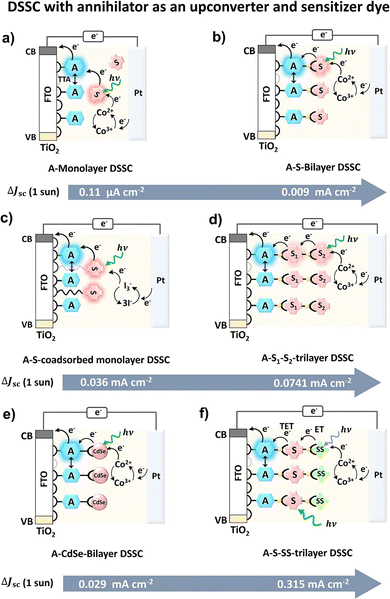 |
| Fig. 8 (a–f) Illustration of the development in TTA-UC integrated DSSC where an annihilator acts as an electron donor dye to the semiconductor (TiO2), and Co2+/CO3+ or I3−/I− as redox mediator. A = annihilator, S = triplet sensitizer, CdSe = quantum dot sensitizer, and SS = singlet sensitizer. | |
Hanson's research group went one step further and devised a fully solid-state TTA-UC integrated DSSC in the form of annihilator–sensitizer bilayers linked with Zn ion and fabricated on TiO2 or ZrO2 semiconductors.107–109 The 4,4′-(anthracene-9,10-diyl) bis(4,1-phenylene)diphosphonic acid (DPPA) and Pt(II) tetrakis(4-carboxyphenyl)porphyrin (PtTCPP) were fabricated on to TiO2 or ZrO2 with a spatiotemporal control as anode electrode. The upconverted singlet state of DPPA injected the electron to the conduction band of TiO2 to start the electron flow to induce photocurrent upon absorption of the green light of solar spectrum by the PtTCPP and Co-(bpy)3(PF6)2 as redox mediator to this device. The resulting device showed Jsc = 0.009 ± 0.002 mA cm−2 upon one sun excitation of AM 1.5 solar spectrum (Fig. 8b).108 To further broaden the absorption range of the solar spectrum they introduced a dual sensitizer approach i.e. TiO2-DPPA-PdTCPP-PtTCPP trilayer (Fig. 8d). Due to higher photon absorption by the sensitizers, the Jsc = 0.0741 mA cm−2 was recorded under one sun excitation of AM 1.5 solar spectrum.109 Recently, they reported S0 to T1 sensitizer, carboxylated osmium(II) polypyridyl (Os) and phosphonated bis(9,10-diphenylethynyl)anthracene annihilator (PBPEA) based DSSC to harvest NIR photons up to 950 nm. The TiO2-PBPEA-Zn-Os bilayered DSSC with I3−/3I− as redox mediator showed ΔJsc ∼ 3.5 μA cm−2 under AM 1.5 solar excitation.110 The low ΔJsc despite broad absorption range was attributed to slow sensitizer to annihilator triplet energy transfer, a low injection yield for the annihilator, and fast back energy transfer from the upconverted state to the sensitizer.110 To avoid the sensitizer's aggregation on the annihilator-TiO2 film Nagata's research group co-adsorbed the sensitizer (PtTPO) on TiO2 with a long alkyl chain spacer (Fig. 8c). The TiO2-4,4′-(anthracene-9,10-diyl)bis(4,1-phenylene)dicarboxylic acid (ADDA)-PtTPO co-adsorbed film showed ΔJsc = 0.036 mA cm−2 upon 1 sun excitation with simulated AM 1.5G radiation.111
Schmidt et al. reviewed these developments in 2017 and suggested ΔJsc = 0.0741 mA cm−2 still short of device-relevant figure of merit i.e., ΔJsc = 0.1 mA cm−2 under 1 sun excitation.112 They suggested a roadmap to achieve the ΔJsc ≥ 0.1 mA cm−2 by improving the device design, transparency of solar cell, solid-state upconverter, broadening of sensitizers absorption cross-section by new sensitization approach like semiconductors quantum dots, etc.
Semiconductor quantum dots are emerging as new triplet sensitizers due to their size and tunable spectrum because of the quantum confinement effect.113 The delocalization of their frontier molecular orbital over several nanometres results in a small singlet–triplet energy gap. Due to the long-lived triplet energy on the surface of the nanocrystals, it can be transferred to the surface-attached annihilator/acceptor via electron exchange. The annihilator can then transfer energy to the semiconductor of the solar cell. Hanson's research group explored this possibility and fabricated CdSe quantum dots over the TiO2-DPPA surface as a photoanode (Fig. 8e).114 The resulting DSSC showed ΔJsc = 0.029 mA cm−2 upon 1 sun excitation with simulated AM 1.5G radiation. However, the obtained ΔJsc is lower than that of DSSC with molecular sensitizer, due to the multiple factors.
For example, (1) lower sensitizer surface loadings, (2) low triplet energy transfer yield (∼40–80%), (3) slow regeneration kinetics and (4) competitive quenching of triplet excited CdSe by the Co(II)/Co(III)(phen)3 redox mediator. In an interesting development, Hanson and co-workers exploited Monguzzi's singlet sensitization approach to increase TTA-UC quantum yield to increase ΔJsc of DSSC. The singlet sensitizer absorbs the photons in the transparency window between the sensitizer and annihilator and transfers the energy to the triplet sensitizer to increase the absorption cross-section. Fluorescein was used as a singlet sensitizer (SS) in a tri-layered TiO2-DPPA-PtCCP photoanode (Fig. 8f).115 The resulting device showed a record ΔJsc = 0.315 mA cm−2 under 1 sun.
Other than the annihilator as an electron donor, conventional DSSC with a separate electron donor dye with I3−/I− as a redox mediator was also integrated with both TTA-UC solution and solid-state film (Fig. 9a and b).24,116
To harvest the maximum photon an Al2O3 reflector was placed behind the TTA-UC solution or film in these devices. The upconverted photons emitted by the annihilator were absorbed by the dye that transferred the electron to the TiO2 conduction band. It is due to the overlap of the absorption spectrum of dye with the emission spectrum of the annihilator. For example, the TTA-UC solution (rubrene/PQ4PdNA) integrated DSSC with D149 as an electron donor dye and rear reflector showed ΔJsc = 0.0046 mA cm−2 at 1 sun excitation of 670 nm laser (Fig. 9a).24 They suggested poor device design for low ΔJsc. However, integration with TTA-UC film (perylene/PdTPBP doped polyurethane) with TiO2-D131 dye as electron donor showed enhancement of ΔJsc = 1.65 mA cm−2 under 7 mW cm−2 (1 sun) of 635 nm laser excitation (Fig. 9b).116 They attributed enhancement to better device design with a background reflector, solid-state upconverter, and higher TTA-UC quantum yield. This number fits well into the device relevant ΔJsc = 0.1 mA cm−2.
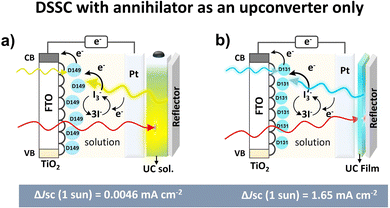 |
| Fig. 9 (a and b) Illustration of the development of the solution to solid-state TTA-UC integrated DSSC where UC photons of annihilator are absorbed by dye (D149 or D131) that acts as a sensitizer dye to inject an electron into the conduction band of a semiconductor. | |
Other than thin film and DSSC, metal halide perovskite-based solar cells (PVSCs) are fast emerging as a cheap and sustainable alternative to c-Si solar cells.117 This is due to their exceptional properties like high extinction coefficient in the visible spectrum, the large diffusion length of charge carriers, and long excited state lifetimes.118 As a result, PVSCs have witnessed solar power conversion efficiency of 25% in a decade.117,119 However, it is still lower than the Shockley–Queisser limit.8 It is due to many factors, including crystal packing of perovskites, oxygen quenching, absorption limited to the visible region, etc. Hence, the upconversion of NIR photons by integration with TTA-UC materials can increase their efficiency. Since the maximum upconversion wavelength of TTA-UC materials is limited to 1140 nm, they are suitable for integration with PVSCs having bandgap in the visible region.21,120 Kimizuka, Yanai, and co-workers, integrated PVSC's Cs0.05FA0.54MA0.41Pb(I0.98Br0.02)3 with Os(atpy)(tbbpy)Cl+-rubrene-DBP doped PVA TTA-UC film with spiro-OMeTAD as a hole transport layer (Fig. 10a). TTA-UC integrated PVSC showed quadratic dependence of ΔJsc with the 938 nm laser excitation arising due to the absorption of UC photons by the perovskite solar cell (Fig. 10a).85
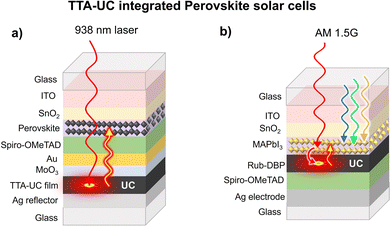 |
| Fig. 10 Illustration of the TTA-UC integrated perovskite solar cells. (a) Perovskites Cs0.05FA0.54MA0.41Pb(I0.98Br0.02)3 as semiconductor absorber integrated to Os(atpy)(tbbpy)Cl+-rubrene-DBP doped PVA TTA-UC film. (b) Perovskites, MAPbI3 as triplet sensitizer and semiconductor absorber crystallized on rubrene-DBP layer. | |
They observed ΔJsc = 0.1 mA cm−2 but at very high excitation intensity (4 W cm−2).
Further working in this direction, Tan, Chen, and co-workers, reported an enhancement of ΔJsc = 2.38 mA cm−2 of MAPbI3 solar cell fabricated on rubrene-DBP layers at AM 1.5G illumination (Fig. 10b).121 Unlike the other TTA-UC integrated solar cell devices, they deposited TTA-UC layers between the electron transport layer (SnO2) and hole transport layer (Spiro-OMeTAD). Interestingly, they suggested the MAPbI3 layer acting both as a triplet sensitizer and solar cell. However, the exclusive contribution of TTA-UC to the total ΔJsc enhancement is still disputed for this solar cell. It is because, the rubrene-DBP doping could have promoted perovskite growth owing to the formation of π-conjugated agglomerates, that served as suitable nuclei for better perovskite crystal packing. Hence, such a large enhancement in ΔJsc can be partly attributed to better crystal packing of perovskite leading to enhanced ΔJsc.
To shed doubts over the exclusive contribution of TTA-UC in ΔJsc Tan, Chen, and co-workers reported a dual sensitization approach, where in addition to perovskite as sensitizer they doped the rubrene-DBP with NIR sensitizer, copper-2,3, 9,10,16,17,23,24-octa-fluoro phthalocyanine (F8CuPc). The resulting device (Fig. 11) showed enhancement of ΔJsc = 2.96 mA cm−2 compared to the reference perovskite film and ΔJsc = 1.14 mA cm−2 compared to the perovskite-rubrene-DBP film.122 Hence validating their claims. Additionally, the hydrophobic nature of F8CuPc acted as a moisture barrier against humidity.
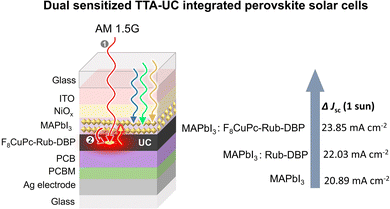 |
| Fig. 11 Illustration of the dual sensitized TTA-UC integrated perovskite solar cell and consequent ΔJsc enhancement. | |
While PVs can convert solar energy into electricity, storing electricity in PV modules for a long period has been an issue. Instead, the generated electricity can be stored in an electric battery bank. However, alternative technology, i.e. molecular solar thermal energy storage systems (MOST) can store solar energy in chemical bonds for a long period. The stored photon energy can be later released in the form of heat as per requirement. Hence, in the next section, we will discuss the potential of MOST and its implications with TTA-UC to increase the efficiency of storage.
Photochemical energy storage driven by TTA-UC
A typical MOST showing photo-thermal switching of Norbornadiene (NBD) ↔ Quadricyclane (QC) system is shown in Fig. 12a.123
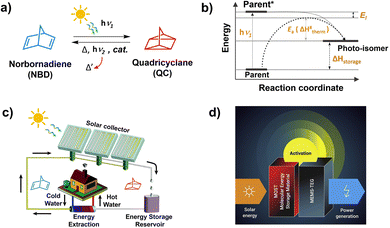 |
| Fig. 12 Illustration of (a) photothermal switching of norbornadiene–quadricyclane system, (b) energy diagram of photo-thermal switching, and application of MOST systems for (c) heating or cooling of homes and (d) electrical power generation. (b and c) is reproduced from ref. 17 with permission from the Royal Society of Chemistry. (d) is reproduced from ref. 18 with permission from Cell Press. | |
Here the parent NBD undergoes photoisomerization to QC upon absorption of photon energy (hv1). The QC stores the photon energy in chemical bonds and releases it as thermal energy (ΔHstorage) in a process of converting back to NBD activated by light (hv2), heat, or catalyst (Fig. 12a and b). The energy stored by photo-isomer can primarily be used for; (1) heating/cooling of houses in winter in temperate regions, and (2) electrical power generation upon integration with thermoelectric generators (Fig. 12c and d).17,18
Similar to PV devices, where solar cells have small bandgap limitations, the organic molecules used in MOST systems also have a small solar absorption window. Most of the organic molecules used in MOST systems absorb in the UVB-to-blue region which is a small part of the AM 1.5G solar spectrum and has a low spectral irradiance.124 Integration with TTA-UC materials can increase the solar flux due to the upconversion of a visible photon to UV photons (Fig. 13a). Hence, the Vis to UV TTA-UC materials are most relevant for MOST applications. However, not much work has been done in these directions due to many photophysical and device fabrication issues. Among the key issues is the overlap of the absorption/emission spectrum of the photo-switch with the sensitizer/annihilator pair. Therefore, the foremost challenge is to find suitable photoswitch/TTA-UC pair that does not interfere with each other. For example, in one such proof-of-concept demonstration, our research group integrated fulvalene diruthenium (FvRu2) derivative-based photothermal switch with palladium octa-ethyl porphyrin (PdOEP) and diphenyanthracene (DPA) based TTA-UC solution.125 The low energy FvRu2 has an absorption maximum at around 400 nm with a tail extending into the blue region that overlaps with the emission spectrum of DPA. Incidentally, the high-energy photo-isomers of FvRu2 do not absorb at all in the visible region.
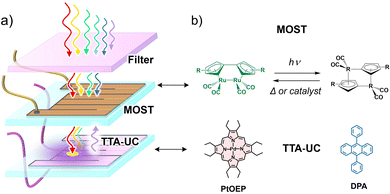 |
| Fig. 13 (a) Illustration of the microfluidic flow reactor comprising of TTA-UC fluidic chip integrated into molecular solar thermal energy storage fluidic chip (MOST). (b) Illustration of photothermal switching of fulvalene diruthenium derivative (green/black; R = 1,1-dimethyltridecyl) system (MOST), and molecular structures of the TTA-UC sensitizer (PdOEP) and annihilator (DPA). | |
Due to this optical transparency, the green photons could be upconverted to blue photons via TTA-UC using PdOEP as a sensitizer (Fig. 13b). Hence the conversion efficiency of FvRu2 could be increased by 130% due to the enhanced photon flux. Baluschev's research group has proposed the barium metal complexed Z isomers of crown ether functionalized hemicyanine dyes as a suitable candidate for photon energy storage upon E to Z photoisomerization with blue light excitation. To enhance the blue photon flux or shift the action spectrum toward a low energy, they integrated this system with a Perylene-PdTPBP-based TTA-UC solution that resulted in E–Z isomerization upon excitation with 635 nm laser (Fig. 14)126 However, they did not report back conversion of the Z isomer to release the stored photon energy. Such systems could become practically more relevant if designed in the solid-state (Fig. 12c and d). However, the small photon conversion quantum yields of these photos-witches are a limiting factor. Hence, photo-thermal switches with ϕC ∼ 100% could be a game changer.123 And specifically for TTA-UC integration there must be optical transparency for one of the photo isomer in the TTA-UC region.
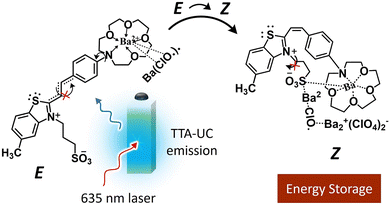 |
| Fig. 14 Illustration of the TTA-UC assisted E to Z isomerization of the barium complexed crown ether functionalized hemicyanine dyes upon 635 nm laser excitation. | |
Summary and future perspectives
The progress in TTA-UC enhanced solar energy conversion systems is on a slow rise. The actual reason for the slow progress is the requirement of transparent high band gap solar cell devices, which themselves are at the early developmental stage. Therefore, research on finding an efficient solid-state broadband TTA-UC upconverter and corresponding suitable band gap solar cells are moving side by side. Therefore, the majority of the earliest work has been done by integrating liquid upconversion solution below the thin film solar cells (a-Si:H, P3HT:ICBA).101–105 Later annihilator adsorbed on TiO2 as an electron donor dye in DSSCs,106–109,111,113–115 and liquid/solid upconverter integrated conventional DSSCs were developed.24,116 Among these systems a maximum short circuit current density, ΔJsc = 1.65 mA cm−2 has been recorded with solid-state photon upconverter (PdTPBP-perylene doped polyurethane film) upon 635 nm laser excitation equivalent to 1 sun.116 Well, it is greater than the threshold limit for PV's applications i.e. ΔJsc = 0.1 mA cm−2.112 Hence, it highlighted the importance of solid-state photon upconverters for PV devices. Of late, perovskites solar cells have been integrated with TTA-UC upconverter either by placing TTA-UC film below the solar cell85 or fabricating them between hole and electron transport layers.121,122 The latter device design has yielded a maximum ΔJsc enhancement of 2.96 mA cm−2 due to TTA-UC. Interestingly in addition to photon upconversion, TTA-UC layers assisted in better crystal packing of perovskites and improved moisture stability. This, show the importance of crystalline TTA-UC systems for PV integration to improve longevity. The performance could further be improved by co-crystallization of broad-band sensitizer–annihilator pairs for maximum harvesting of transmitted photons. Further development of efficient perovskite solar cells working above 1.62 eV in the visible region would exponentially increase their applications with efficient crystalline TTA-UC photon upconverters.89,92,93 But important consideration over here is the annihilator design with a high statistical probability factor in addition to triplet exciton diffusion length and lifetime in the crystalline state. We found that f is highly dependent on the emission wavelength and derivatization of the parent acene. However, in a suitable crystalline framework, it can be increased by recycling quintet and high-energy triplets via quintet–singlet spin mixing and reverse intersystem crossing.60
While PV technology is seen as a sustainable energy approach, commercial crystalline solar cells have a typical life of ≈25 years.127 In the past decade the cumulative PV capacity worldwide has boomed from 48 GW to 700 GW, which is also increasing the number of end-of-life (EOL) solar panels. Going by the current expansion of PV technology, the world will see ≈78 million tons of solar panel waste by 2050.128 In addition to the possible waste, the PV modules occupy a lot of space which adds to the cost. To address such issues, research on their recycling (down or upcycling) is already underway129,130 and alternative fabrication frameworks in the form of thin film plastic solar technologies are already in the market.131 But they may also face a similar fate regarding environmental waste since they are generally fabricated on polyester or polyimide sheets which are synthetic plastic.132 Therefore, replacing the fabrication material with robust biomaterial can enhance the sustainability factor since it could degrade in the natural environment after recycling chemicals at the EOL. We have demonstrated such a possibility by fabricating efficient broadband solid-state TTA-UC bioplastics using protein as an encapsulator to synthetic chromophores and their consequent recycling at the EOL.31,32 Such biopolymers can also be used to fabricate solar cell materials to replace polyester or polyimide sheets. For example, most earth-abundant biopolymers like cellulose and chitosan possess suitable dielectric and mechanical properties as that of polyester required for solar cells.133–135 Moreover, coating these biopolymers with Nylon 11 which is a natural polyimide derived from castor beans can decrease the water vapor permeability. For example, recently Gao et al. used flexible nanocellulose coated with acrylic resin as a back-sheet substrate for the fabrication of perovskite solar cells that showed a power conversion efficiency of 4.25%.136 We believe that the fabrication of more such bio-fabricated solar cells and further integration with TTA-UC bioplastics would pave the way for future bioplastic solar cells that our research group is committed to achieving.
Regarding TTA-UC integrated MOST systems, there has not been great progress due to many spectral factors related to both photo-switch and TTA-UC systems. Over the years our research group has developed various MOST devices with several NBD, azobenzene, and dihydroazulene derivatives-based photo-switches to harvest maximum solar photons.123,137 The NBD ↔ QC system has a high ϕc in addition to the transparent visible spectral window beyond 400 nm that could be utilized for integration with an efficient Vis to UV TTA-UC system. Because NBD and its derivatives typically absorbs between 320 to 450 nm, its photo-isomerization yield can be increase by increasing solar flux in this region by upconverting 400–550 nm light using suitable sensitizer–annihilator pair. However, low UC efficiency of Vis to UV systems over the years has been a big obstacle that has now been sorted with the discovery of highly efficient TIPS-naphthalene based TTA-UC systems near the sub-solar irradiance.36,79 The TIPS-naphthalene emits between 300–400 nm which is in the absorption range of NBD. Hence are suitable for efficiency enhancement of the NBD ↔ QC systems in ambient conditions. However, such systems need to be developed in the solid-state for practical fabrication with the solid-state MOST devices, which our research group is committed to achieving.
Author contributions
Lukas Naimovičius, Pankaj Bharmoria, and Kasper Moth-Poulsen conceptualized the idea, wrote the first draft, revised, and edited the final draft of this manuscript.
Conflicts of interest
There are no conflicts to declare.
Acknowledgements
L. N. acknowledges Erasmus+ Traineeship Program, P. B. acknowledges La-Caixa junior research leadership-post doctoral program (ID: 100010434, fellowship code: LCF/BQ/P122/11910023). K. M. P. acknowledges funding from the European Research Council (No. 101002131), the Swedish Energy Agency, the Göran Gustafsson Foundation, the Swedish Research Council, Swedish Research Council Formas, the European Research Council (ERC) under grant agreement CoG, PHOTHERM – 101002131, the Catalan Institute of Advanced Studies (ICREA), and the European Union's Horizon 2020 Framework Programme under grant agreement no. 951801.
Notes and references
- R. E. Blankenship, Early Evolution of Photosynthesis, Plant Physiol., 2010, 154, 434–438 CrossRef CAS PubMed
.
-
M. E. Hannibal, The history and future of fire, 2021, vol. 374 Search PubMed
.
- M. Buchanan, Energy transitions, Nat. Phys., 2017, 13, 1144 Search PubMed
.
-
R. A. Kerr, Humans Fueled Global Warming Millennia Ago, 2013, vol. 342 Search PubMed
.
-
G. Austin and K. Sugihara, Labour-Intensive Industrialization in Global History, Taylor & Francis, 2013 Search PubMed
.
- Paris Agreement, United Nations Treaty Collect., 2016, XXVII.7.d.
- M. Green, E. Dunlop, J. Hohl-Ebinger, M. Yoshita, N. Kopidakis and X. Hao, Solar cell efficiency tables (version 57), Prog. Photovoltaics Res. Appl., 2021, 29, 3–15 Search PubMed
.
- W. Shockley and H. J. Queisser, Detailed balance limit of efficiency of p–n junction solar cells, J. Appl. Phys., 1961, 32, 510–519 CrossRef CAS
.
- A. Shah, P. Torres, R. Tscharner, N. Wyrsch and H. Keppner, Photovoltaic technology: the case for thin-film solar cells, Science, 1999, 285, 692–698 CrossRef CAS PubMed
.
- M. D. McGehee, Paradigm shifts in dye-sensitized solar cells, Science, 2011, 334, 607–608 CrossRef CAS PubMed
.
- A. B. Muñoz-García, I. Benesperi, G. Boschloo, J. J. Concepcion, J. H. Delcamp, E. A. Gibson, G. J. Meyer, M. Pavone, H. Pettersson, A. Hagfeldt and M. Freitag, Dye-sensitized solar cells strike back, Chem. Soc. Rev., 2021, 50, 12450–12550 RSC
.
- F. Dimroth, T. N. D. Tibbits, M. Niemeyer, F. Predan, P. Beutel, C. Karcher, E. Oliva, G. Siefer, D. Lackner, P. Fuß-Kailuweit, A. W. Bett, R. Krause, C. Drazek, E. Guiot, J. Wasselin, A. Tauzin and T. Signamarcheix, Four-Junction Wafer-Bonded Concentrator Solar Cells, IEEE J. Photovoltaics, 2016, 6, 343–349 Search PubMed
.
- E. J. Juarez-Perez and M. Haro, Perovskite solar cells take a step forward, Science, 2020, 368, 1309 CrossRef CAS PubMed
.
- A. Ghazy, M. Safdar, M. Lastusaari, H. Savin and M. Karppinen, Advances in upconversion enhanced solar cell performance, Sol. Energy Mater. Sol. Cells, 2021, 230, 111234 CrossRef CAS
.
- A. J. Carrod, V. Gray and K. Börjesson, Recent advances in triplet–triplet annihilation upconversion and singlet fission, towards solar energy applications, Energy Environ. Sci., 2022, 15, 4982–5016 RSC
.
- J. Pedrini and A. Monguzzi, Recent advances in the application triplet–triplet annihilation-based photon upconversion systems to solar technologies, J. Photonics Energy, 2017, 8, 1 Search PubMed
.
- Z. Wang, A. Roffey, R. Losantos, A. Lennartson, M. Jevric, A. U. Petersen, M. Quant, A. Dreos, X. Wen, D. Sampedro, K. Börjesson and K. Moth-Poulsen, Macroscopic heat release in a molecular solar thermal energy storage system, Energy Environ. Sci., 2019, 12, 187–193 RSC
.
- Z. Wang, Z. Wu, Z. Hu, J. O. Hernández, E. Mu, Z. Y. Zhang, M. Jevric, Y. Liu, X. Fu, F. Wang, T. Li and K. Moth-Poulsen, Chip-scale solar thermal electrical power generation, Cell Rep. Phys. Sci., 2022, 3, 100789 CrossRef CAS
.
- C. A. Parker and C. G. Hatchard, Delayed fluorescence from solutions of anthracene and phenanthrene, Proc. R. Soc. London, Ser. A, 1962, 269, 574–584 Search PubMed
.
- P. E. Keivanidis, S. Baluschev, T. Miteva, G. Nelles, U. Scherf, A. Yasuda and G. Wegner, Up-Conversion Photoluminescence in Polyfluorene Doped with Metal(II)-Octaethyl Porphyrins, Adv. Mater., 2003, 15, 2095–2098 CrossRef CAS
.
- P. Bharmoria, H. Bildirir and K. Moth-Poulsen, Triplet–triplet annihilation based near infrared to visible molecular photon upconversion, Chem. Soc. Rev., 2020, 49, 6529–6554 RSC
.
- V. Gray, K. Moth-Poulsen, B. Albinsson and M. Abrahamsson, Towards efficient solid-state triplet–triplet annihilation based photon upconversion: supramolecular, macromolecular and self-assembled systems, Coord. Chem. Rev., 2018, 362, 54–71 CrossRef CAS
.
- Y. Sasaki, S. Amemori, N. Yanai and N. Kimizuka, Singlet-to-Triplet Absorption for Near-Infrared-to-Visible Photon Upconversion, Bull. Chem. Soc. Jpn., 2021, 94, 1760–1768 CrossRef CAS
.
- A. Nattestad, Y. Y. Cheng, R. W. Macqueen, T. F. Schulze, F. W. Thompson, A. J. Mozer, B. Fückel, T. Khoury, M. J. Crossley, K. Lips, G. G. Wallace and T. W. Schmidt, Dye-sensitized solar cell with integrated triplet–triplet annihilation upconversion system, J. Phys. Chem. Lett., 2013, 4, 2073–2078 CrossRef CAS PubMed
.
- S. Baluschev, V. Yakutkin, T. Miteva, Y. Avlasevich, S. Chernov, S. Aleshchenkov, G. Nelles, A. Cheprakov, A. Yasuda, K. Müllen and G. Wegner, Blue-green up-conversion: noncoherent excitation by NIR light, Angew. Chem., Int. Ed., 2007, 46, 7693–7696 CrossRef CAS PubMed
.
- Y. Zhou, F. N. Castellano, T. W. Schmidt and K. Hanson, On the Quantum Yield of Photon Upconversion via Triplet–Triplet Annihilation, ACS Energy Lett., 2020, 5, 2322–2326 CrossRef CAS
.
- D. L. Dexter, A theory of sensitized luminescence in solids, J. Chem. Phys., 1953, 21, 836–850 CrossRef CAS
.
- U. Resch-Genger and K. Rurack, Determination of the photoluminescence quantum yield of dilute dye solutions (IUPAC Technical Report), Pure Appl. Chem., 2013, 85, 2005–2026 CrossRef CAS
.
- P. Bharmoria, S. Hisamitsu, H. Nagatomi, T. Ogawa, M. A. Morikawa, N. Yanai and N. Kimizuka, Simple and Versatile Platform for Air-Tolerant Photon Upconverting Hydrogels by Biopolymer-Surfactant-Chromophore Co-assembly, J. Am. Chem. Soc., 2018, 140, 10848–10855 CrossRef CAS PubMed
.
- N. Yanai, K. Suzuki, T. Ogawa, Y. Sasaki, N. Harada and N. Kimizuka, Absolute Method to Certify Quantum Yields of Photon Upconversion via Triplet–Triplet Annihilation, J. Phys. Chem. A, 2019, 123, 10197–10203 CrossRef CAS PubMed
.
- P. Bharmoria, S. Hisamitsu, Y. Sasaki, T. S. Kang, M. A. Morikawa, B. Joarder, K. Moth-Poulsen, H. Bildirir, A. Mårtensson, N. Yanai and N. Kimizuka, Photon upconverting bioplastics with high efficiency and in-air durability, J. Mater. Chem. C, 2021, 9, 11655–11661 RSC
.
- P. Bharmoria, F. Edhborg, H. Bildirir, Y. Sasaki, S. Ghasemi, A. Mårtensson, N. Yanai, N. Kimizuka, B. Albinsson, K. Börjesson and K. Moth-Poulsen, Recyclable optical bioplastics platform for solid state red light harvesting via triplet–triplet annihilation photon upconversion, J. Mater. Chem. A, 2022, 10, 21181–21752 RSC
.
- A. M. Brouwer, Standards for photoluminescence quantum yield measurements in solution (IUPAC technical report), Pure Appl. Chem., 2011, 83, 2213–2228 CrossRef CAS
.
- L. B. Josefsen and R. W. Boyle, Photodynamic Therapy and the Development of Metal-Based Photosensitisers, Met.-Based Drugs, 2008, 2008, 276109 Search PubMed
.
-
Z. Mahmood, S. Ji, J. Zhao, M. Hussain, F. Sadiq, N. Rehmat and M. Imran, in Emerging Strategies to Reduce Transmission and Thermalization Losses in Solar Cells: Redefining the Limits of Solar Power Conversion Efficiency, ed. J. S. Lissau and M. Madsen, Springer International Publishing, Cham, 2022, pp. 71–105 Search PubMed
.
- A. Olesund, J. Johnsson, F. Edhborg, S. Ghasemi, K. Moth-Poulsen and B. Albinsson, Approaching the Spin-Statistical Limit in Visible-to-Ultraviolet Photon Upconversion, J. Am. Chem. Soc., 2022, 144, 3706–3716 CrossRef CAS PubMed
.
- E. Radiunas, S. Raišys, S. Juršenas, A. Jozeliunaite, T. Javorskis, U. Šinkeviciute, E. Orentas and K. Kazlauskas, Understanding the limitations of NIR-to-visible photon upconversion in phthalocyanine-sensitized rubrene systems, J. Mater. Chem. C, 2020, 8, 5525–5534 RSC
.
- R. Haruki, Y. Sasaki, K. Masutani, N. Yanai and N. Kimizuka, Leaping across the visible range: near-infrared-to-violet photon upconversion employing a silyl-substituted anthracene, Chem. Commun., 2020, 56, 7017–7020 RSC
.
- N. Yanai and N. Kimizuka, New Triplet Sensitization Routes for Photon Upconversion: Thermally Activated Delayed Fluorescence Molecules, Inorganic Nanocrystals, and Singlet-to-Triplet Absorption, Acc. Chem. Res., 2017, 50, 2487–2495 CrossRef CAS PubMed
.
- Y. Wei, K. Pan, X. Cao, Y. Li, X. Zhou and C. Yang, Multiple Resonance Thermally Activated Delayed Fluorescence Sensitizers Enable Green-to-Ultraviolet Photon Upconversion: Application in Photochemical Transformations, CCS Chem., 2022, 4, 3852–3863 CrossRef CAS
.
- E. Bassan, A. Gualandi, P. G. Cozzi and P. Ceroni, Design of BODIPY dyes as triplet photosensitizers: electronic properties tailored for solar energy conversion, photoredox catalysis and photodynamic therapy, Chem. Sci., 2021, 12, 6607–6628 RSC
.
- X. Lin, Z. Chen, Y. Han, C. Nie, P. Xia, S. He, J. Li and K. Wu, ZnSe/ZnS Core/Shell Quantum Dots as Triplet Sensitizers toward Visible-to-Ultraviolet B Photon Upconversion, ACS Energy Lett., 2022, 7, 914–919 CrossRef CAS
.
- M. Wu, D. N. Congreve, M. W. B. Wilson, J. Jean, N. Geva, M. Welborn, T. Van Voorhis, V. Bulovic, M. G. Bawendi and M. A. Baldo, Solid-state infrared-to-visible upconversion sensitized by colloidal nanocrystals, Nat. Photonics, 2016, 10, 31–34 CrossRef CAS
.
- M. Mahboub, Z. Huang and M. L. Tang, Efficient Infrared-to-Visible Upconversion with Subsolar Irradiance, Nano Lett., 2016, 16, 7169–7175 CrossRef CAS PubMed
.
- Z. A. Vanorman and L. Nienhaus, Bulk Metal Halide Perovskites as Triplet Sensitizers: Taking Charge of Upconversion, ACS Energy Lett., 2021, 6, 3686–3694 CrossRef CAS
.
- A. J. Carrod, A. Cravcenco, C. Ye and K. Börjesson, Modulating TTA efficiency through control of high energy triplet states, J. Mater. Chem. C, 2022, 10, 4923–4928 RSC
.
- E. Radiunas, L. Naimovičius, S. Raišys, A. Jozeliūnaitė, E. Orentas and K. Kazlauskas, Efficient NIR-to-vis photon upconversion in binary rubrene films deposited by simplified thermal evaporation, J. Mater. Chem. C, 2022, 10, 6314–6322 RSC
.
- F. Edhborg, H. Bildirir, P. Bharmoria, K. Moth-Poulsen and B. Albinsson, Intramolecular Triplet–Triplet Annihilation Photon Upconversion in Diffusionally Restricted Anthracene Polymer, J. Phys. Chem. B, 2021, 125, 6255–6263 CrossRef CAS PubMed
.
- A. Olesund, V. Gray, J. Mårtensson and B. Albinsson, Diphenylanthracene Dimers for Triplet–Triplet Annihilation Photon Upconversion: Mechanistic Insights for Intramolecular Pathways and the Importance of Molecular Geometry, J. Am. Chem. Soc., 2021, 143, 5745–5754 CrossRef CAS PubMed
.
- D. G. Congrave, B. H. Drummond, V. Gray, A. D. Bond, A. Rao, R. H. Friend and H. Bronstein, Suppressing aggregation induced quenching in anthracene based conjugated polymers, Polym. Chem., 2021, 12, 1830–1836 RSC
.
- E. M. Rigsby, T. Miyashita, D. A. Fishman, S. T. Roberts and M. L. Tang, CdSe nanocrystal sensitized photon upconverting film, RSC Adv., 2021, 11, 31042–31046 RSC
.
- N. A. Durandin, J. Isokuortti, A. Efimov, E. Vuorimaa-Laukkanen, N. V. Tkachenko and T. Laaksonen, Critical Sensitizer Quality Attributes for Efficient Triplet–Triplet Annihilation Upconversion with Low Power Density Thresholds, J. Phys. Chem. C, 2019, 123, 22865–22872 CrossRef CAS
.
- Y. Murakami and K. Kamada, Kinetics of photon upconversion by triplet–triplet annihilation: a comprehensive tutorial, Phys. Chem. Chem. Phys., 2021, 23, 18268–18282 RSC
.
- A. Monguzzi, J. Mézyk, F. Scotognella, R. Tubino and F. Meinardi, Upconversion-induced fluorescence in multicomponent systems: steady-state excitation power threshold, Phys. Rev. B: Condens. Matter Mater. Phys., 2008, 78, 195112 CrossRef
.
- S. J. Jacobs, D. A. Shultz, R. Jain, J. Novak and D. A. Dougherty, Evaluation of potential ferromagnetic coupling units: the bis(TMM) [bis(trimethylenemethane)] approach to high-spin organic molecules, J. Am. Chem. Soc., 1993, 115, 1744–1753 CrossRef CAS
.
- K. Itoh, Electronic structures of aromatic hydrocarbons with high spin multiplicities in the electronic ground state, Pure Appl. Chem., 1978, 50, 1251–1259 CAS
.
-
A. Köhler and H. Bassler, Bimolecular processes, Electronic processes inorganic semiconductors, Wiley-VCH, 2015, pp. 287–292 Search PubMed
.
- X. Wang, R. Tom, X. Liu, D. N. Congreve and N. Marom, An energetics perspective on why there are so few triplet–triplet annihilation emitters, J. Mater. Chem. C, 2020, 1, 10816–10824 RSC
.
- Y. Luo, K. Zhang, Z. Ding, P. Chen, X. Peng, Y. Zhao, K. Chen, C. Li, X. Zheng, Y. Huang, X. Pu, Y. Liu, S.-J. Su, X. Hou and Z. Lu, Ultra-fast triplet–triplet-annihilation-mediated high-lying reverse intersystem crossing triggered by participation of nπ*-featured excited states, Nat. Commun., 2022, 13, 6892 CrossRef CAS PubMed
.
- D.-G. Ha, R. Wan, C. A. Kim, T.-A. Lin, L. Yang, T. Van Voorhis, M. A. Baldo and M. Dincă, Exchange controlled triplet fusion in metal–organic frameworks, Nat. Mater., 2022, 21, 1275–1281 CrossRef CAS PubMed
.
- L. Naimovičius, E. Radiunas, B. Chatinovska, A. Jozeliūnaitė, E. Orentas and K. Kazlauskas, Functionalized diketopyrrolopyrrole compounds for NIR-to-visible photon upconversion, J. Mater. Chem. C, 2023, 11, 698–704 RSC
.
- D. Beljonne and A. Rao, Photon Upconversion from Near-Infrared to Blue Light with TIPS-Anthracene as an E ffi cient Triplet−Triplet Annihilator, ACS Mater. Lett., 2019, 1, 660–664 CrossRef
.
- A. Monguzzi, R. Tubino, S. Hoseinkhani, M. Campione and F. Meinardi, Low power, non-coherent sensitized photon up-conversion: modelling and perspectives, Phys. Chem. Chem. Phys., 2012, 14, 4322–4332 RSC
.
- X. Wang and N. Marom, An energetics assessment of benzo[a]tetracene and benzo[a]pyrene as triplet–triplet annihilation emitters, Mol. Syst. Des. Eng., 2022, 1, 11–13 Search PubMed
.
- S. Raišys, S. Juršėnas and K. Kazlauskas, Boost in Solid-State Photon Upconversion Efficiency through Combined Approach of Melt-Processing and Purification, Sol. RRL, 2022, 6, 1–9 CrossRef
.
- V. Gray, A. Dreos, P. Erhart, B. Albinsson, K. Moth-poulsen and M. Abrahamsson, Loss channels in triplet – triplet annihilation photon upconversion: importance of annihilator singlet and triplet surface shapes, Phys. Chem. Chem. Phys., 2017, 19, 10931–10939 RSC
.
- Q. Zhou, M. Zhou, Y. Wei, X. Zhou, S. Liu, S. Zhang and B. Zhang, Solvent effects on the triplet–triplet annihilation upconversion of diiodo-Bodipy and perylene, Phys. Chem. Chem. Phys., 2017, 19, 1516–1525 RSC
.
- S. Hoseinkhani, R. Tubino, F. Meinardi and A. Monguzzi, Achieving the photon up-conversion thermodynamic yield upper limit by sensitized triplet–triplet annihilation, Phys. Chem. Chem. Phys., 2015, 17, 4020–4024 RSC
.
- R. P. Groff, R. E. Merrifield, P. Avakian and E. Station, Singlet and triplet channels for triplet-exciton fusion in anthracene crystals, Chem. Phys. Lett., 1970, 5, 168–170 CrossRef CAS
.
- J. Fourny and G. Delacôte, Comparison of high and zero magnetic field values of non radiative triplet–triplet annihilation rate constants in crystalline anthracene, Chem. Phys. Lett., 1970, 8, 495–498 CrossRef
.
- V. Ern, J. L. Saint-Clair, M. Schott and G. Delacote, Effects of exciton interactions on the fluorescence yield of crystalline tetracene, Chem. Phys. Lett., 1971, 10, 287–290 CrossRef CAS
.
- D. G. Bossanyi, Y. Sasaki, S. Wang, D. Chekulaev, N. Kimizuka, N. Yanai and J. Clark, Spin Statistics for Triplet–Triplet Annihilation Upconversion: Exchange Coupling, Intermolecular Orientation, and Reverse Intersystem Crossing, JACS Au, 2021, 1, 2188–2201 CrossRef CAS PubMed
.
- Y. Y. Cheng, B. Fuckel, T. Khoury, R. G. C. R. Clady, M. J. Y. Tayebjee, N. J. Ekins-Daukes, M. J. Crossley and T. W. Schmidt, Kinetic Analysis of Photochemical Upconversion by Triplet–Triplet Annihilation: Beyond Any Spin Statistical Limit, J. Phys. Chem. Lett., 2010, 1, 1795–1799 CrossRef CAS
.
- P. Baronas, G. Kreiza, L. Naimovičius, E. Radiunas, K. Kazlauskas, E. Orentas and S. Juršėnas, Sweet Spot of Intermolecular Coupling in Crystalline Rubrene: Intermolecular Separation to Minimize Singlet Fission and Retain Triplet–Triplet Annihilation, J. Phys. Chem. C, 2022, 126, 15327–15335 CrossRef CAS PubMed
.
- R. Englman and J. Jortner, The energy gap law for radiationless transitions in large molecules, Mol. Phys., 1970, 18, 145–164 CrossRef CAS
.
- W. Siebrand and Radiationless Transitions, in Polyatomic Molecules. II. Triplet-Ground-State Transitions in Aromatic Hydrocarbons, J. Chem. Phys., 1967, 47, 2411–2422 CrossRef CAS
.
- W. Sun, A. Ronchi, T. Zhao, J. Han, A. Monguzzi and P. Duan, Highly efficient photon upconversion based on triplet–triplet annihilation from bichromophoric annihilators, J. Mater. Chem. C, 2021, 9, 14201–14208 RSC
.
- Y. Wei, Y. Li, Z. Li, X. Xu, X. Cao, X. Zhou and C. Yang, Efficient Triplet–Triplet Annihilation Upconversion in Solution and Hydrogel Enabled by an S–T Absorption Os(II) Complex Dyad with an Elongated Triplet Lifetime, Inorg. Chem., 2021, 60, 19001–19008 CrossRef CAS PubMed
.
- N. Harada, Y. Sasaki, M. Hosoyamada, N. Kimizuka and N. Yanai, Discovery of Key TIPS-Naphthalene for Efficient Visible-to-UV Photon Upconversion under Sunlight and Room Light, Angew. Chem., Int. Ed., 2021, 60, 142–147 CrossRef CAS PubMed
.
- B. Joarder, N. Yanai and N. Kimizuka, Solid-State Photon Upconversion Materials: Structural Integrity and Triplet-Singlet Dual Energy Migration, J. Phys. Chem. Lett., 2018, 9, 4613–4624 CrossRef CAS PubMed
.
- J. Alves, J. Feng, L. Nienhaus and T. W. Schmidt, Challenges, progress and prospects in solid state triplet fusion upconversion, J. Mater. Chem. C, 2022, 10, 7783–7798 RSC
.
- A. Abulikemu, Y. Sakagami, C. Heck, K. Kamada, H. Sotome, H. Miyasaka, D. Kuzuhara and H. Yamada, Solid-State, Near-Infrared to Visible Photon Upconversion via Triplet–Triplet
Annihilation of a Binary System Fabricated by Solution Casting, ACS Appl. Mater. Interfaces, 2019, 11, 20812–20819 CrossRef CAS PubMed
.
- E. Radiunas, M. Dapkevičius, S. Raišys, S. Juršenas, A. Jozeliunaite, T. Javorskis, U. Šinkevičiute, E. Orentas and K. Kazlauskas, Impact of T-butyl substitution in a rubrene emitter for solid state NIR-to-visible photon upconversion, Phys. Chem. Chem. Phys., 2020, 22, 7392–7403 RSC
.
- T. A. Lin, C. F. Perkinson and M. A. Baldo, Strategies for High-Performance Solid-State Triplet–Triplet-Annihilation-Based Photon Upconversion, Adv. Mater., 2020, 32, 1–7 Search PubMed
.
- M. Kinoshita, Y. Sasaki, S. Amemori, N. Harada, Z. Hu, Z. Liu, L. K. Ono, Y. Qi, N. Yanai and N. Kimizuka, Photon Upconverting Solid Films with Improved Efficiency for Endowing Perovskite Solar Cells with Near-Infrared Sensitivity, ChemPhotoChem, 2020, 4, 5271–5278 CrossRef CAS
.
- L. Nienhaus, M. Wu, N. Geva, J. J. Shepherd, M. W. B. Wilson, V. Bulović, T. Van Voorhis, M. A. Baldo and M. G. Bawendi, Speed Limit for Triplet-Exciton Transfer in Solid-State PbS Nanocrystal-Sensitized Photon Upconversion, ACS Nano, 2017, 11, 7848–7857 CrossRef CAS PubMed
.
- J. Duan, Y. Liu, Y. Zhang, Z. Chen, X. Xu, L. Ye, Z. Wang, Y. Yang, D. Zhang and H. Zhu, Efficient solid-state infrared-to-visible photon upconversion on atomically thin monolayer semiconductors, Sci. Adv., 2022, 8, 4935 CrossRef PubMed
.
-
S. M. Takeshi Mori, T. Mori, A. Saito, T. Masuda, H. Saomoto and M. Hagihara, High-Efficiency Near-Infrared-to-Visible Photon Upconversion in Poly(vinyl alcohol) Porous Film, ChemRxiv, 2022, preprint DOI:10.26434/chemrxiv-2022-mjjxb-v2.
- L. Li, Y. Zeng, J. Chen, T. Yu, R. Hu, G. Yang and Y. Li, Thermally Activated Delayed Fluorescence via Triplet Fusion, J. Phys. Chem. Lett., 2019, 10, 6239–6245 CrossRef CAS PubMed
.
- R. Vadrucci, A. Monguzzi, F. Saenz, B. D. Wilts, Y. C. Simon and C. Weder, Nanodroplet-Containing Polymers for Efficient Low-Power Light Upconversion, Adv. Mater., 2017, 29, 1–8 CrossRef PubMed
.
- J. Kim, F. Deng, F. N. Castellano and J. Kim, High Efficiency Low-Power Upconverting Soft Materials, Chem. Mater., 2012, 24, 2250–2252 CrossRef CAS
.
- R. Enomoto, M. Hoshi, H. Oyama, H. Agata, S. Kurokawa, H. Kuma, H. Uekusa and Y. Murakami, van der Waals solid solution crystals for highly efficient in-air photon upconversion under subsolar irradiance, Mater. Horiz., 2021, 8, 3449–3456 RSC
.
- K. Kamada, Y. Sakagami, T. Mizokuro, Y. Fujiwara, K. Kobayashi, K. Narushima, S. Hirata and M. Vacha, Efficient triplet–triplet annihilation upconversion in binary crystalline solids fabricated: Via solution casting and operated in air, Mater. Horiz., 2017, 4, 83–87 RSC
.
- C. Battaglia, A. Cuevas and S. De, Wolf, High-efficiency crystalline silicon solar cells: status and perspectives, Energy Environ. Sci., 2016, 9, 1552–1576 RSC
.
- K. Börjesson, A. Lennartson and K. Moth-Poulsen, Efficiency Limit of Molecular Solar Thermal Energy Collecting Devices, ACS Sustainable Chem. Eng., 2013, 1, 585–590 CrossRef
.
- P. Bharmoria, S. Ghasemi, F. Edhborg, R. Losantos, Z. Wang, A. Mårtensson, M. Morikawa, N. Kimizuka, Ü. İşci, F. Dumoulin, B. Albinsson and K. Moth-Poulsen, Far-red triplet sensitized Z-to-E photoswitching of azobenzene in bioplastics, Chem. Sci., 2022, 13, 11904–11911 RSC
.
- V. A. Bren, A. D. Dubonosov, V. I. Minkin and V. A. Chernoivanov, Norbornadiene–quadricyclane—an effective molecular system for the storage of solar energy, Russ. Chem. Rev., 1991, 60, 451 CrossRef
.
- A. Lennartson, A. Roffey and K. Moth-Poulsen, Designing photoswitches for molecular solar thermal energy storage, Tetrahedron Lett., 2015, 56, 1457–1465 CrossRef CAS
.
- T. J. B. Zähringer, J. A. Moghtader, M. Bertrams, B. Roy, M. Uji, N. Yanai and C. Kerzig, Blue-to-UVB Upconversion, Solvent Sensitization and Challenging Bond Activation Enabled by a Benzene-Based Annihilator, Angew. Chem., Int. Ed., 2022, e202215340 Search PubMed
.
- X. Jiang, X. Guo, J. Peng and D. Zhao, Triplet − Triplet Annihilation Photon Upconversion in Polymer Thin Film: Sensitizer Design, ACS Appl. Mater. Interfaces, 2016, 8, 11441–11449 CrossRef CAS PubMed
.
- Y. Y. Cheng, B. Fückel, R. W. MacQueen, T. Khoury, R. G. C. R. Clady, T. F. Schulze, N. J. Ekins-Daukes, M. J. Crossley, B. Stannowski, K. Lips and T. W. Schmidt, Improving the light-harvesting of amorphous silicon solar cells with photochemical upconversion, Energy Environ. Sci., 2012, 5, 6953–6959 RSC
.
- T. F. Schulze, Y. Y. Cheng, B. Fückel, R. W. MacQueen, A. Danos, N. J. L. K. Davis, M. J. Y. Tayebjee, T. Khoury, R. G. C. R. Clady, N. J. Ekins-Daukes, M. J. Crossley, B. Stannowski, K. Lips and T. W. Schmidt, Photochemical Upconversion Enhanced Solar Cells: Effect of a Back Reflector, Aust. J. Chem., 2012, 65, 480–485 CrossRef CAS
.
- T. F. Schulze, J. Czolk, Y. Y. Cheng, B. Fückel, R. W. MacQueen, T. Khoury, M. J. Crossley, B. Stannowski, K. Lips, U. Lemmer, A. Colsmann and T. W. Schmidt, Efficiency enhancement of organic and thin-film silicon solar cells with photochemical upconversion, J. Phys. Chem. C, 2012, 116, 22794–22801 CrossRef CAS
.
- T. F. Schulze, Y. Y. Cheng, T. H. Khoury, M. Crossley, B. Stannowski, K. Lips and T. W. Schmidt, Micro-optical design of photochemical upconverters for thin-film solar cells, J. Photonics Energy, 2013, 3, 34598 CrossRef CAS
.
- Y. Y. Cheng, A. Nattestad, T. F. Schulze, R. W. Macqueen, B. Fückel, K. Lips, G. G. Wallace, T. Khoury, M. J. Crossley and T. W. Schmidt, Increased upconversion performance for thin film solar cells: a trimolecular composition, Chem. Sci., 2016, 7, 559–568 RSC
.
- C. Simpson, T. M. Clarke, R. W. MacQueen, Y. Y. Cheng, A. J. Trevitt, A. J. Mozer, P. Wagner, T. W. Schmidt and A. Nattestad, An intermediate band dye-sensitised solar cell using triplet–triplet annihilation, Phys. Chem. Chem. Phys., 2015, 17, 24826–24830 RSC
.
- S. P. Hill, T. Banerjee, T. Dilbeck and K. Hanson, Photon Upconversion and Photocurrent Generation via Self-Assembly at Organic-Inorganic Interfaces, J. Phys. Chem. Lett., 2015, 6, 4510–4517 CrossRef CAS PubMed
.
- S. P. Hill, T. Dilbeck, E. Baduell and K. Hanson, Integrated Photon Upconversion Solar Cell via Molecular Self-Assembled Bilayers, ACS Energy Lett., 2016, 1, 3–8 CrossRef CAS
.
- T. Dilbeck, S. P. Hill and K. Hanson, Harnessing molecular photon upconversion at sub-solar irradiance using dual sensitized self-assembled trilayers, J. Mater. Chem. A, 2017, 5, 11652–11660 RSC
.
- D. Beery, A. Arcidiacono, J. P. Wheeler, J. Chen and K. Hanson, Harnessing near-infrared light via S0 to T1 sensitizer excitation in a molecular photon upconversion solar cell, J. Mater. Chem. C, 2022, 10, 4947–4954 RSC
.
- T. Morifuji, Y. Takekuma and M. Nagata, Integrated Photon Upconversion Dye-Sensitized Solar Cell by Co-adsorption with Derivative of Pt-Porphyrin and Anthracene on Mesoporous TiO2, ACS Omega, 2019, 4, 11271–11275 CrossRef CAS PubMed
.
- L. Frazer, J. K. Gallaher and T. W. Schmidt, Optimizing the Efficiency of Solar Photon Upconversion, ACS Energy Lett., 2017, 2, 1346–1354 CrossRef CAS
.
-
P. Bharmoria and S. P. M. Ventura, in Nanomaterials for Healthcare, Energy and Environment, ed. A. H. Bhat, I. Khan, M. Jawaid, F. O. Suliman, H. Al-Lawati and S. M. Al-Kindy, Springer Singapore, Singapore, 2019, pp. 1–29 Search PubMed
.
- D. Beery, J. P. Wheeler, A. Arcidiacono and K. Hanson, CdSe Quantum Dot Sensitized Molecular Photon Upconversion Solar Cells, ACS Appl. Energy Mater., 2020, 3, 29–37 CrossRef CAS
.
- Y. Zhou, C. Ruchlin, A. J. Robb and K. Hanson, Singlet Sensitization-Enhanced Upconversion Solar Cells via Self-Assembled Trilayers, ACS Energy Lett., 2019, 4, 1458–1463 CrossRef CAS
.
- C. Li, C. Koenigsmann, F. Deng, A. Hagstrom, C. A. Schmuttenmaer and J. H. Kim, Photocurrent Enhancement from Solid-State Triplet–Triplet Annihilation Upconversion of Low-Intensity, Low-Energy Photons, ACS Photonics, 2016, 3, 784–790 CrossRef CAS
.
- J. Y. Kim, J. W. Lee, H. S. Jung, H. Shin and N. G. Park, High-Efficiency Perovskite Solar Cells, Chem. Rev., 2020, 120, 7867–7918 CrossRef CAS PubMed
.
- V. Sarritzu, N. Sestu, D. Marongiu, X. Chang, Q. Wang, S. Masi, S. Colella, A. Rizzo, A. Gocalinska, E. Pelucchi, M. L. Mercuri, F. Quochi, M. Saba, A. Mura and G. Bongiovanni, Direct or Indirect Bandgap in Hybrid Lead Halide Perovskites?, Adv. Opt. Mater., 2018, 6, 1–8 Search PubMed
.
- National Renewable Energy Laboratory (NREL), Best Research-Cell Efficiencies.
- E. M. Gholizadeh, S. K. K. Prasad, Z. L. Teh, T. Ishwara, S. Norman, A. J. Petty, J. H. Cole, S. Cheong, R. D. Tilley, J. E. Anthony, S. Huang and T. W. Schmidt, Photochemical upconversion of near-infrared light from below the silicon bandgap, Nat. Photonics, 2020, 14, 585–590 CrossRef CAS
.
- W. Sheng, J. Yang, X. Li, G. Liu, Z. Lin, J. Long, S. Xiao, L. Tan and Y. Chen, Tremendously enhanced photocurrent enabled by triplet–triplet annihilation up-conversion for high-performance perovskite solar cells, Energy Environ. Sci., 2021, 14, 3532–3541 RSC
.
- W. Sheng, J. Yang, X. Li, J. Zhang, Y. Su, Y. Zhong, Y. Zhang, L. Gong, L. Tan and Y. Chen, Dual Triplet Sensitization Strategy for Efficient and Stable Triplet–Triplet Annihilation Upconversion Perovskite Solar Cells, CCS Chem., 2022, 3, 1–12 Search PubMed
.
- Z. Wang, H. Hölzel and K. Moth-Poulsen, Status and challenges for molecular solar thermal energy storage system based devices, Chem. Soc. Rev., 2022, 51, 7313–7326 RSC
.
- F. Kasten and A. T. Young, Revised optical air mass tables and approximation formula, Appl. Opt., 1989, 28, 4735–4738 CrossRef CAS PubMed
.
- K. Börjesson, D. Dzebo, B. Albinsson and K. Moth-Poulsen, Photon upconversion facilitated molecular solar energy storage, J. Mater. Chem. A, 2013, 1, 8521–8524 RSC
.
- A. Vasilev, R. Dimitrova, M. Kandinska, K. Landfester and S. Baluschev, Accumulation of the photonic energy of the deep-red part of the terrestrial sun irradiation by rare-earth metal-free E–Z photoisomerization, J. Mater. Chem. C, 2021, 9, 7119–7126 RSC
.
- M. S. Chowdhury, K. S. Rahman, T. Chowdhury, N. Nuthammachot, K. Techato, M. Akhtaruzzaman, S. K. Tiong, K. Sopian and N. Amin, An overview of solar photovoltaic panels’ end-of-life material recycling, Energy Strateg. Rev., 2020, 27, 100431 CrossRef
.
- X. Wang, X. Tian, X. Chen, L. Ren and C. Geng, A review of end-of-life crystalline silicon solar photovoltaic panel recycling technology, Sol. Energy Mater. Sol. Cells, 2022, 248, 111976 CrossRef CAS
.
- Y. Xu, J. Li, Q. Tan, A. L. Peters and C. Yang, Global status of recycling waste solar panels: a review, Waste Manage., 2018, 75, 450–458 CrossRef PubMed
.
- M. Tao, V. Fthenakis, B. Ebin, B.-M. Steenari, E. Butler, P. Sinha, R. Corkish, K. Wambach and E. S. Simon, Major challenges and opportunities in silicon solar module recycling, Prog. Photovoltaics Res. Appl., 2020, 28, 1077–1088 CrossRef CAS
.
- R. F. Service, Science, 2011, 332, 293 CrossRef CAS PubMed
.
- J. K. Rath, M. Brinza, Y. Liu, A. Borreman and R. E. I. Schropp, Fabrication of thin film silicon solar cells on plastic substrate by very high frequency PECVD, Sol. Energy Mater. Sol. Cells, 2010, 94, 1534–1541 CrossRef CAS
.
- A. Petritz, A. Wolfberger, A. Fian, M. Irimia-Vladu, A. Haase, H. Gold, T. Rothländer, T. Griesser and B. Stadlober, Cellulose as biodegradable high-k dielectric layer in organic complementary inverters, Appl. Phys. Lett., 2013, 103, 153303 CrossRef
.
- I. Nainggolan, T. I. Nasution, S. R. E. Putri, D. Azdena, M. Balyan and H. Agusnar, Study on chitosan film properties as a green dielectric, IOP Conf.: Ser. Mater. Sci. Eng., 2018, 309, 12081 Search PubMed
.
- C. G. A. Lima, R. S. de Oliveira, S. D. Figueiró, C. F. Wehmann, J. C. Góes and A. S. B. Sombra, DC conductivity and dielectric permittivity of collagen–chitosan films, Mater. Chem. Phys., 2006, 99, 284–288 CrossRef CAS
.
- L. Gao, L. Chao, M. Hou, J. Liang, Y. Chen, H.-D. Yu and W. Huang, Flexible, transparent nanocellulose paper-based perovskite solar cells, npj Flex. Electron., 2019, 3, 4 CrossRef
.
- Z. Wang, H. Moïse, M. Cacciarini, M. B. Nielsen, M. Morikawa, N. Kimizuka and K. Moth-Poulsen, Liquid-Based Multijunction Molecular Solar Thermal Energy Collection Device, Adv. Sci., 2021, 8, 2103060 CrossRef CAS PubMed
.
- S. Izawa and M. Hiramoto, Efficient solid-state photon upconversion enabled by triplet formation at an organic semiconductor interface, Nat. Photonics, 2021, 15, 895–900 CrossRef CAS
.
- L. Wei, C. Fan, M. Rao, F. Gao, C. He, Y. Sun, S. Zhu, Q. He, C. Yang and W. Wu, Triplet–triplet annihilation upconversion in LAPONITE®/PVP nanocomposites: absolute quantum yields of up to 23.8% in the solid state and application to anti-counterfeiting, Mater. Horiz., 2022, 9, 3048–3056 RSC
.
|
This journal is © the Partner Organisations 2023 |
Click here to see how this site uses Cookies. View our privacy policy here.