DOI:
10.1039/D3QM00121K
(Review Article)
Mater. Chem. Front., 2023,
7, 2337-2358
Recent advances in electrochromic materials and devices for camouflage applications
Received
2nd February 2023
, Accepted 6th March 2023
First published on 7th March 2023
Abstract
Electrochromic (EC) materials and devices, whose optical properties (color, reflectance, emittance, etc.) can be manipulated by switching to a low-voltage bias, show great promise in many applications such as smart windows, adaptive military camouflage, displays, and anti-glare rearview mirrors. In recent years, EC materials and devices for camouflage, including color camouflage and thermal camouflage, have attracted growing attention and research interest due to their many attractive properties such as rapid response, controllable optical modulation, low energy consumption (bistability), and flexibility. Despite the continuous development of related fields, the design and fabrication of EC devices that truly meet the requirements of camouflage applications still face tremendous challenges. Herein, in this review, we first briefly introduce the structures and main performance parameters of EC devices for camouflage. Then we comprehensively summarize the latest research progress of EC technology in the camouflage field from the aspects of color camouflage and thermal camouflage. Finally, the current challenges and future perspectives in the EC camouflage field are proposed and discussed. It is our hope that this review will accelerate the development of these promising EC materials and devices and provide guidance for developing mature camouflage devices.
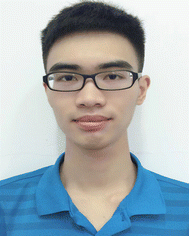
Haichang Fu
| Haichang Fu received his Bachelor's Degree in Chemical Engineering and Technology from Hunan Institute of Science and Technology in 2018. He is currently a PhD candidate in Chemical Engineering and Technology at the Institute of Chemical Engineering and Technology at Zhejiang University of Technology. His current research focuses on the synthesis of novel soluble electrochromic conjugated polymers and their application in camouflage. |
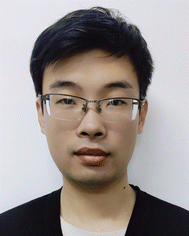
Ling Zhang
| Ling Zhang received his PhD degree in Chemical Engineering and Technology from the Institute of Chemical Engineering and Technology at Zhejiang University of Technology in 2021. After graduation, he continued to work at the Zhejiang University of Technology undergoing postdoctoral research. His research interests focus on the design, synthesis, and application of conjugated polymer-based electrochromic materials. |
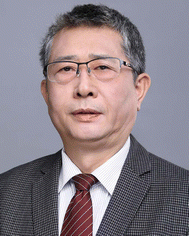
Cheng Zhang
| Prof. Cheng Zhang received his PhD degree in polymer materials at Zhejiang University in 1998. In the following year, he carried out research work at Tokyo Institute of Technology as a JSPS post-doctoral fellow. In 2002, he joined the College of Chemical Engineering, Zhejiang University of Technology as a professor. His research interests mainly focus on the design and synthesis of novel conjugated polymer materials, as well as their fundamental and application research in the field of organic optoelectronics and energy storage. |
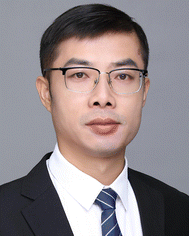
Weijun Li
| Dr Weijun Li received his PhD degree in Polymer Chemistry and Physics at Jilin University in 2013 under the supervision of Professor Yuguang Ma. Then he underwent one year of postdoc research work at the University of Hong Kong. In 2015, he joined the College of Chemical Engineering, Zhejiang University of Technology as an associate professor. His research focuses on the synthesis and photophysical properties of organic photoelectronic materials, as well as their applications in the field of organic electrochromism, electroluminescence, and energy storage. |
1. Introduction
With the rapid development of science and technology, camouflage technologies are developing toward lighter, more comfortable and more intelligent properties. In terms of adaptive chromic camouflage technology, there are three types of chromic technologies, including thermochromism,1–3 photochromism,4,5 and electrochromism,6–8 which control the optical properties of materials and devices in response to an external stimulus such as heat, light, or electricity. Among them, the application of thermochromic and photochromic materials and devices in adaptive camouflage is limited, because their optical properties strongly depend on the temperature and light intensity, and are often difficult to control and operate precisely.9 Moreover, the response speed and stability of photochromic materials and devices require further investigation and improvement. Among various camouflage technologies, electrochromic (EC) camouflage has received extensive attention and research interest due to its unique advantages and potential values. Some EC camouflage materials and devices with excellent properties have gradually emerged and been reported in detail.10–12 In recent years, EC camouflage technologies have made significant progress in both practical and theoretical terms, and are expected to be one of the next-generation camouflage technologies that people have long been awaiting.
The working principle of EC camouflage is based on the electrochemically driven redox process of EC materials to produce color/reflectivity/emissivity changes so that the objects exhibit adaptive changes to the surrounding environment and achieve perfect concealment from visual and infrared detection. Based on the differences in physical mechanisms, EC camouflage technology can be divided into two categories: color camouflage and thermal camouflage (Fig. 1). Compared with other camouflage materials and devices, EC materials and devices demonstrate many unique advantages in camouflage applications. First, most existing EC materials show certain flexibility and processability while maintaining good compatibility with multiple substrates, including metal, plastic, and fibers, which meet wearable fabric requirements.14–16 Second, EC devices have good optical tunability, which can be tuned by matching the optical properties of each functional layer of the device to meet the needs of camouflage in different surroundings.17,18 Finally, the unique optical memory effect and fast response of EC devices are also highly favorable for camouflage applications.19–22 Therefore, EC materials and devices have great application potential in color camouflage and thermal camouflage. Compared to other EC applications (smart windows, EC displays, antiglare rearview mirrors, etc.), ideal EC camouflage devices have higher performance requirements, such as smaller chromatic aberration
with the surrounding environment to ensure concealment of camouflage, better flexibility to ensure comfort and practicality, and better stability and durability to support their life. Despite the remarkable progress that EC camouflage technology has achieved through the design of EC materials and devices, there are some critical issues that need to be addressed to boost their future research and applications, for instance, the design and development of EC camouflage devices which can independently control visible light (VIS) reflectivity and infrared (IR) emissivity, and the fabrication and research of high-performance wearable fabric EC camouflage devices. The current research and development process of EC camouflage devices is still in the early stage. And the practical application of relevant products still has a long way to go.
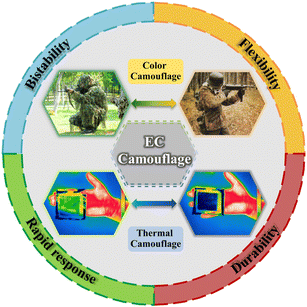 |
| Fig. 1 An illustration of EC camouflage. Reproduced from ref. 13. Copyright 2018, American Chemical Society. | |
The study of EC camouflage materials and devices is of great significance to further expand the EC application fields. However, there is little systematic and overall design guidance. Thus, comprehensive summary and analysis of the recent advances in EC camouflage are necessary to provide directions for developing high-performance EC camouflage devices to push their real-world applications. This review will systematically summarize and discuss in detail the latest development of EC camouflage materials and devices to comprehensively understand their current research level and existing challenges for further development. First, we present the EC device structures and main performance parameters for camouflage applications. Then, the latest research progress of EC technology in color camouflage and thermal camouflage is summarized in detail. The advantages and limitations of each material and device or design strategy are analyzed and compared to guide the fabrication of high-performance EC camouflage devices. Finally, the challenges and perspectives for future research on EC camouflage materials and devices are proposed and discussed. It is our hope that this review will provide guidance for developing high-performance mature EC camouflage devices.
2. Electrochromism
Electrochromism refers to the reversible change of the optical properties (such as colors and transmittance) of materials under charge insertion/extraction or chemical reduction/oxidation induced by the application of an external voltage or current.23–25 The term “electrochromism” was first coined by Platt in 1961 when he discovered that amorphous WO3 exhibited different colors under different external voltage conditions.26 Since then, electrochromism has been attracting the interest of researchers.27–30 In 1984, the concept of energy-saving windows (smart windows) was proposed to make electrochromism known to people.31,32 During its development over 50 years, EC technology has been used in a wide range of applications, including smart windows,33–36 displays,37–39 and sunglasses,40–42 due to the tremendous efforts of outstanding pioneers.
2.1 Structure of EC devices for camouflage
For the application of EC technology, electrochromic devices (ECDs) as basic working modules need to be studied and manufactured. For different applications, ECDs can be divided into two categories: transmissive window-type devices (for smart windows and EC sunglasses, etc.) and reflective-type devices (for EC displays, adaptive camouflage, and EC antiglare mirrors, etc.).43 It is worth noting that the EC camouflage devices discussed in this review are reflective-type devices. In the traditional design, ECDs are usually in a seven superimposed thin layers structure consisting of two substrates, two conducting electrodes, an EC layer, an electrolyte layer, and an ion storage layer, as shown in the schematic illustration (Fig. 2a). To meet future and sophisticated application needs, ECDs with a lateral structure in which the EC layer and the ion storage layer are arranged in a shoulder-by-shoulder manner have been proposed and manufactured (Fig. 2b).44 In camouflage ECDs, the EC layer plays a core role; it undertakes the task of optical modulation or color change. In this regard, we will systematically discuss the EC layer in Section 3.1 and Section 4. The ion storage layer functions as a counter electrode layer, undergoing reversible electrochemical oxidation (reduction) to coordinate the reduction (oxidation) of the EC material to balance the charge shuttling from the EC layer through the electrolyte.45–47 The electrolyte layer sits in the center of the device, which can separate the EC layer and ion storage layer to prevent direct electrical contact and transport ions inside the device.48 Substrates and conducting layers as conductive electrodes which determine the mechanical properties of the devices are commonly discussed together.49
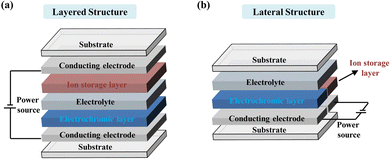 |
| Fig. 2 Structures of the classic (a) layered and (b) lateral ECD. | |
2.2 Performance parameters for camouflage
In order to promote the development of EC materials and devices, researchers have used a series of performance indexes to evaluate them, generally including optical and non-optical analyses. The optical analysis focuses on measuring changes in color and transmittance through techniques related to optical spectroscopy, including optical modulation, contrast ratio, optical memory, and chromatic aberration (
). Non-optical analyses include electrochemical and other conventional analyses for evaluating the electrochemical/kinetics properties of film and devices, including switching time, long-term stability and durability, and coloration efficiency (CE). Of course, for different applications, researchers are concerned with different performance parameters and requirements. For instance, EC materials and devices used for electronic displays must exhibit ultra-fast response rates (<40 ms); in contrast, when used for smart windows, the requirement for response time is not too high, but the requirements for optical modulation capabilities and memory effects are outstanding. As the field grows, it is essential to give precise definitions of individual performance metrics and to provide a standardized performance measurement for different EC applications. Here, we focus on the camouflage field. The camouflage performance of the EC materials and devices is usually evaluated by the main performance parameters of optical modulation, switching rates, cycling stability and weatherability, coloration efficiency, optical memory, and color difference. High-performance EC camouflage devices should exhibit excellent modulation capabilities, lasting cycling stability, and outstanding optical memory while maintaining a small
with the surrounding environment to ensure its camouflage.
3. EC materials and devices for color camouflage
Adaptive color camouflage requires the material to adjust its color to the color of the surroundings to blend with it and achieve perfect concealment of the target.50,51 In order to survive and reproduce, many natural creatures, such as oceanic cephalopods and chameleons, are born with the ability to change their skin color in this way to blend in with the natural environment for camouflage. Currently, EC technologies have significant application potential for adaptive color camouflage due to their rich color and ease of control.
The critical point in EC color camouflage is to design and prepare high-performance EC materials and devices with camouflage color switching. Firstly, it is clear that the primary requirement for EC materials and devices for military color camouflage is the ability to switch between camouflage colors. Camouflage colors usually refer to yellow and green shades, such as sandy yellow and dark green. Secondly, to achieve an efficient camouflage effect, the excellent EC properties of the materials and devices are also required, such as fast switching time and robust stability. In this section, the efforts made by researchers to realize camouflage applications in terms of color modulation as well as performance optimization are summarized from both material and device design.
3.1 EC materials for color camouflage
To date, EC materials can be divided into inorganic and organic EC materials according to the difference in EC mechanisms. For inorganic materials, single color variation is their greatest limitation in applications,52 so is adaptive color camouflage. For example, WO3, the most widely used inorganic EC material, exhibits only a monochromic color change from transparent to dark blue.53 This problem can be effectively solved by the introduction of structural colors and the preparation of composite materials.54,55 The great advantage of organic EC materials compared to inorganic materials is that the color can be tuned by means of molecular structural design.56–58 Of course, structural colors and color composites are also common means of color modulation in organic materials.59,60 Next, we discuss in detail the strategies that can be used to prepare EC materials for camouflage color conversions.
3.1.1 Introduced structural colors.
Structural colors, well known from coloration in nature, refer to colors emerging from the interaction (refraction, diffuse reflection, or diffraction) of light with micro- and nano-structures with periodicities on the order of the wavelength,61–63 including Fano and Fabry–Perot (F–P) resonances,64,65 and localized surface plasmon resonances (LSPR)66–68 and so on. Different from dyes and pigments, structural colors require only very thin layers, or more precisely tiny volumes, to create brighter colors under sunlight that do not fade over time.69 Combining electrically-driven chemical color changes with structural color changes (also known as physical color) is a viable strategy for realizing color modulation in EC materials and has received extensive attention.
In 2012, Geoffrey A. Ozin et al.70 reported an electrochromic Bragg mirror (ECBM) based on multilayer photonic crystal structures of WO3 and NiO with different refractive indices and achieved a reversible color change from dark green to yellow. The change in the refractive index of the film at different applied voltages is responsible for the color tuning of ECBM. To enhance the reflectance modulation of the ECBM, Xingyuan Liu and Ying Lv et al.71 fabricated electroresponsive WO3-based electrochromic distributed Bragg reflectors (ECDBRs) by alternating the deposition of WO3 layers with various porosities (refractive indices) (Fig. 3a). By simply changing the deposition angle and thickness of each WO3 layer, the Bragg wavelength of ECDBRs could be adjusted in the VIS range continuously. Among them, ECDBR-550 is capable of reversible switching between yellow-green and green at the applied voltage, as shown in Fig. 3a.
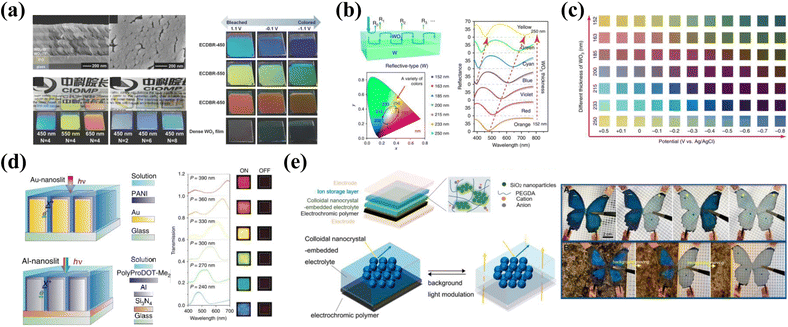 |
| Fig. 3 (a) Left: cross-section, surface SEM images and photographs of the ECDBR. Right: EC properties of ECDBRs. Reproduced from ref. 71. Copyright 2017, Wiley-VCH GmbH. (b) Left: schematic and calculated color coordinates of an EC electrode using a metallic W layer as a current collector. Right: simulated (dashed lines) and measured (solid lines) reflection spectra with different thickness of WO3. (c) Color gallery obtained from an F–P nanocavity-type EC electrode at different applied potentials. Reproduced from ref. 65. Copyright 2020 (CC BY 4.0), Springer Nature. (d) Left: schematic of a plasmonic EC electrode with Au (top) or Al (bottom) nanoslit arrays. Right: optical transmission spectra of PolyProDOT-Me2-coated Al-nanoslit structures with different slit periods (P). Reproduced from ref. 73. Copyright 2016 (CC BY 4.0), Springer Nature. (e) Left: schematic of the photonic nanocrystal embedded EC. Right: the color appearance/disappearance of the butterfly device for both active and passive camouflage. Reproduced from ref. 75. Copyright 2021, American Chemical Society. | |
Color tuning in EC materials can also be achieved by constructing an F–P resonances structure.64 Zhigang Zhao et al.65 fabricated an ultra-compact asymmetric F–P nanocavity-type EC film by introducing metallic tungsten (W) between the WO3 layers and Indium Tin Oxide (ITO) electrodes to induce strong interference resonance (Fig. 3b). By adjusting the thickness of the WO3 layer, the F–P nanocavity-type EC electrode can display various structure colors. In addition, the EC electrode could also achieve multicolor displays by applying different voltages (Fig. 3c). In particular, when the thickness of WO3 is 163 nm, the electrode could achieve reversible switching in yellow (−0.4 V) and green (−0.8 V) colors. This research result is an important development in the multicolor display of inorganic EC materials.
Furthermore, as the most frequent Plasmonic resonance structures, structural colors can also be introduced into organic EC materials for the purpose of color modulation.72 In 2016, A. Alec Talin et al.73 fabricated a fast speed switching and multicolor EC electrode based on plasmonic nanoslit arrays (Fig. 3d). The required nanoslit arrays were obtained by focused ion beam (FIB) milling. Then, polyaniline (PANI) and poly(2,2-dimethyl-3,4 propylenedioxythiophene) (PolyProDOT-Me2) (as EC material) were fabricated on nanoslit arrays by potentio-dynamic cycling to a thickness of 15 nm. The peak spectral transmission of the PolyProDOT-Me2 electrodes could be adjusted by altering the period of the array to achieve a full-color EC optical response, including yellow and green (Fig. 3d). And then, the ultra-thin film brought about by the introduction of structural color resulted in a fast response time (80 ms). A similar method of introducing structural color into PANI film was reported by Hui-Ying Qu and co-workers.74 The authors have prepared a lamellar PANI/PS-b-P2VP thin film to enable the simulation of chromatophores and iridophores color changes using the combined EC effect of PANI and structural coloration of PS-b-P2VP.
In addition to constructing special structures in EC layers or electrodes, color tuning can also be achieved by introducing structural colors into the electrolyte. Jianguo Mei et al.75 reported an electrolyte with a special structure by embedding silica nanoparticles into an ethylene glycol (EG)/poly(ethylene glycol) methacrylate (PEGMA) matrix. The voltage-controlled EC polymer enables the device to actively switch from a colored state to a high transmission state. On the other hand, the devices show background adaptive visual effects in a passive manner when the EC polymer is kept in a highly transmissive state. Finally, a butterfly ECD capable of both active and passive camouflage was prepared to demonstrate its application potential (Fig. 3e).
3.1.2 Modified molecular structure.
Over the past few years, great achievements have been made in modulating the color of organic EC materials by modifying the molecular structure,76–78 which is also considered to be one of the most important strategies to achieve color tuning of EC materials. Using this strategy, a lot of green and yellow EC materials for adaptive color camouflage have been designed and synthesized, such as small organic molecules,79–82 metal–organic complexes,83,84 and conjugated polymers.85–87
Small organic molecules mainly include viologen and its derivatives,88,89 organic redox dyes,16,90etc. Among various small molecule EC materials, the cathodic EC material 1,1′-disubstituted-4,4′-bipyridinium salts, commonly called viologens, is by far the most intensively investigated.91,92 The EC color properties of viologens could be readily tuned by altering the N-substituent group and counter anions.88 C. Daniel Frisbie et al.79 synthesized a viologen derivative (CPQ) with 4-cyanophenyl as the N-substituent group, which could reversibly switch from yellow to green (Fig. 4a). Later, Hong Chul Moon et al.80 synthesized a viologen derivative (TFMFPhV(TFSI)2) using 3-fluoro-4-(trifluoromethyl)phenyl as the N-substituent, which also exhibited a yellow-to-green color electrochromism. In comparison to CPQ, the TFMFPhV(TFSI)2 exhibited an improved EC performance with better coloration/bleaching operational stability. And there are other strategies to achieve color modulation of viologen. Gang He's group proposed the extending p-conjugation method to stabilize the radical state and tune the EC properties of viologens.81 Based on this, a p-extended chalcogenoviologens (Se-PhV2+) with stable radical states and reversible color changes between yellow and dark green was successfully obtained (Fig. 4b).
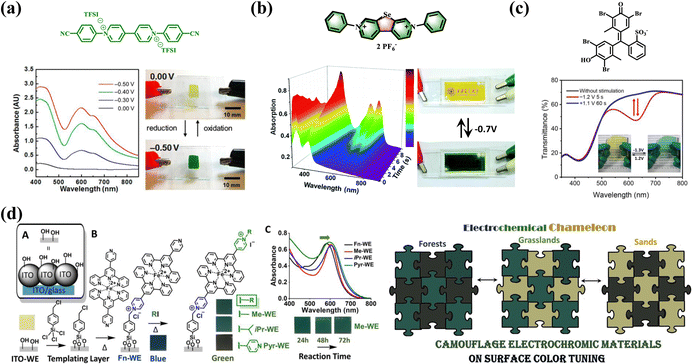 |
| Fig. 4 (a) Molecular structure, spectra and photographs of viologen derivative CPQ in the bleached and colored states. Reproduced from ref. 79. Copyright 2016, American Chemical Society. (b) Molecular structure, UV-vis spectra, and photographs of p-extended chalcogenoviologens (Se-PhV2+). Reproduced from ref. 81. Copyright 2020, Royal Society of Chemistry. (c) Molecular structure, UV-vis spectra, and photographs of bromocresol green derivatives at −1.2 V and +1.5 V. Reproduced from ref. 82. Copyright 2021, Chinese Chemical Society. (d) Left: scheme demonstrating steps for the preparation of WEs. Right: schematic of adaptive military camouflage. Reproduced from ref. 83. Copyright 2021, American Chemical Society. | |
Recently, Sean Xiao-An Zhang and Yu-Mo Zhang et al.82 reported a bromocresol green derivative that can also switch between yellow and green with different electrical stimulation (Fig. 4c). Unlike the EC mechanism of other small molecule materials, the EC mechanism of bromocresol green derivative is based on proton-coupled electron transfer, which is the reversible proton release/capture of molecules during the electrochemical oxidation/reduction process under voltage stimulation.90
In addition to the above-mentioned relevant studies on small organic molecules, Olena V. Zenkina et al.83 reported an impressive EC camouflage material based on metal–organic complexes. Due to metal-to-ligand charge transfer (MLCT), the EC properties of metal complexes were found to be greatly dependent on the electrochemical oxidation of the metal center, and its actual color is determined by the nature of the metal center and the structure of the ligand.93 The authors synthesized a series of green-color EC materials by reacting a templating layer with pyridyl nitrogen atoms (Fig. 4d). The color of the EC foundation monolayer could be finely tuned by altering the N-quaternization ammonium group. The tests showed that these materials have different shades of green and become yellowish or clay under applied voltage. The accessible color gamut meets the requirements of military camouflage and can simulate various natural environments, including forests and sands (Fig. 4d).
Despite the late start of the research, electrochromic conjugated polymer (ECP) materials have also received remarkable attention and shown attractive potential for adaptive military camouflage.10 Color tuning can be efficiently achieved by controlling the band gap of the ECP materials in neutral and doped states, which is the greatest advantage of ECP materials. Through the efforts of many related research and development projects, researchers have used structural design strategies to prepare many high-performance ECP materials that can be applied in military camouflage. Polyaniline (PANI), a classical ECP material, is a very desirable material for EC military camouflage due to the color switching ability between green and yellow.94,95 Xiaolong Weng et al.96 prepared a coral-like PANI film with nanowire structures by the combination of galvanostatic and cyclic voltammetric electrodeposition techniques. The PANI film showed reversible color changes between brownish-yellow, green, and dark green, which is essential for adaptive camouflage in natural conditions (Fig. 5a). In addition, there are many factors that affect the EC properties of the PANI, such as acid dopants, thickness, and morphology of film, substrate, etc. Among them, acid dopants are a large and widely studied influence.97 Commonly used acidic dopants are divided into inorganic acids such as HClO4 and H2SO4 and organic acids such as dodecylbenzene sulfonate acid (DBSA) and camphorsulfonic acid (CSA).
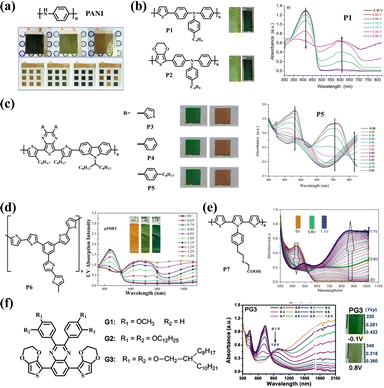 |
| Fig. 5 (a) Molecular structure and photographs of PANI at different applied potentials. Reproduced from ref. 96. Copyright 2022, Elsevier Ltd. (b) Structure, photographs and spectra of polymers P1 and P2. Reproduced from ref. 85. Copyright 2006, American Chemical Society. (c) Structure and photographs and spectra of polymers P3–P5. Reproduced from ref. 86. Copyright 2009, American Chemical Society. (d) Structure, photographs and spectra of thiophene derivative P6. Reproduced from ref. 87. Copyright 2018, Royal Society of Chemistry. (e) Structure, photographs and spectra of polymers P7. Reproduced from ref. 100. Copyright 2018, Elsevier B.V. (f) Structure, photographs and spectra of EDOT-based polymer. Reproduced from ref. 10 (CC BY 3.0). Copyright 2016, Royal Society of Chemistry. | |
Based on triphenylamine and carbazole structures similar to aniline, many ECP camouflage materials have also been synthesized and reported.85,86 As early as 2006, two ECPs, P1 and P2, were synthesized by Mario Leclerc et al.85 by using the polymerization reaction between the 4-butyltriphenylamine unit and thiophene or 3,4-ethylenedioxythiophene (EDOT) (Fig. 5b). These two ECPs show good optical and EC properties for military camouflage with a color transition from pale yellow to green. Subsequently, three new poly(2,7-carbazole) derivatives (P3–P5) (Fig. 5c) were also reported,86 which reflect the green light in their neutral state and show the transmissive brown color after being oxidized. In addition, poly(2,7-carbazole) derivatives (P3–P5) show excellent solubility in common organic solvents such as chloroform, toluene, and tetrahydrofuran, which is important for their further commercial applications via solution processing.
Electrochemical polymerization is a well-established methodology to prepare polymer film directly on an electrode surface without unmanageable chemical polymerization reactions and complicated purifications.98,99 Cheng Zhang et al.87 prepared a star-shaped thiophene derivative P6 consisting of one central core of phenyl and three arms of bithiophene by electrochemical polymerization (Fig. 5d). The P6 film can switch reversibly between orange-yellow, green and blue under applied voltage. Furthermore, the film demonstrated an outstanding EC switching speed, with the coloring and bleaching times of 1.5 s and 4.2 s between orange-yellow and green, respectively. These results show that P6 polymers have the potential to be used as a yellow-to-green EC material with rapid switching time for adaptive military camouflage. Similarly, Fatma Baycan Koyuncu et al.100 synthesized an EC polymer based on the pyrrole–thiophene structure that can also switch reversibly between brown, green, and blue (Fig. 5e). Hong Meng, Wei Huang, and coauthors10 synthesized a donor–acceptor-type EDOT-based polymer that reversibly changes between green color and sand-tone colors by adjusting the alkyl chain on the side chain of the quinoline group (Fig. 5f).
In addition, metallic supramolecular polymers (MSPs) have been extensively studied as an emerging EC conductive polymer material.101–104 Metal-to-ligand charge transfer (MLCT) plays a key role in the EC behavior of MSPs.105 Furthermore, the structure of the ligand also has significant influence on the EC dynamics and optical properties of MSPs.106 Masayoshi Higuchi et al. have intensively investigated MSPs containing different central metal ions such as Fe(II), Ru(II), and Co(II), etc.107–109 They synthesized dual-redox metallo-supramolecular polymers with zigzag structures by using Fe(II) as the redox-active ion and 4,4-bis(2,2:6,2-terpyridinyl)phenyl-triphenylamine as the redox-active ligand.110 Under applied voltage, the MSPs film shows a reversible color change from brown to light green, and exhibits high optical contrast and excellent cyclic stability. This kind of novel EC material has great application potential in color camouflage through the reasonable design of central metal ions and ligand structure.
Finally, the modifiability of organic EC materials allows for the design and synthesis of new materials with a variety of desired functions through the introduction of some functional groups.111,112 For instance, Chunyang Jia et al.113 reported a flexible and stretchable EC copolymer film based on triphenylamine and self-healing Diels–Alder groups with excellent self-healing properties. This EC flexible film with self-healing properties also has great potential for application in military camouflage.
3.1.3 Prepared composite materials.
Considering the color switching abilities of different materials, the desired color switch can be achieved by mixing EC materials of complementary colors to form composites. According to the type of EC material, composites can be classified as inorganic/inorganic, inorganic/organic, and organic/organic types. Researchers have fabricated high-performance EC materials for adaptive military camouflage by manufacturing composite film. We will discuss each of these strategies systematically in this section to facilitate understanding.
The composite of two or more inorganic materials is an effective strategy for adjusting the color of the material.55,114 Shu-Hong Yu et al.115 reported multicolor inorganic EC composite film by coassembling W18O49 and V2O5 nanowires. By applying different voltages, the W18O49/V2O5 nanowires film shows dynamic colors of orange, green, and gray (Fig. 6a). Importantly, both the transmittance and color of the film could be easily controlled by manipulating the layers of coassembled nanowires and the ratios between the two nanowires. Subsequently, Rufan Zhang and coauthors improved the multicolor EC properties of vanadium oxide and the diversity of its camouflage environment by reconstructing a vanadium oxide containing various valence states of the V element.12 The prepared composite film shows outstanding multicolor EC performance with five reversible color transformations (orange, yellow, green, gray, and blue) at voltages of less than 1.5 V (Fig. 6b). The Au@VxO2x+1 nanoflowers could be adapted as a functional coating material to camouflage warships in the ocean and tanks in different natural scenarios, which shows an excellent camouflage function (Fig. 6b).
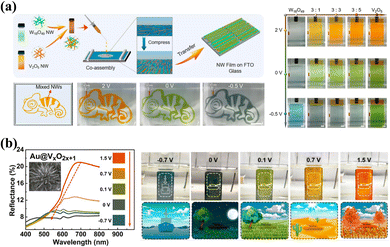 |
| Fig. 6 (a) Left: schematic and photographs for coassembling W18O49 and V2O5 NWs. Right: optical images of pure W18O49 NWs, coassemblies, and pure V2O5 NWs under different applied voltages. Reproduced from ref. 115. Copyright 2021, American Chemical Society. (b) Left: optical reflection spectra and corresponding optical images of Au@VxO2x+1 NFs films at different applied voltages. Right: application scenarios of the Au@VxO2x+1 NFs in adaptive camouflage fields. Reproduced from ref. 12. Copyright 2022, American Chemical Society. | |
In addition to color adjustment, the compound is also an effective strategy for improving EC performance. In 2018, Yucheng Wu et al.116 prepared WO3 nanorod/V2O5 dot hybrid arrays (Fig. 7a). Under the different applied voltages, the composite film could reversibly switch between black, green, and orange-yellow based on the color overlay (Fig. 7a). Meanwhile, this well-designed structure provides ion transport channels and significantly increases the active sites for electron and ion transfer. Thus, the nanorod arrays exhibit significantly enhanced EC properties with superior cyclic stability compared to the individual components. Compounding EC materials with transparent conducting nanomaterials provides a large electrochemically active surface area and an abundance of gaps, thus resulting in fast response times and excellent stability.115 Jiang-ping Tu and coauthors117 prepared a SnO2/V2O5 core/shell composite film with a rough surface by combining a hydrothermal method and electrodeposition (Fig. 7b). By alternating the strength of the applied voltages, the composite film could reversibly transform between yellow, green, and blue, which allows the material to be used as an adaptive camouflage material in all conditions of sand, forest and sea for military applications. Meanwhile, the composite film demonstrated superior EC performance to the single V2O5 film in optical modulation and cyclic stability (Fig. 7b).
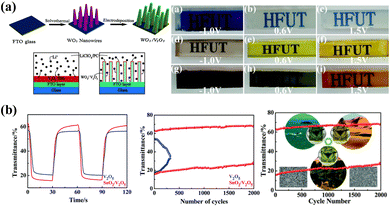 |
| Fig. 7 (a) Top left: schematic of the formation process of WO3/V2O5 hybrid films. Bottom left: a model of insertion/extraction of Li+ ions in different structures. Right: digital photographs of films at different applied voltages. Reproduced from ref. 116. Copyright 2018, Royal Society of Chemistry. (b) Left: transmittance curves and cyclic stability of V2O5 and SnO2/V2O5 films. Right: schematic of adaptive military camouflage. Reproduced from ref. 117. Copyright 2019, Royal Society of Chemistry. | |
Another viable strategy is to combine inorganic with organic to create inorganic/organic hybrid electrodes to realize multicolor camouflage of composite films. Guofa Cai et al.118 fabricated a WO3/PANI core/shell dual EC nanowire array film (Fig. 8a). Composite electrodes containing the cathodic coloration material WO3 and the anodic coloration material PANI could lead to a dual-electrochromism effect by separating the electroactivity within the application potential window, enabling a reversible color change between green, yellow, and dark blue (Fig. 8b). Recently, to improve the color accessibility of V2O5 film, a high-performance V2O5-methyl cellulose (VMC) composite film was fabricated by Quanyao Zhu, Jinyong Zhang, and coauthors (Fig. 8c).119 The as-prepared VMC composite film shows various colors (yellow, green, blue, chartreuse, emerald, medium, and dark green) at different applied voltages. Based on this, the as-prepared inorganic/organic hybrid film is able to simulate natural environments, including grassland and desert (Fig. 8d).
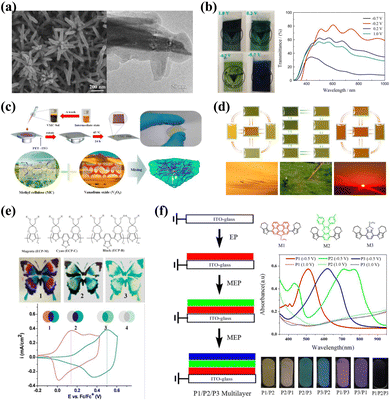 |
| Fig. 8 (a) SEM and TEM images and (b) photographs and transmittance spectra of a WO3/PANI core/shell nanowire array. Reproduced from ref. 118. Copyright 2014, Elsevier B.V. (c) Top: schematic of the spin-coating process. Bottom: morphology and structure of the VMC composite film. (d) Fully colored VMC RECDs that correspond to natural environments. Reproduced from ref. 119. Copyright 2022, American Chemical Society. (e) Repeat unit structures of the polymers, photographs of a multicolored “butterfly” ECD, and cyclic voltammograms of the polymers. Reproduced from ref. 123. Copyright 2011, American Chemical Society. (f) Left: schematic of the MEP for P1/P2/P3 Multilayers. Right: molecular structures of the monomers and absorbance spectra of polymers in the neutral state and oxidized state. Reproduced from ref. 126. Copyright 2021, Elsevier B.V. | |
In addition, the preparation of composites using two or more organic materials by solution blending or stacking strategies is a promising and straightforward idea for color modulation.120 The color mixing theory based on the subtraction model of cyan-magenta-yellow-black (CMY-K) and red-yellow-blue (RYB) has been widely used in the electrochromic field.121 It opens up endless possibilities for the use of organic EC materials, including military camouflage. John R. Reynolds's group has carried out many impressive studies on organic blend and stack materials.121,122 For example, they prepared “butterfly” EC composite films by stacking two ECP materials which display magenta and cyan, respectively.123 The repeat unit structure of the two conjugated polymers is presented in Fig. 8e. Following “color-mixing theory” principles, the two polymer stacks appear in black. As the voltage increased, the butterfly device exhibited multicolor switching due to the different oxidation voltages of the polymer film (Fig. 8e). Similar approaches were also reported by Jianguo Mei and coauthors.124 As reported in this paper, under ultraviolet light illumination, the EC polymer was cross-linked with the crosslinker to endow them with solvent-resistant properties, resulting in the preparation of multilayers. Moreover, the authors constructed a full-color palette by varying the film thickness of each ECP layer. Finally, the goal of color tuning could also be achieved by using the solubility of the ECPs material for solution mixing.125
In addition to the above-mentioned related studies, another classic method of tuning color is the preparation of multilayer composite films through multilayer electrochemical polymerization (MEP) technology, as reported by Cheng Zhang, Weijun Li, and coauthors (Fig. 8f).126 As shown in that work, the authors chose three EDOT-based monomers (M1–M3), which exhibited red, green, and blue colors after electrochemical polymerization. The selection of the monomers should take into account the polymerization voltage window of the monomer and the EC voltage window of the polymer. Then, the multilayer films based on three individual polymers were successfully deposited onto ITO-glass by the MEP technology. By controlling the thickness of each polymer layer, multilayers of various colors have been successfully prepared, including pleasing black EC film (Fig. 8f).
3.2 EC devices for color camouflage
The design and manufacture of ECDs are key steps toward the application of materials. In the conventional design, ECD is usually a “sandwich” structure consisting of two substrates, two conductive electrodes, an EC layer, an electrolyte layer, and an ion storage layer, making up a total of seven layers. The complex device structure also provides a margin for color adjustment and performance improvement. In the following sections, we will focus on EC camouflage devices and discuss their design strategies and underlying issues.
3.2.1 EC camouflage devices based on camouflage material.
Based on the existing yellow-to-green switching camouflage materials, scientists have designed and prepared many EC camouflage devices and demonstrated their feasibility in camouflage applications. One of the most straightforward strategies for camouflage devices is to use a camouflage material as the EC layer, followed by a colorless electrolyte and a colorless transparent substrate. Based on this strategy, Mario Leclerc et al.85 fabricated an EC camouflage cell by using conjugated polymer P1 as the EC layer and bare ITO as the counter electrode (Fig. 9a). The prepared device could achieve reversible change from yellow to green at applied voltages of 0.0 V and 2.4 V, which is the same color as that observed for thin film in solution. Finally, a hybrid plastic/textile EC cell was also prepared to demonstrate its application in wearable adaptive camouflage. However, the irreversibility of ITO redox leads to a large driving voltage and poor stability of the device.46 This makes it unsuitable for industrial applications of EC camouflage devices.
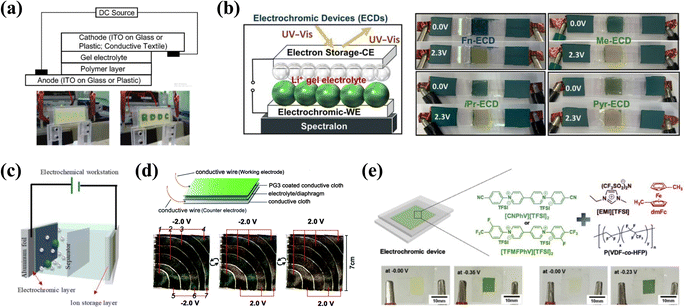 |
| Fig. 9 (a) Schematic and photographs of sandwich-type EC cells. Reproduced from ref. 85. Copyright 2006, American Chemical Society. (b) Left: schematic of the solid-state ECDs. Right: photographs of the ECD at the colored and bleached states. Reproduced from ref. 83. Copyright 2021, American Chemical Society. (c) Schematic of VMC RECDs. Reproduced from ref. 119. Copyright 2021, American Chemical Society. (d) Schematic and photographs of fabric chameleonic ECD. Reproduced from ref. 10 (CC BY 3.0). Copyright 2016, Royal Society of Chemistry. (e) Top: ECD configuration and molecular structures of components in the EC gels. Bottom: photographs of the ECD. Reproduced from ref. 80. Copyright 2017, Elsevier B.V. | |
To improve the EC performance of the camouflage device, an ion storage layer with excellent stability is required. Poly(3,4-ethylenedioxythiophene) (PEDOT) and poly(3,4-ethylenedioxythiophene)/poly(styrenesulfonate) (PEDOT: PSS) is commonly used as an ion storage layer due to its excellent electrochemical and chemical stability.127,128 Fatma Baycan Koyuncu et al.100 constructed a complementary ECD by using camouflage material poly(SNSPA) (P7) as the EC layer and PEDOT: PSS as the counter layer. The prepared device has a fast switching time (about 0.5 s) and lasting cyclic stability (98% of the optical contrast is retained after 1000 steps). For camouflage devices, the use of colored ion storage layers not only reduces the contrast of the device, but it is also not easy for the device to achieve a specific camouflage color or hue. Using colorless ion storage materials can effectively solve this problem. Olena V. Zenkina et al.83 assembled a solid-state EC camouflage device using TiO2 anatase transparent paste as the counter electrode (Fig. 9b). The result demonstrated that the device retained the color switching of EC materials while also showing high long-term durability (95% retention of the contrast after 3300 steps), fast response time (less than 4 s) and excellent coloration efficiency. In addition, other colorless ionic storage layers, such as inorganic oxides,129,130 poly(2,2,6,6-tetramethylpiperidinyloxy-4-ylmethacrylate) (PTMA) derivatives,131–133 and conjugated polymers,43,46 could also be applied to camouflage devices. Notably, the matched electroactive voltage window of the ion storage material and the EC material is also a major influence on device performance.45
In addition to the above-mentioned relevant strategies, Zhang Jinyong's group has reported a clever method for preparing reflective camouflage devices.119 In this work, a reflective camouflage device was fabricated with two pieces of VMC composite film, one of which served as the working electrode and the other was pre-built with Li+ as the counter electrode. To isolate color interference with the counter electrodes and achieve a high optical reflectivity of the device, the authors placed a white diaphragm between the working electrode and the counter electrode (Fig. 9c). In addition, aligning the EC layer laterally with the ion storage layer was also a strategy for preparing camouflaged devices, as reported by Meng Hong et al. (Fig. 9d).10
Unlike other EC materials, viologen is usually mixed with electrolytes to prepare integrated gel devices due to its solubility differences in different states.79 Hong Chul Moon et al.80 prepared an EC ion gel based on viologen derivative CPQ and TFMFPhV(TFSI)2, and dimethyl ferrocene (dmFc) was used as a counter redox material (Fig. 9e). It has been demonstrated that the addition of Fc could decrease the operating voltage and improve the cycle stability of the EC device.134 The prepared gel device achieves switching from yellow to green reversibly (Fig. 9e).
3.2.2 EC camouflage devices based on device design strategies.
Preparation of devices for color camouflage by preparing EC materials for camouflage color switching is tedious. In contrast, the direct use of device structures to prepare high-performance devices for camouflage applications is a simple and effective method. The electrodes, electrolytes, and substrates could all be optimally designed to tune and enhance the color and performance of the device. In the following sections, we will discuss and summarize these device design strategies in detail.
3.2.2.1 EC electrode color complementary.
The parallel connection of two or more devices using double-sided conductive electrodes is one of the most common means of achieving multicolor device preparation.135,136 John R. Reynolds et al.38 reported a multicolor prototype EC device based on soluble conjugated polymer. In this work, a dual-active EC device based on subtractive color mixing utilizing the cyan-magenta-yellow (CMY) primary system was prepared (Fig. 10a). Essentially, the dual-active device was the integration of two independently controlled EC devices into a pseudo-three-electrode setup since the two counter electrodes share a substrate. By controlling the voltage applied across each film, there were various color states accessible to the device. The dual-active structure device with ECP-cyan and ECP-yellow as working electrodes could be reversibly switched between a variety of yellows and greens (Fig. 10b). Similarly, there are other strategies to achieve this state, for instance, an employed three-stacked ECD reported by Jong S. Park, Sung Kyu Park and coauthors.136 The multi-stacked ECDs were implemented by the combinatorial stacking of the coloration devices, exhibiting a wide range of color adjustments, including black.
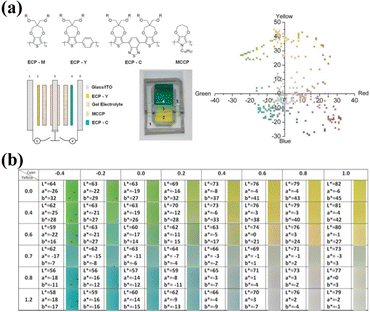 |
| Fig. 10 (a) Left: repeat unit structures of ECPs, and exploded view and photographs of a dual-active ECD. Right: plot of a*b* values of all color points recorded with dual-active devices. (b) Photographs and L*a*b* coordinates dual-active (bottom) cyan + yellow ECDs. Reproduced from ref. 38. Copyright 2014, American Chemical Society. | |
Although the multilayer device structure allows access to a wide gamut of color states, its complex structure makes it not the desired route in many applications. A dual EC electrode device is a much simpler solution, particularly for color camouflage applications where a single, particular color state is desirable. Typically, the EC layer plays a central role in devices, undertaking the task of optical modulation or color change. The ion storage layer acts as a counter electrode layer that undergoes reversible electrochemical oxidation (reduction) to balance the charge shuttling from the EC layer through the electrolyte without color change.45 However, in a dual EC electrode device, both electrodes are complementary to each other for the purpose of color adjustment.137,138 Based on the color stacking strategy, the device could achieve the desired multicolor switching capability through rational design. Additionally, the structure of the dual EC electrode device is conducive to enhancing the EC performance (optical contrast, stability, etc.) of the ECD through rational design.137,138
A similar method has been widely used to prepare high-performance yellow-green switching camouflage devices. Yao Li et al.139 prepared all-solid-state ECDs using WO3 and V2O5 as complementary electrodes (Fig. 11a). According to the subtractive color mixture theory, the all-solid-state EC device appears yellow-green at 2.5 V due to the transparent WO3 and yellow-green V2O5 in the bleached state. At −2.5 V, the all-solid-state ECD appears emerald green due to the mixing of blue WO3 and yellow V2O5 in the colored state. This well-designed ECD achieves a reversible color change between yellow-green and emerald green (Fig. 11a), as well as excellent cycle stability (>5000 cycles). The two complementary electrodes could not be controlled independently in complementary ECD, which significantly limits its multicolor performance. A Zn-based ECD was reported to efficiently solve this problem by Abdulhakem Y. Elezzabi, Haizeng Li, and coauthors.140 The two sodium vanadium oxide electrodes could be colored and bleached independently due to the introduction of the Zn sandwich (Fig. 11b). Due to the color superposition of two electrodes, the prepared ECD achieved a reversible switch between six colors (including a variety of yellow and green colors) (Fig. 11b). To further broaden the color palettes of devices, WO3 and sodium vanadium oxide films were selected as two asymmetric electrodes in the device.141 As shown in Fig. 11c, the Zn-anode-based EC devices of dual EC electrodes could switch between 16 colors based on the color overlay effect. In essence, the device could display all the color shades within the enclosed area, including a wide range of green and yellow hues (Fig. 11c).
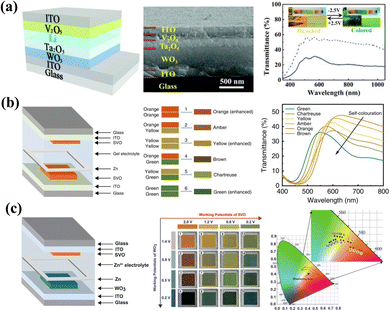 |
| Fig. 11 (a) Structural schematic, cross-sectional SEM image, and transmittance curves of the ECD. Reproduced from ref. 139. Copyright 2020, Royal Society of Chemistry. (b) Schematic of the Zn-SVO ECD, and schematic and transmittance spectra of the color overlay effect. Reproduced from ref. 140 (CC BY 4.0). Copyright 2020, Springer Nature. (c) Schematic, digital images and CIE color coordinates of the SVO-Zn-WO3 ECD. Reproduced from ref. 141. Copyright 2022, Wiley-VCH GmbH. | |
In addition, it has been shown that dual polymer ECDs using two complementary ECP electrodes could enhance overall EC performance due to their electrochemical reversibility and compatibility with the polymer electrodes. Cheng Zhang and Weijun Li et al.11 reported a dual conjugated polymer ECD. In this report, the authors synthesized two soluble EC materials that possess a yellow-to-transmissive and green-to-transmissive electrochromism, respectively (Fig. 12a). At the same time, the two ECPs prepared have approximate electrochromic potentials, which is an essential factor for the preparation of the desired dual conjugated polymer ECDs with good stability and the expected switch color change. Then, the dual conjugated polymer ECD with P1 and P2 as complementary electrodes was fabricated (Fig. 12a). This well-designed ECD presented yellow-to-green electrochromism, which showed excellent EC performances, including low driving voltage, fast switching speed, and lasting cyclic stability (Fig. 12b). Finally, the patterned and flexible dual-conjugated polymer devices demonstrated promising applications in color camouflage (Fig. 12c). This work is an important step towards enabling enhanced capabilities for EC color camouflage.
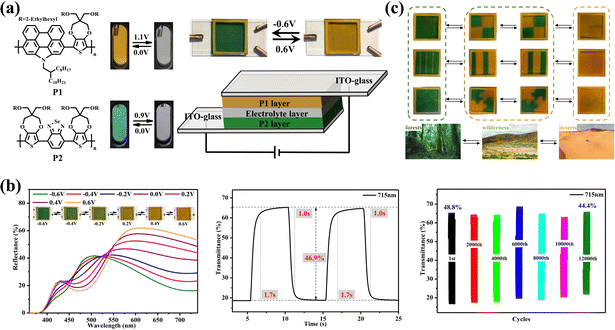 |
| Fig. 12 (a) Left: structure of polymer and colors of the neutral and oxidized states of the film. Right: the structure and color of yellow-to-green ECDs. (b) EC properties of yellow-to-green ECDs. (c) Military camouflage ECDs. Reproduced from ref. 11. Copyright 2022, Elsevier B.V. | |
3.2.2.2 Electrolyte coloring engineering.
Similarly, the color of the electrolyte layer can be introduced to broaden the palette of EC devices. Hong Meng, Xing Xing, and coauthors reported EC smart windows based on a temperature-stimulated phase hydrogel electrolyte.142 In this work, the electrolyte layer could achieve a highly scattered zero-transparent private state controlled by temperature. Thus, the EC devices could also switch between a nontransparent “white private state” (only scattered white light is transmitted) and an “absolute private state” (dark, zero-transmission state). It provides a new strategy for the design of EC device engineering. Furthermore, they proposed a strategy by introducing a thermochromic electrolyte so that the electrolyte layer and the EC layer share responsibility for the color change of the devices (Fig. 13a).143 It not only provides a strategy for color tuning of ECDs but is also a viable idea for high-performance yellow-to-green switching camouflage.
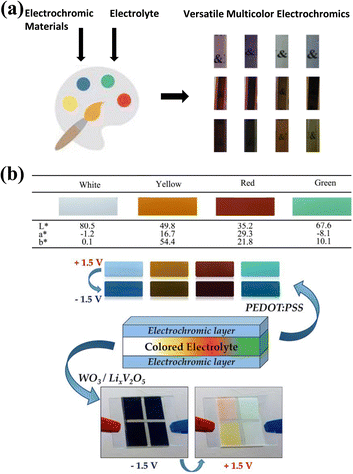 |
| Fig. 13 (a) Digital images of the ECD with the thermochromic electrolyte. Reproduced from ref. 143. Copyright 2022, Wiley-VCH GmbH. (b) Top: photographs and L*a*b* coordinates of electrolytes. Bottom: digital images of the ECD with the colored electrolytes. Reproduced from ref. 144. Copyright 2022, Elsevier B.V. | |
In addition, the direct introduction of colored electrolytes without the function of color change to achieve color tuning in EC devices is a much simpler and more effective method, as reported by Aline Rougier and coauthors.144 In this work, the authors tuned the color of the electrolyte by mixing four pigments into a gel polymer electrolyte, which resulted in four different colors of the electrolyte (white, yellow, red, and green) (Fig. 13b). In the white electrolyte device, the device could switch reversibly between blue and light blue, showing the color change of the PEDOT:
PSS. Notably, the high opacity of the white electrolyte isolates the two EC layers from color interference, and at the same time, produces a large reflection value, which is very favorable for reflective-type camouflage devices. Introducing a white diaphragm into the electrolyte could also achieve this goal.119 In other color electrolyte devices, depending on the electrolyte, different colors could be observed when the device is in the oxidized and reduced states. By changing the pigment color, a colored opacity electrolyte could be imagined and integrated into the camouflage device to achieve color tuning.
3.2.2.3 Complementary substrate colors.
EC camouflage devices are reflective-type devices and do not require a transparent substrate layer like transmissive-type devices (e.g., smart windows). The color of the substrate layer of the device can also be cleverly used. The use of yellow gold/nylon 66 porous substrates in military camouflage devices was reported by Yao Li and coauthors.145 In their work, a flexible bio-inspired chameleon skin EC device was assembled with WO3 porous film (Fig. 14a). As we all know, WO3 film can realize the reversible color switch between transparent and blue by applying voltage. According to the subtractive color mixture theory, the ECD they prepared could achieve a reversible transition from yellow to green. Meanwhile, under different applied voltages, various tones of green could be obtained according to the blue tones of WO3 (Fig. 14b). They achieved the same result by using Prussian blue (PB) (transparent to blue) with a similar strategy.146
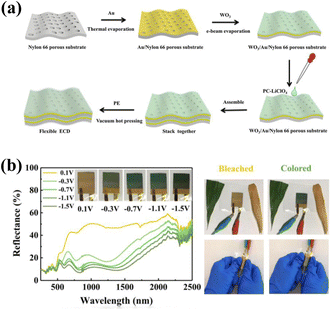 |
| Fig. 14 (a) Schematic for the assembling of flexible ECD. (b) Reflectance spectra and digital photos of the flexible ECD at different voltages. Reproduced from ref. 145. Copyright 2021, Elsevier B.V. | |
3.2.3 Preparation of wearable fabric devices.
Unlike other EC applications (e.g., smart windows, displays, etc.), the design and fabrication of high-performance large-area wearable fabric devices are critical for EC adaptive camouflage applications. Many practical and clever device structures and preparation techniques have been reported to achieve this goal. The commonly used strategies for preparing wearable fabric EC devices include (i) depositing EC film directly on fabric substrates147,148 and (ii) preparing fibrous EC devices.149,150
Gregory A. Sotzing et al.151 reported a stretchable e-textile by simply soaking Spandex fabric in a PEDOT-PSS aqueous dispersion. The electrical conductivity of the fabric varies between 0.1 and 2.0 S cm−1 due to the substrate; the lower conductivity also leads to the slow response (color change) of the EC fabric. To efficiently solve this problem and further improve the response of fabric devices, they developed a modified reflective-type fabric device architecture using steel counters as the two electrodes (Fig. 15a).152 In this device, the EC polymer switches between different colored states at a time of ca. 0.3 s due to the high conductivity (ca. 9800 S cm−1).
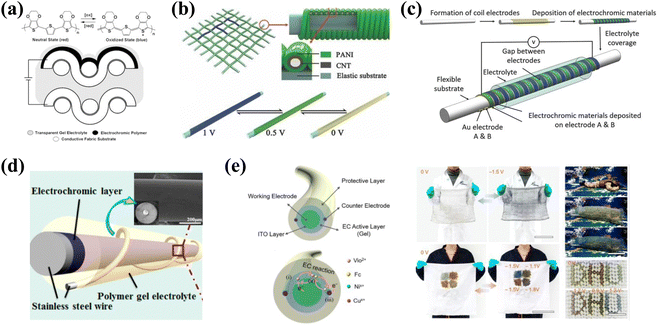 |
| Fig. 15 (a) Top: structure of the EC polymer. Bottom: cross-section schematic of fabric ECD. Reproduced from ref. 152. Copyright 2010, American Chemical Society. (b) Schematic of the EC, wearable fiber-shaped supercapacitor. Reproduced from ref. 153. Copyright 2014, John Wiley and Sons. (c) Schematic of parallel coil electrodes. Reproduced from ref. 154. Copyright 2014, Wiley-VCH GmbH. (d) Structure diagram of the EC fibers. Reproduced from ref. 149. Copyright 2014, American Chemical Society. (e) Left: structural scheme and schematic representation of electrochemical reactions of the EC fibers. Right: multifunctional applications of EC fibers. Reproduced from ref. 150. Copyright 2014, American Chemical Society. | |
Compared with conventional fabric-based structure devices, fiber-based EC devices offer many unique and promising advantages, such as a higher integration capability and the ability to be processed by conventional weaving, spinning, or knitting techniques. Huisheng Peng et al.153 fabricated a fiber-shaped EC supercapacitor via electro-depositing polyaniline (PANI) onto sheets of aligned carbon nanotubes (CNT) as two electrodes (Fig. 15b). The as-prepared fiber-shaped EC supercapacitor could be further woven into fabrics to display designed patterns. Furthermore, fiber-based devices could make rapid and reversible transitions between blue, green, and light yellow under different applied voltages (Fig. 15b). Another approach worthy of attention is a design strategy based on lateral device structures, as reported by Tong Lin and coworkers.154 The required dual helix electrodes were prepared by depositing tungsten oxide and poly(3-methylthiophene) onto two electrodes, respectively (Fig. 15c). The results showed that the lateral helix fiber devices could reversibly switch between dark red, green, and gold at the different applied potentials. Furthermore, the fiber devices demonstrated high flexibility and fast response. This is expected to be useful for the development of smart apparel and adaptive military camouflage in the future.
In addition to the above-mentioned related studies, Hongzhi Wang's group performed many impressive studies on EC fiber-based devices.149,150 They prepared fiber devices using stainless steel wires (SSWs) as a substrate (Fig. 15d).149 Three RGB EC materials were deposited on the surface of SSWs by electrochemical polymerization. The EC layer was then coated with a polymer gel electrolyte, and finally, another finer stainless steel wire was screwed on top as a counter electrode. These well-designed EC fibers could reversibly switch between sky blue and dark blue (PEDOT), blue and red (poly(3-methyl-thiophene) (P3MT)), and green and yellow (poly(2,5-dimethoxyaniline) (PDMA)), respectively. Furthermore, to make fiber devices more practical, they demonstrated the preparation of hundreds of meters of EC fibers based on a parallel dual-counter-electrode structure using custom-built equipment (Fig. 15e).150 The use of a parallel dual-counter-electrode structure allows for a more even distribution of the electric field and faster electron transfer, and improved the time and uniformity of the color change. Finally, the preparation of large-area smart color-changing textiles demonstrates their potential application in the field of EC color camouflage (Fig. 15e).
4. EC materials and devices for thermal camouflage
With the joint efforts of many researchers, research on EC materials and devices is not limited to the visible light field. Recently, infrared (IR) EC materials and devices have been extensively studied due to their great potential for applications in smart windows, thermal camouflage, etc.6–8,155 Unlike the color camouflage discussed earlier, thermal camouflage can achieve infrared concealment of a target by tuning the thermal radiation to be the same or similar to the background environment.156 According to the Stefan–Boltzmann law, the regulation of thermal radiation can be achieved by tuning either the local temperature field or the local emissivity of the target. Among them, EC thermal camouflage is achieved by adjusting the emissivity. Due to atmospheric windows, most infrared detectors operate in the mid-infrared (MIR) range within two wavelengths ranges, 3–5 μm and 8–13 μm. Thus, the modulation range of an expected camouflage material should encompass these wavelengths and exhibit the greatest modulation capability. The currently reported IR emissivity modulated EC materials can be divided into inorganic EC materials such as tungsten oxide (WO3),157–159 lithium titanate (Li4Ti5O12),160 and carbon materials,161,162etc., and organic EC materials such as polyaniline (PANI).163,164 In this section, we briefly describe recent advances in inorganic and organic EC materials for thermal camouflage EC materials and devices.
WO3 is a promising candidate for thermal camouflage applications. In 1999, John A. Woollam et al.165 studied the emissivity of semicrystalline WO3 and NiO in their unintercalated and fully intercalated states within the range of 2–14 μm. The authors obtained a large modulation range and a high modulation of the IR emissivity (Δε = 0.538) by adjusting the thickness of the NiO and WO3 film. A. Rougier et al.158 studied the IR modulation properties of WO3 film in detail. As reported in this paper, deposition conditions, including the duration of deposition, the substrate natures, and the chamber pressure, have an important influence on the IR properties of WO3 films. Controlling the crystallinity and micro-structure are the two main ways to improve the emissivity modulation of WO3. Yao Li et al.159 studied the effects of crystallinity on the IR emittance of WO3 film by preparing amorphous WO3 (a-WO3) and crystalline WO3 (c-WO3). The results indicate that the c-WO3 all-solid-state EC device showed a higher emittance regulation of 0.37 in the 8–14 μm range and 0.3 in the 2.5–25 μm range (Fig. 16a). J.P. Tu, G.F. Cai, and coauthors reported vertically aligned hierarchical WO3 nano-architectures.166 The nanostructured array film with thicknesses of about 1.1 μm show remarkable enhancement of the EC properties in the IR range.
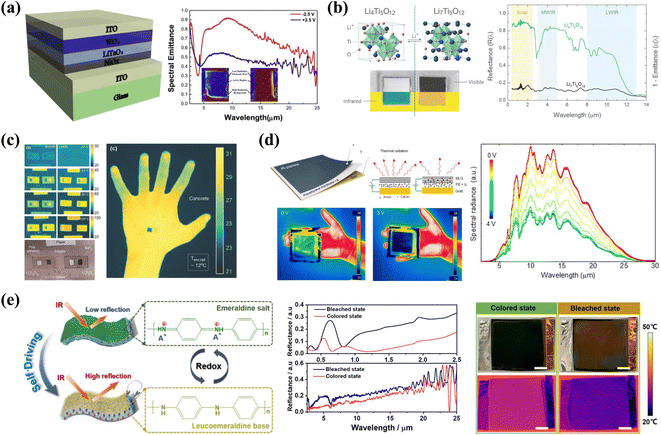 |
| Fig. 16 (a) Left: schematic of ECD structure. Right: spectral emittance curves and IR optical photographs of ECDs. Reproduced from ref. 159. Copyright 2019, Elsevier B.V. (b) Crystal structures, photographs, and visible-to-infrared spectral reflectance of Li4Ti5O12 and Li7Ti5O12 nanoparticles. (c) Left: MWIR and LWIR thermographs of LTO. Right: LWIR thermograph of a hand, with PE-coated L and DL LTO, held against concrete. Reproduced from ref. 160. Copyright 2018, Wiley-VCH GmbH. (d) Left: schematic drawing and the working principle schematic of the active thermal surface. Right: spectra of the thermal radiation from the device at different bias voltages. Reproduced from ref. 13. Copyright 2018, American Chemical Society. (e) Self-driven behavior and IR reflectance management of the IR-ECD. Reproduced from ref. 164. Copyright 2021, American Chemical Society. | |
In 1995, Tsutamu Ohzuku, Atsushi Ueda, and coauthors167 reported the color change during the transition from the Li4Ti5O12 phase (white) to the Li7Ti5O12 phase (blue) upon lithium insertion. With further research, the light modulation properties of Li4Ti5O12 extend from the VIS to the IR region, suggesting that Li4Ti5O12 is also a promising EC material for thermal camouflage.168 Yuan Yang et al.160 reported the broadband EC properties of Li4Ti5O12 and further investigated its potential in thermal camouflage applications. Upon Li+ intercalation, the transition of LTO from the delithiated (DL) state (Li4Ti5O12) to the lithiated (L) state (Li7Ti5O12), resulting in a drastic change in the electromagnetic properties and thus in the optical properties between the two states in the VIS to IR range (Fig. 16b). The results demonstrated that the Li4Ti5O12 nanoparticles electrodes showed large emissivity tunability of 0.74, 0.68, and 0.30 in the solar reflectance, mid-wave infrared emittance (3–5 μm), and long-wave infrared emittance (8–13 μm), respectively (Fig. 16b). Finally, the authors also tested the temperature regulation range of the LTO-based electrode (Fig. 16c).
Carbon materials such as graphene and carbon nanotubes have tunable Fermi energy levels and excellent electrical conductivity, making them suitable candidates for EC thermal camouflage materials.161,162 Coskun Kocabas et al.13 reported multilayer graphene that enables modulation of IR emissivity by reversible insertion of non-volatile ionic liquids under the action of electricity (Fig. 16d). The emissivity of the multilayer graphene electrode could be switched between high (0.8) and low states (0.3) at bias voltage while also exhibiting a fast switching time (<1 s). To achieve the preparation of large-area EC thermal camouflage devices, a sandwich-structured thin film based on multiwalled carbon nanotubes (MWCNTs) was reported by Lin Xiao and coauthors.162 The prepared film demonstrates efficient EC modulation with a thermal emissivity in the range of about 0.15–0.7. In addition, excellent cycling stability (optical modulation retains 89% after 3500 steps) was obtained in this film. Mid-infrared ECDs based on MWCNT films could achieve large-area preparation and superior EC performance, such as large modulation depth and high stability, and are expected to be used in adaptive thermal camouflage.
During the reversible electrochemical oxidation and reduction process, the conducting polymers generally exhibit strong IR absorption bands due to the formation of polarons and bipolarons.169 The formation of polarons results in absorption peaks usually in the VIS or near-infrared (NIR) region, while the absorption peaks resulting from bipolarons formation may be in the MIR range or even the far-infrared (FIR) range. The resulting modulation of emissivity in the MIR region shows attractive application potential in thermal camouflage.
PANI demonstrates excellent emissivity modulation capabilities in the MIR spectral range, making it already show significant promise in thermal camouflage. Researchers have been researching the properties of PANI to modulate in the MIR since as early as 1999. P. Topart et al.170 reported an IR-modulated EC device using PANI film as the active layer. The assembled device demonstrated properties of excellent MIR modulation during the doping/dedoping process, which realized a reflectivity variation of about 0.45 at 12 μm for potentials from −0.2 to 0.45 V. Subsequently, Prasanna Chandrasekhar and coauthors94 fabricated a two-electrode flexible all-solid-state device capable of 0.4 through 45 μm region modulation. The as-prepared device demonstrated high emittance variation from 0.32 to 0.79 (Δε = 0.4) in the range of 2.5 to 45 μm.
An in-depth study of the IR EC mechanism of PANI is necessary to improve the IR modulation efficiency for its application in thermal camouflage. Many groups have contributed significantly to the mechanistic study of the IR electrochromic of PANI.171 Yao Li and Jiupeng Zhao et al.95 demonstrated that the IR emissivity modulation of PANI film was achieved by the formation and elimination of polarons and bipolarons delocalized in the PANI chains by investigating PANI porous film in different conjugated and correspondingly dedoping states. Furthermore, there are many factors that affect the modulation of IR emissivity of PANI materials and devices,97 such as acid dopants, thickness, and morphology of PANI film, substrate, etc. Yao Li's group has done a lot of work in this area.163,172,173 Considering the continuous energy consumption required to run the drive, flexible self-driven IR EC devices with variable optical and thermal management were reported by Qianqian Zhang, Hao Wang, and coauthors (Fig. 16e).164 The as-prepared self-driven IR devices showed a reflectance contrast of 21.2%, fast switching, and a high CE of 93.6 cm2 C−1 at 1500 nm, which also demonstrated tunable thermal management (Fig. 16e).
5. Conclusion and Perspective
During recent decades, EC technology has made impressive advances in camouflage applications due to its unique advantages. In this review, recent advances in EC camouflage technology have been reviewed and discussed in detail in terms of color camouflage and thermal camouflage. In EC color camouflage, we systematically summarized strategies for the preparation of camouflage devices from two aspects: materials design and device design. There are three main design strategies for material design: introduction of structural colors, design of molecular structures, and preparation of composite materials. Corresponding camouflage material structures were comprehensively introduced and the possible device design strategies for EC camouflage were systemically discussed. In addition, the design ideas and advantages of these strategies were also discussed in detail, which can provide essential guidance for further design of high-performance camouflage materials and devices in the future. For EC thermal camouflage, we briefly illustrated the classification of thermal camouflage materials and summarized the applications of these IR-modulated materials in camouflage.
Due to the unremitting efforts of outstanding scientists, EC camouflage technology has achieved remarkable progress in recent years. Nonetheless, there are still some critical issues that must be considered and addressed before the real-world application of EC camouflage becomes feasible. First, for color camouflage, the color switch of the EC device needs to match the camouflage color and ensure as many color switches as possible. The currently reported EC materials and devices have been validated for color camouflage applications only by image comparison, which is far from sufficient. To improve the problem, the desired camouflage effect was demonstrated by comparison with standard camouflage colors (L*a*b* values and reflectance spectra). Meanwhile, some critical EC properties for practical camouflage applications, such as cycle stability, need to be further enhanced. Furthermore, the weatherabilities are also critical parameters. However, no relevant research has been reported in the field of EC camouflage. Second, the fabrication process of high-performance wearable fabric devices is scarce. Currently, there are two main strategies for preparing EC wearable fabric devices, one is to use fabric as a substrate, and the other is to prepare fiber-shaped EC devices and then further prepare them with textile technology. The EC devices using fabric as a substrate demonstrate better flexibility than ordinary EC devices; however, their poor breathability, adhesion, and implantability limit their application in camouflage clothing and fabrics. These problems could be solved by the strategy of preparing fiber-shaped devices; however, the nonuniform electric field distribution and long electron transfer pathway lead to very slow color switching of the devices. Lastly, multi-band camouflage remains an unmet key goal, as EC devices with color camouflage while maintaining high IR emissivity modulation have rarely been realized. However, with the continuous progress and development of reconnaissance technology, the development of multi-band compatible EC camouflage materials and devices is an inevitable trend. On the one hand, more attention should be paid to the exploitation and optimization of suitable EC materials with multi-band modulation capabilities, such as polyaniline (PANI). On the other hand, combining materials with different modulation ranges or designing multilayer device structures is also a viable approach when the performance of a single material cannot meet the requirements. We anticipate that the abovementioned challenges and considerations proposed in this review will provide valuable guidance for constructing high-performance EC camouflage devices for real-world applications.
Author contributions
Haichang Fu: investigation and writing the original draft. Ling Zhang: revising the manuscript. Yujie Dong: revising the manuscript. Cheng Zhang: supervision and visualization. Weijun Li: editing and revising the manuscript, conceiving the ideas, and supervision. All authors discussed the results and commented on the manuscript.
Conflicts of interest
There are no conflicts to declare.
Acknowledgements
This work was supported by the National Natural Science Foundation of China (52103232 and 51603185) and the Natural Science Foundation of Zhejiang Province (LY19E030006 and LQ19E030016).
Notes and references
- Y. Qu, Q. Li, L. Cai, M. Pan, P. Ghosh, K. Du and M. Qiu, Thermal camouflage based on the phase-changing material GST, Light: Sci. Appl., 2018, 7, 26 CrossRef PubMed
.
- J. Lee, H. Sul, Y. Jung, H. Kim, S. Han, J. Choi, J. Shin, D. Kim, J. Jung, S. Hong and S. H. Ko, Thermally Controlled, Active Imperceptible Artificial Skin in Visible-to-Infrared Range, Adv. Funct. Mater., 2020, 30, 2003328 CrossRef CAS
.
- Y. Zhao, D. Fan and Q. Li, Deformable manganite perovskite-based resonator with adaptively modulating infrared radiation, Appl. Mater. Today, 2020, 21, 100808 CrossRef
.
- R. Pardo, M. Zayat and D. Levy, Photochromic organic-inorganic hybrid materials, Chem. Soc. Rev., 2011, 40, 672–687 RSC
.
- Z. J. Coppens and J. G. Valentine, Spatial and Temporal Modulation of Thermal Emission, Adv. Mater., 2017, 29, 1701275 CrossRef PubMed
.
- J. Niu, Y. Wang, X. Zou, Y. Tan, C. Jia, X. Weng and L. Deng, Infrared electrochromic materials, devices and applications, Appl. Mater. Today, 2021, 24, 101073 CrossRef
.
- H. Wei, J. Gu, F. Ren, L. Zhang, G. Xu, B. Wang, S. Song, J. Zhao, S. Dou and Y. Li, Smart Materials for Dynamic Thermal Radiation Regulation, Small, 2021, 17, 2100446 CrossRef CAS PubMed
.
- H. Gong, W. Li, G. Fu, Q. Zhang, J. Liu, Y. Jin and H. Wang, Recent progress and advances in electrochromic devices exhibiting infrared modulation, J. Mater. Chem. A, 2022, 10, 6269–6290 RSC
.
- L. Xiao, H. Ma, J. Liu, W. Zhao, Y. Jia, Q. Zhao, K. Liu, Y. Wu, Y. Wei, S. Fan and K. Jiang, Fast Adaptive Thermal Camouflage Based on Flexible VO2/Graphene/CNT Thin Films, Nano Lett., 2015, 15, 8365–8370 CrossRef CAS PubMed
.
- H. Yu, S. Shao, L. Yan, H. Meng, Y. He, C. Yao, P. Xu, X. Zhang, W. Hu and W. Huang, Side-chain engineering of green color electrochromic polymer materials: toward adaptive camouflage application, J. Mater. Chem. C, 2016, 4, 2269–2273 RSC
.
- H. Fu, S. Yan, T. Yang, M. Yin, L. Zhang, X. Shao, Y. Dong, W. Li and C. Zhang, New dual conjugated polymer electrochromic device with remarkable yellow-to-green switch for adaptive camouflage, Chem. Eng. J., 2022, 438, 135455 CrossRef CAS
.
- B. Wang, Y. Huang, Y. Han, W. Zhang, C. Zhou, Q. Jiang, F. Chen, X. Wu, R. Li, P. Lyu, S. Zhao, F. Wang and R. Zhang, A Facile Strategy To Construct Au@VxO2x+1 Nanoflowers as a Multicolor Electrochromic Material for Adaptive Camouflage, Nano Lett., 2022, 22, 3713–3720 CrossRef CAS PubMed
.
- O. Salihoglu, H. B. Uzlu, O. Yakar, S. Aas, O. Balci, N. Kakenov, S. Balci, S. Olcum, S. Suzer and C. Kocabas, Graphene-Based Adaptive Thermal Camouflage, Nano Lett., 2018, 18, 4541–4548 CrossRef CAS PubMed
.
- M. Laurenti, S. Bianco, M. Castellino, N. Garino, A. Virga, C. F. Pirri and P. Mandracci, Toward Plastic Smart Windows: Optimization of Indium Tin Oxide Electrodes for the Synthesis of Electrochromic Devices on Polycarbonate Substrates, ACS Appl. Mater. Interfaces, 2016, 8, 8032–8042 CrossRef CAS PubMed
.
- A. W. Lang, A. M. Österholm and J. R. Reynolds, Paper-Based Electrochromic Devices Enabled by Nanocellulose-Coated Substrates, Adv. Funct. Mater., 2019, 29, 1903487 CrossRef
.
- C. Wang, X. Jiang, P. Cui, M. Sheng, X. Gong, L. Zhang and S. Fu, Multicolor and Multistage Response Electrochromic Color-Memory Wearable Smart Textile and Flexible Display, ACS Appl. Mater. Interfaces, 2021, 13, 12313–12321 CrossRef CAS PubMed
.
- S. Zhang, S. Cao, T. Zhang, A. Fisher and J. Y. Lee, Al3+ intercalation/de intercalation-enabled dual-band electrochromic smart windows with a high optical modulation, quick response and long cycle life, Energy Environ. Sci., 2018, 11, 2884–2892 RSC
.
- C. Kortz, A. Hein, M. Ciobanu, L. Walder and E. Oesterschulze, Complementary hybrid electrodes for high contrast electrochromic devices with fast response, Nat. Commun., 2019, 10, 4874 CrossRef PubMed
.
- C. Park, S. Seo, H. Shin, B. D. Sarwade, J. Na and E. Kim, Switchable silver mirrors with long memory effects, Chem. Sci., 2015, 6, 596–602 RSC
.
- H. Shin, S. Seo, C. Park, J. Na, M. Han and E. Kim, Energy saving electrochromic windows from bistable low-HOMO level conjugated polymers, Energy Environ. Sci., 2016, 9, 117–122 RSC
.
- D. Bessinger, K. Muggli, M. Beetz, F. Auras and T. Bein, Fast-Switching Vis-IR Electrochromic Covalent Organic Frameworks, J. Am. Chem. Soc., 2021, 143, 7351–7357 CrossRef CAS PubMed
.
- Y. Luo, H. Jin, Y. Lu, Z. Zhu, S. Dai, L. Huang, X. Zhuang, K. Liu and L. Huang, Potential Gradient-Driven Fast-Switching Electrochromic Device, ACS Energy Lett., 2022, 7, 1880–1887 CrossRef CAS
.
- X. Lv, W. Li, M. Ouyang, Y. Zhang, D. S. Wright and C. Zhang, Polymeric electrochromic materials with donor–acceptor structures, J. Mater. Chem. C, 2017, 5, 12–28 RSC
.
- Z. Wang, X. Wang, S. Cong, F. Geng and Z. Zhao, Fusing electrochromic technology with other advanced technologies: A new roadmap for future development, Mater. Sci. Eng., R, 2020, 140, 100524 CrossRef
.
- G. Cai, J. Wang and P. S. Lee, Next-Generation Multifunctional Electrochromic Devices, Acc. Chem. Res., 2016, 49, 1469–1476 CrossRef CAS PubMed
.
- J. R. Platt, Electrochromism, a Possible Change of Color Producible in Dyes by an Electric Field, J. Chem. Phys., 1961, 34, 862–863 CrossRef CAS
.
- L. Qian, X. Lv, M. Ouyang, A. Tameev, K. Katin, M. Maslov, Q. Bi, C. Huang, R. Zhu and C. Zhang, Fast Switching Properties and Ion Diffusion Behavior of Polytriphenylamine Derivative with Pendent Ionic Liquid Unit, ACS Appl. Mater. Interfaces, 2018, 10, 32404–32412 CrossRef CAS PubMed
.
- Y. Kim, M. Han, J. Kim and E. Kim, Electrochromic capacitive windows based on all conjugated polymers for a dual function smart window, Energy Environ. Sci., 2018, 11, 2124–2133 RSC
.
- W. T. Neo, Q. Ye, Z. Shi, S.-J. Chua and J. Xu, Influence of catalytic systems in Stille polymerization on the electrochromic performance of diketopyrrolopyrrole-based conjugated polymers, Mater. Chem. Front., 2018, 2, 331–337 RSC
.
- N. Yan, W. Zhang, G. Li, S. Zhang, X. Yang, K. Zhou, D. Pei, Z. Zhao and G. He, AIE-active 9,10-azaboraphenanthrene-containing viologens for reversible electrochromic and electrofluorochromic applications, Mater. Chem. Front., 2021, 5, 4128–4137 RSC
.
- C. M. Lampert, Electrochromic materials and devices for energy efficient windows, Sol. Energy Mater., 1984, 11, 1–27 CrossRef CAS
.
- J. S. E. M. Svensson and C. G. Granqvist, Electrochromic tungsten oxide films for energy efficient windows, Sol. Energy Mater., 1984, 11, 29–34 CrossRef CAS
.
- Y. Ke, J. Chen, G. Lin, S. Wang, Y. Zhou, J. Yin, P. S. Lee and Y. Long, Smart Windows: Electro-, Thermo-, Mechano-, Photochromics, and Beyond, Adv. Energy Mater., 2019, 9, 1902066 CrossRef CAS
.
- H. Li, W. Zhang and A. Y. Elezzabi, Transparent Zinc-Mesh Electrodes for Solar-Charging Electrochromic Windows, Adv. Mater., 2020, 32, 2003574 CrossRef CAS PubMed
.
- J.-L. Wang, Y.-R. Lu, H.-H. Li, J.-W. Liu and S.-H. Yu, Large Area Co-Assembly of Nanowires for Flexible Transparent Smart Windows, J. Am. Chem. Soc., 2017, 139, 9921–9926 CrossRef CAS PubMed
.
- G. Cai, A. L.-S. Eh, L. Ji and P. S. Lee, Recent Advances in Electrochromic Smart Fenestration, Adv. Sustain. Syst., 2017, 1, 1700074 CrossRef
.
- C. Gu, A.-B. Jia, Y.-M. Zhang and S. X.-A. Zhang, Emerging Electrochromic Materials and Devices for Future Displays, Chem. Rev., 2022, 122, 14679–14721 CrossRef CAS PubMed
.
- R. H. Bulloch, J. A. Kerszulis, A. L. Dyer and J. R. Reynolds, Mapping the broad CMY subtractive primary color gamut using a dual-active electrochromic device, ACS Appl. Mater. Interfaces, 2014, 6, 6623–6630 CrossRef CAS PubMed
.
- R. J. Mortimer, A. L. Dyer and J. R. Reynolds, Electrochromic organic and polymeric materials for display applications, Displays, 2006, 27, 2–18 CrossRef CAS
.
- A. M. Österholm, D. E. Shen, J. A. Kerszulis, R. H. Bulloch, M. Kuepfert, A. L. Dyer and J. R. Reynolds, Four Shades of Brown: Tuning of Electrochromic Polymer Blends Toward High-Contrast Eyewear, ACS Appl. Mater. Interfaces, 2015, 7, 1413–1421 CrossRef PubMed
.
- Y. Wang, Z. Meng, H. Chen, T. Li, D. Zheng, Q. Xu, H. Wang, X. Y. Liu and W. Guo, Pulsed electrochemical deposition of porous WO3 on silver networks for highly flexible electrochromic devices, J. Mater. Chem. C, 2019, 7, 1966–1973 RSC
.
- H. Fu, Y. Tang, F. Zhan, L. Zhang, W. Zhan, Y. Dong, W. Li and C. Zhang, Dual polymer electrochromic sunglasses with black to anti-blue-ray conversion based on new anti-blue-ray transparent polymer, Chem. Eng. J., 2023, 461, 141848 CrossRef CAS
.
- E. P. Knott, M. R. Craig, D. Y. Liu, J. E. Babiarz, A. L. Dyer and J. R. Reynolds, A minimally coloured dioxypyrrole polymer as a counter electrode material in polymeric electrochromic window devices, J. Mater. Chem., 2012, 22, 4953–4962 RSC
.
- X. Tang, G. Chen, Z. Li, H. Li, Z. Zhang, Q. Zhang, Z. Ou, Y. Li, C. Qi and J. Luo, Structure evolution of electrochromic devices from ‘face-to-face’ to ‘shoulder-by-shoulder’, J. Mater. Chem. C, 2020, 8, 11042–11051 RSC
.
- D. Eric Shen, A. M. Österholm and J. R. Reynolds, Out of sight but not out of mind: the role of counter electrodes in polymer-based solid-state electrochromic devices, J. Mater. Chem. C, 2015, 3, 9715–9725 RSC
.
- L. Yu, X. Xing, D. Fang and H. Meng, Highly Transparent Conjugated Polymer as the Counter Electrode in Electrochromic Smart Windows, Adv. Opt. Mater., 2022, 10, 2201423 CrossRef CAS
.
- S.-Y. Kao, Y. Kawahara, S. Nakatsuji and K.-C. Ho, Achieving a large contrast, low driving voltage, and high stability electrochromic device with a viologen chromophore, J. Mater. Chem. C, 2015, 3, 3266–3272 RSC
.
- V. K. Thakur, G. Ding, J. Ma, P. S. Lee and X. Lu, Hybrid materials and polymer electrolytes for electrochromic device applications, Adv. Mater., 2012, 24, 4071–4096 CrossRef CAS PubMed
.
- W. Kang, C. Yan, C. Y. Foo and P. S. Lee, Foldable Electrochromics Enabled by Nanopaper Transfer Method, Adv. Funct. Mater., 2015, 25, 4203–4210 CrossRef CAS
.
- J. Teyssier, S. V. Saenko, D. Van Der Marel and M. C. Milinkovitch, Photonic crystals cause active colour change in chameleons, Nat. Commun., 2015, 6, 6368 CrossRef CAS PubMed
.
- Y. Qiao, Z. Meng, P. Wang and D. Yan, Research Progress of Bionic Adaptive Camouflage Materials, Front. Mater., 2021, 8, 637664 CrossRef
.
- Q. Wu, X. Wang, P. Sun, Z. Wang, J. Chen, Z. Chen, G. Song, C. Liu, X. Mu, S. Cong and Z. Zhao, Electrochromic Metamaterials of Metal-Dielectric Stacks for Multicolor Displays with High Color Purity, Nano Lett., 2021, 21, 6891–6897 CrossRef CAS PubMed
.
- C. Hu, L. Li, J. Zhou, B. Li, S. Zhao and C. Zou, Enhanced Contrast of WO3-Based Smart Windows by Continuous LiIon Insertion and Metal Electroplating, ACS Appl. Mater. Interfaces, 2022, 14, 32253–32260 CrossRef CAS PubMed
.
- J. Chen, Z. Wang, C. Liu, Z. Chen, X. Tang, Q. Wu, S. Zhang, G. Song, S. Cong, Q. Chen and Z. Zhao, Mimicking Nature's Butterflies: Electrochromic Devices with Dual-Sided Differential Colorations, Adv. Mater., 2021, 33, 2007314 CrossRef CAS PubMed
.
- Y. Ding, M. Wang, Z. Mei and X. Diao, Novel Prussian White@MnO2-Based Inorganic Electrochromic Energy Storage Devices with Integrated Flexibility, Multicolor, and Long Life, ACS Appl. Mater. Interfaces, 2022, 14, 48833–48843 CrossRef CAS PubMed
.
- L. Beverina, G. A. Pagani and M. Sassi, Multichromophoric electrochromic polymers: colour tuning of conjugated polymers through the side chain functionalization approach, Chem. Commun., 2014, 50, 5413–5430 RSC
.
- P. M. Beaujuge, C. M. Amb and J. R. Reynolds, Spectral Engineering in π-Conjugated Polymers with Intramolecular Donor-Acceptor Interactions, Acc. Chem. Res., 2010, 43, 1396–1407 CrossRef CAS PubMed
.
- P. M. Beaujuge and J. R. Reynolds, Color Control in π-Conjugated Organic Polymers for Use in Electrochromic Devices, Chem. Rev., 2010, 110, 268–320 CrossRef CAS PubMed
.
- K. Xiong, G. Emilsson, A. Maziz, X. Yang, L. Shao, E. W. H. Jager and A. B. Dahlin, Plasmonic Metasurfaces with Conjugated Polymers for Flexible Electronic Paper in Color, Adv. Mater., 2016, 28, 9956–9960 CrossRef CAS PubMed
.
- S. Yan, Y. Dong, W. Li, L. Chen, Y. Dai, N. Ren, Y. Wu, Y. Zhang and C. Zhang, Electrochemical and electrochromic properties of bilayer polymer films prepared by electrochemical polymerization based on star-shaped thiophene derivatives, New J. Chem., 2019, 43, 9566–9573 RSC
.
- N. Jiang, X. Zhuo and J. Wang, Active Plasmonics: Principles, Structures, and Applications, Chem. Rev., 2018, 118, 3054–3099 CrossRef CAS PubMed
.
- S. Daqiqeh Rezaei, Z. Dong, J. You En Chan, J. Trisno, R. J. H. Ng, Q. Ruan, C.-W. Qiu, N. A. Mortensen and J. K. W. Yang, Nanophotonic Structural Colors, ACS Photonics, 2020, 8, 18–33 CrossRef
.
- B. Yang, H. Cheng, S. Chen and J. Tian, Structural colors in metasurfaces: principle, design and applications, Mater. Chem. Front., 2019, 3, 750–761 RSC
.
- J. Chen, Y. Li, T. Zhang, X. Zha, X. Tang, X. Mu, P. Sun, G. Song, S. Cong, Q. Chen and Z. Zhao, Reversible Active Switching of Fano and Fabry-Pérot Resonances by Electrochromic Operation, Laser Photonics Rev., 2022, 16, 2200303 CrossRef CAS
.
- Z. Wang, X. Wang, S. Cong, J. Chen, H. Sun, Z. Chen, G. Song, F. Geng, Q. Chen and Z. Zhao, Towards full-colour tunability of inorganic electrochromic devices using ultracompact Fabry-Perot nanocavities, Nat. Commun., 2020, 11, 302 CrossRef CAS PubMed
.
- M. Gugole, O. Olsson, K. Xiong, J. C. Blake, J. Montero Amenedo, I. Bayrak Pehlivan, G. A. Niklasson and A. Dahlin, High-Contrast Switching of Plasmonic Structural Colors: Inorganic versus Organic Electrochromism, ACS Photonics, 2020, 7, 1762–1772 CrossRef CAS
.
- Y. Li, J. van de Groep, A. A. Talin and M. L. Brongersma, Dynamic Tuning of Gap Plasmon Resonances Using a Solid-State Electrochromic Device, Nano Lett., 2019, 19, 7988–7995 CrossRef CAS PubMed
.
- A. Tsuboi, K. Nakamura and N. Kobayashi, A localized surface plasmon resonance-based multicolor electrochromic device with electrochemically size-controlled silver nanoparticles, Adv. Mater., 2013, 25, 3197–3201 CrossRef CAS PubMed
.
- F. Neubrech, X. Duan and N. Liu, Dynamic plasmonic color generation enabled by functional materials, Sci. Adv., 2020, 6, eabc2709 CrossRef CAS PubMed
.
- E. Redel, J. Mlynarski, J. Moir, A. Jelle, C. Huai, S. Petrov, M. G. Helander, F. C. Peiris, G. von Freymann and G. A. Ozin, Electrochromic Bragg mirror: ECBM, Adv. Mater., 2012, 24, OP265–OP269 CrossRef CAS PubMed
.
- L. Xiao, Y. Lv, J. Lin, Y. Hu, W. Dong, X. Guo, Y. Fan, N. Zhang, J. Zhao, Y. Wang and X. Liu, WO3-Based Electrochromic Distributed Bragg Reflector: Toward Electrically Tunable Microcavity Luminescent Device, Adv. Opt. Mater., 2018, 6, 1700791 CrossRef
.
- J. Peng, H.-H. Jeong, Q. Lin, S. Cormier, H.-L. Liang, M. F. L. D. Volder, S. Vignolini and J. J. Baumberg, Scalable electrochromic nanopixels using plasmonics, Sci. Adv., 2019, 5, eaaw2205 CrossRef CAS PubMed
.
- T. Xu, E. C. Walter, A. Agrawal, C. Bohn, J. Velmurugan, W. Zhu, H. J. Lezec and A. A. Talin, High-contrast and fast electrochromic switching enabled by plasmonics, Nat. Commun., 2016, 7, 10479 CrossRef CAS PubMed
.
- D. Zhang, J. Wang, Z. Tong, H. Ji and H. Y. Qu, Bioinspired Dynamically Switchable PANI/PS-b-P2VP Thin Films for Multicolored Electrochromic Displays with Long-Term Durability, Adv. Funct. Mater., 2021, 31, 2106577 CrossRef CAS
.
- K. Chen, J. He, D. Zhang, L. You, X. Li, H. Wang and J. Mei, Bioinspired Dynamic Camouflage from Colloidal Nanocrystals Embedded Electrochromics, Nano Lett., 2021, 21, 4500–4507 CrossRef CAS PubMed
.
- W. Li, M. Yin, J. Liu, H. Fu, X. Shao, Y. Dong, Q. Song, C. Zhang and W. Y. Wong, Reversible color modulation of luminescent conjugated polymers based on a chemical redox mechanism and applications in rewritable paper and multiple information encryption, Mater. Horiz., 2022, 9, 2198–2206 RSC
.
- X. Chen, Z. Xu, S. Mi, J. Zheng and C. Xu, Spray-processable red-to-transmissive electrochromic polymers towards fast switching time for display applications, New J. Chem., 2015, 39, 5389–5394 RSC
.
- Q. Huang, J. Chen, X. Shao, L. Zhang, Y. Dong, W. Li, C. Zhang and Y. Ma, New electropolymerized triphenylamine polymer films and excellent multifunctional electrochromic energy storage system materials with real-time monitoring of energy storage status, Chem. Eng. J., 2023, 461, 141974 CrossRef CAS
.
- H. C. Moon, C. H. Kim, T. P. Lodge and C. D. Frisbie, Multicolored, Low-Power, Flexible Electrochromic Devices Based on Ion Gels, ACS Appl. Mater. Interfaces, 2016, 8, 6252–6260 CrossRef CAS PubMed
.
- H. Oh, D. G. Seo, T. Y. Yun, S. B. Lee and H. C. Moon, Novel viologen derivatives for electrochromic ion gels showing a green-colored state with improved stability, Org. Electron., 2017, 51, 490–495 CrossRef CAS
.
- G. Li, R. Song, W. Ma, X. Liu, Y. Li, B. Rao and G. He, π-Extended chalcogenoviologens with stable radical state enable enhanced visible-light-driven hydrogen evolution and static/dynamic electrochromic displays, J. Mater. Chem. A, 2020, 8, 12278–12284 RSC
.
- C. Gu, X. Wang, A.-B. Jia, H. Zheng, W. Zhang, Y. Wang, M. Li, Y.-M. Zhang and S. X.-A. Zhang, A Strategy of Stabilization via Active Energy-Exchange for Bistable Electrochromic Displays, CCS Chem., 2022, 4, 2757–2767 CrossRef CAS
.
- N. O. Laschuk, I. I. Ebralidze, E. B. Easton and O. V. Zenkina, Post-Synthetic Color Tuning of the Ultra-Effective and Highly Stable Surface-Confined Electrochromic Monolayer: Shades of Green for Camouflage Materials, ACS Appl. Mater. Interfaces, 2021, 13, 39573–39583 CrossRef CAS PubMed
.
- R. Banasz, M. Kubicki and M. Walesa-Chorab, Yellow-to-brown and yellow-to-green electrochromic devices based on complexes of transition metal ions with a triphenylamine-based ligand, Dalton Trans., 2020, 49, 15041–15053 RSC
.
- S. Beaupre, J. Dumas and M. Leclerc, Toward the Development of New Textile/Plastic Electrochromic Cells Using Triphenylamine-Based Copolymers, Chem. Mater., 2006, 18, 4011–4018 CrossRef CAS
.
- S. Beaupre, A.-C. Breton, J. Dumas and M. Leclerc, Multicolored Electrochromic Cells Based On Poly(2,7-Carbazole) Derivatives For Adaptive Camouflage, Chem. Mater., 2009, 21, 1504–1513 CrossRef CAS
.
- Y. Dong, F. Luo, L. Chen, F. Yuan, Y. Hou, W. Li, S. Yan, Y. Dai, M. Ouyang and C. Zhang, Multi-color electrochromism containing green color based on electrochemically polymerized star-shaped phenyl bithiophene, Phys. Chem. Chem. Phys., 2018, 20, 12923–12928 RSC
.
- J. Ding, C. Zheng, L. Wang, C. Lu, B. Zhang, Y. Chen, M. Li, G. Zhai and X. Zhuang, Viologen-inspired functional materials: synthetic strategies and applications, J. Mater. Chem. A, 2019, 7, 23337–23360 RSC
.
- K. Madasamy, D. Velayutham, V. Suryanarayanan, M. Kathiresan and K.-C. Ho, Viologen-based electrochromic materials and devices, J. Mater. Chem. C, 2019, 7, 4622–4637 RSC
.
- Y. Wang, Y. M. Zhang and S. X. Zhang, Stimuli-Induced Reversible Proton Transfer for Stimuli-Responsive Materials and Devices, Acc. Chem. Res., 2021, 54, 2216–2226 CrossRef CAS PubMed
.
- R. G. Compton, A. M. Waller, P. M. S. Monk and D. R. Rosseinsky, Electron Paramagnetic Resonance Spectroscopy of Electrodeposited Species from Solutions of 1,1'= bis-(p-Cyanop henyl)-4,4'-bipyridiIium (Cyanophenyl Paraquat, CPQ), J. Chem. Soc., Faraday Trans., 1990, 86, 2583–2586 RSC
.
- D. R. Rosseinsky and P. M. S. Monk, Electrochromic cyanophenylparaquat (CPQ: 1,1'-bis-cyanophenyl-4,4'-bipyridilium)
studied voltammetrically, spectroelectrochemically and by ESR, Sol. Energ. Mat. Sol. C., 1992, 25, 201–210 CrossRef CAS
.
- Y. Kuai, W. Li, Y. Dong, W. Y. Wong, S. Yan, Y. Dai and C. Zhang, Multi-color electrochromism from coordination nanosheets based on a terpyridine-Fe(ii) complex, Dalton Trans., 2019, 48, 15121–15126 RSC
.
- P. Chandrasekhar, B. J. Zay, G. C. Birur, S. Rawal, E. A. Pierson, L. Kauder and T. Swanson, Large, Switchable Electrochromism in the Visible Through Far-Infrared in Conducting Polymer Devices, Adv. Funct. Mater., 2002, 12, 95–103 CrossRef CAS
.
- L. Zhang, B. Wang, X. Li, G. Xu, S. Dou, X. Zhang, X. Chen, J. Zhao, K. Zhang and Y. Li, Further understanding of the mechanisms of electrochromic devices with variable infrared emissivity based on polyaniline conducting polymers, J. Mater. Chem. C, 2019, 7, 9878–9891 RSC
.
- J. Niu, J. Zhang, Y. Wang, L. Hu, S. Tang, Z. Wan, C. Jia, X. Weng and L. Deng, A light-weight, thin-thickness, flexible multifunctional electrochromic device integrated with variable optical, thermal management and energy storage, Electrochim. Acta, 2022, 435, 141274 CrossRef CAS
.
- B. Li, Y. Chen, X. Liu, F. Li, J. Wang, Y. Li and N. Li, The Infrared Electrochromic Properties Based on Acid Doped-Polyaniline Films, Front. Mater., 2022, 9, 857395 CrossRef
.
- S. Yan, H. Fu, Y. Dong, W. Li, Y. Dai and C. Zhang, Synthesis, electrochemistry and electrochromic properties of donor-acceptor conjugated polymers based on swivel-cruciform monomers with different central cores, Electrochim. Acta, 2020, 354, 136672 CrossRef CAS
.
- Y. Tang, L. Zhang, S. Yan, Y. Kuai, H. Fu, W. Li, M. Ouyang and C. Zhang, Black-to-transmissive polymer films via electrochemical copolymerization with high-performance electrochromic and supercapacitor bifunction, Sol. Energ. Mat. Sol. C., 2022, 245, 111857 CrossRef CAS
.
- S. Koyuncu and F. B. Koyuncu, A new ITO-compatible side chain-functionalized multielectrochromic polymer for use in adaptive camouflage-like electrochromic devices, React. Funct. Polym., 2018, 131, 174–180 CrossRef CAS
.
- X. Shao, W. Li, Y. Kuai, Q. Huang, Y. Dong, C. Zhang, W.-Y. Wong and M. Ouyang, Organic soluble linear metallo-supramolecular polymer based on iron(II) and terpyridyl ligand with high electrochromic performance, Synthetic Met., 2021, 282, 116953 CrossRef CAS
.
- G. Cai, J. Chen, J. Xiong, A. L. Eh, J. Wang, M. Higuchi and P. S. Lee, Molecular Level Assembly for High-Performance Flexible Electrochromic Energy-Storage Devices, ACS Energy Lett., 2020, 5, 1159–1166 CrossRef CAS
.
- Y. Kuai, T. Yang, F. Yuan, Y. Dong, Q. Song, C. Zhang and W.-Y. Wong, Self-assembled flexible metallo-supramolecular film based on Fe(II) ion and triphenylamine-subsituted alkyl terpyridine towards electrochromic application, Dyes Pigments, 2021, 194, 109623 CrossRef CAS
.
- Y. Zhu, W. Zheng, W. Wang and H.-B. Yang, When polymerization meets coordination-driven self-assembly: metallo-supramolecular polymers based on supramolecular coordination complexes, Chem. Soc. Rev., 2021, 50, 7395–7417 RSC
.
- S. Roy and C. Chakraborty, Nanostructured metallo-supramolecular polymer-based gel-type electrochromic devices with ultrafast switching time and high colouration efficiency, J. Mater. Chem. C, 2019, 7, 2871–2879 RSC
.
- Y. Liu, R. Sakamoto, C.-L. Ho, H. Nishihara and W.-Y. Wong, Electrochromic triphenylamine-based cobalt(II) complex nanosheets, J. Mater. Chem. C, 2019, 7, 9159–9166 RSC
.
- F. S. Han, M. Higuchi and D. G. Kurth, Metallosupramolecular Polyelectrolytes Self-Assembled from Various Pyridine Ring-Substituted Bisterpyridines and Metal Ions: Photophysical, Electrochemical, and Electrochromic Properties, J. Am. Chem. Soc., 2008, 130, 2073–2081 CrossRef CAS PubMed
.
- S. Mondal, T. Yoshida, U. Rana, M. K. Bera and M. Higuchi, Thermally stable electrochromic devices using Fe(II)-based metallo-supramolecular polymer, Sol. Energ. Mat. Sol. C., 2019, 200, 110000 CrossRef CAS
.
- S. Mondal, Y. Ninomiya, T. Yoshida, T. Mori, M. Bera, K. Ariga and M. Higuchi, Dual-Branched Dense Hexagonal Fe(II)-Based Coordination Nanosheets with Red-to-Colorless Electrochromism and Durable Device Fabrication, ACS Appl. Mater. Interfaces, 2020, 12, 31896–31903 CrossRef CAS PubMed
.
- S. Mondal, D. C. Santra, Y. Ninomiya, T. Yoshida and M. Higuchi, Dual-Redox System of Metallo-Supramolecular Polymers for Visible-to-Near-IR Modulable Electrochromism and Durable Device Fabrication, ACS Appl. Mater. Interfaces, 2020, 12, 58277–58286 CrossRef CAS PubMed
.
- R. Zheng, J. Zhang, C. Jia, Z. Wan, Y. Fan, X. Weng, J. Xie and L. Deng, A novel self-healing electrochromic film based on a triphenylamine cross-linked polymer, Polym. Chem., 2017, 8, 6981–6988 RSC
.
- Y. Wang, R. Zheng, J. Luo, H. A. Malik, Z. Wan, C. Jia, X. Weng, J. Xie, L. Deng and X. Yao, Self-healing dynamically cross linked versatile polymer electrolyte: A novel approach towards high performance, flexible electrochromic devices, Electrochim. Acta, 2019, 320, 134489 CrossRef CAS
.
- R. Zheng, Y. Wang, C. Jia, Z. Wan, J. Luo, H. A. Malik, X. Weng, J. Xie and L. Deng, Intelligent Biomimetic Chameleon Skin with Excellent Self-Healing and Electrochromic Properties, ACS Appl. Mater. Interfaces, 2018, 10, 35533–35538 CrossRef CAS PubMed
.
- X. Huo, R. Li, J. Wang, M. Zhang and M. Guo, Repairable electrochromic energy storage devices: A durable material with balanced performance based on titanium dioxide/tungsten trioxide nanorod array composite structure, Chem. Eng. J., 2022, 430, 132821 CrossRef CAS
.
- J. L. Wang, J. W. Liu, S. Z. Sheng, Z. He, J. Gao and S. H. Yu, Manipulating Nanowire Assemblies toward Multicolor Transparent Electrochromic Device, Nano Lett., 2021, 21, 9203–9209 CrossRef CAS PubMed
.
- K. Tang, Y. Zhang, Y. Shi, J. Cui, X. Shu, Y. Wang, J. Liu, J. Wang, H. H. Tan and Y. Wu, Preparation of V2O5 dot-decorated WO3 nanorod arrays for high performance multi-color electrochromic devices, J. Mater. Chem. C, 2018, 6, 12206–12216 RSC
.
- G. Zhao, W. Wang, X. Wang, X. Xia, C. Gu and J. Tu, A multicolor electrochromic film based on a SnO2/V2O5 core/shell structure for adaptive camouflage, J. Mater. Chem. C, 2019, 7, 5702–5709 RSC
.
- G. F. Cai, J. P. Tu, D. Zhou, J. H. Zhang, X. L. Wang and C. D. Gu, Dual electrochromic film based on WO3/polyaniline core/shell nanowire array, Sol. Energ. Mat. Sol. C., 2014, 122, 51–58 CrossRef CAS
.
- S. Sun, S. Cui, F. Wang, M. Gao, W. Wei, J. Dong, X. Xia, Q. Zhu and J. Zhang, Flexible Reflective Electrochromic Devices Based on V2O5-Methyl Cellulose Composite Films, ACS Appl. Electron. Mater., 2022, 4, 4724–4732 CrossRef CAS
.
- L. R. Savagian, A. M. Österholm, D. E. Shen, D. T. Christiansen, M. Kuepfert and J. R. Reynolds, Conjugated Polymer Blends
for High Contrast Black-to-Transmissive Electrochromism, Adv. Opt. Mater., 2018, 6, 1800594 CrossRef
.
- A. L. Dyer, E. J. Thompson and J. R. Reynolds, Completing the color palette with spray-processable polymer electrochromics, ACS Appl. Mater. Interfaces, 2011, 3, 1787–1795 CrossRef CAS PubMed
.
- R. H. Bulloch, J. A. Kerszulis, A. L. Dyer and J. R. Reynolds, An electrochromic painter's palette: color mixing via solution co-processing, ACS Appl. Mater. Interfaces, 2015, 7, 1406–1412 CrossRef CAS PubMed
.
- S. V. Vasilyeva, P. M. Beaujuge, S. Wang, J. E. Babiarz, V. W. Ballarotto and J. R. Reynolds, Material strategies for black-to-transmissive window-type polymer electrochromic devices, ACS Appl. Mater. Interfaces, 2011, 3, 1022–1032 CrossRef CAS PubMed
.
- K. Chen, Y. Wu, L. You, W. Wu, X. Wang, D. Zhang, J. F. Elman, M. Ahmed, H. Wang, K. Zhao and J. Mei, Printing dynamic color palettes and layered textures through modeling-guided stacking of electrochromic polymers, Mater. Horiz., 2022, 9, 425–432 RSC
.
- D. Dai, M. Ouyang, L. Zhang, H. Fu, B. Tao, W. Li, X. Lv, R. Bai, Y. Dong and C. Zhang, Water-soluble CMY primary color electrochromic polymers: design, synthesis and full color control, J. Mater. Chem. C, 2022, 10, 1339–1348 RSC
.
- S. Yan, H. Fu, L. Zhang, Y. Dong, W. Li, M. Ouyang and C. Zhang, Conjugated polymer multilayer by in situ electrochemical polymerization for black-to-transmissive eletrochromism, Chem. Eng. J., 2021, 406, 126819 CrossRef CAS
.
- X. Lv, J. Li, L. Zhang, M. Ouyang, A. Tameev, A. Nekrasov, G. Kim and C. Zhang, High-performance electrochromic supercapacitor based on quinacridone dye with good specific capacitance, fast switching time and robust stability, Chem. Eng. J., 2022, 431, 133733 CrossRef CAS
.
- Q. Huang, J. Chen, S. Yan, X. Shao, Y. Dong, J. Liu, W. Li and C. Zhang, New Donor-Acceptor-Donor Conjugated Polymer with Twisted Donor-Acceptor Configuration for High-Capacitance Electrochromic Supercapacitor Application, Acs Sustain. Chem. Eng., 2021, 9, 13807–13817 CrossRef CAS
.
- J. He, L. You, D. T. Tran and J. Mei, Low-Temperature Thermally Annealed Niobium Oxide Thin Films as a Minimally Color Changing Ion Storage Layer in Solution-Processed Polymer Electrochromic Devices, ACS Appl. Mater. Interfaces, 2019, 11, 4169–4177 CrossRef CAS PubMed
.
- X. Li, Z. Wang, K. Chen, D. Y. Zemlyanov, L. You and J. Mei, Stabilizing Hybrid Electrochromic Devices through Pairing Electrochromic Polymers with Minimally Color-Changing Ion-Storage Materials Having Closely Matched Electroactive Voltage Windows, ACS Appl. Mater. Interfaces, 2021, 13, 5312–5318 CrossRef CAS PubMed
.
- S. V. Vasilyeva, E. Unur, R. M. Walczak, E. P. Donoghue, A. G. Rinzler and J. R. Reynolds, Color purity in polymer electrochromic window devices on indium-tin oxide and single-walled carbon nanotube electrodes, ACS Appl. Mater. Interfaces, 2009, 1, 2288–2297 CrossRef CAS PubMed
.
- J. He, S. Mukherjee, X. Zhu, L. You, B. W. Boudouris and J. Mei, Highly Transparent Crosslinkable Radical Copolymer Thin Film as the Ion Storage Layer in Organic Electrochromic Devices, ACS Appl. Mater. Interfaces, 2018, 10, 18956–18963 CrossRef CAS PubMed
.
- K. Oyaizu, Y. Ando, H. Konishi and H. Nishide, Nernstian Adsorbate-like Bulk Layer of Organic Radical Polymers for High-Density Charge Storage Purposes, J. Am. Chem. Soc., 2008, 130, 14459–14461 CrossRef CAS PubMed
.
- N. Kobayashi, S. Miura, M. Nishimura and H. Urano, Organic electrochromism for a new color electronic paper, Sol. Energ. Mat. Sol. C., 2008, 92, 136–139 CrossRef CAS
.
- Y. Choi, K.-W. Kim, Y. R. In, X. Tang, P. Kim, V. H. V. Quy, Y. M. Kim, J. Lee, C. Choi, C. Jung, S. H. Kim, H. C. Moon and J. K. Kim, Multicolor, dual-image, printed electrochromic displays based on tandem configuration, Chem. Eng. J., 2022, 429, 132319 CrossRef CAS
.
- G. K. Pande, J. S. Heo, J. H. Choi, Y. S. Eom, J. Kim, S. K. Park and J. S. Park, RGB-to-black multicolor electrochromic devices enabled with viologen functionalized polyhedral oligomeric silsesquioxanes, Chem. Eng. J., 2021, 420, 130446 CrossRef CAS
.
- S. A. Sapp, G. A. Sotzing and J. R. Reynolds, High Contrast Ratio and Fast-Switching Dual Polymer Electrochromic Devices, Chem. Mater., 1998, 10, 2101–2108 CrossRef CAS
.
- I. Schwendeman, R. Hickman, G. Sonmez, P. Schottland, K. Zong, D. M. Welsh and J. R. Reynolds, Enhanced Contrast Dual Polymer Electrochromic Devices, Chem. Mater., 2002, 14, 3118–3122 CrossRef CAS
.
- X. Zhang, W. Li, X. Chen, Y. Zhao, L. Wang, M. Chen, Z. Li and Y. Li, Inorganic all-solid-state electrochromic devices with reversible color change between yellow-green and emerald green, Chem. Commun., 2020, 56, 10062–10065 RSC
.
- W. Zhang, H. Li, W. W. Yu and A. Y. Elezzabi, Transparent inorganic multicolour displays enabled by zinc-based electrochromic devices, Light: Sci. Appl., 2020, 9, 121 CrossRef CAS PubMed
.
- W. Zhang, H. Li and A. Y. Elezzabi, Electrochromic Displays Having Two-Dimensional CIE Color Space Tunability, Adv. Funct. Mater., 2021, 32, 2108341 CrossRef
.
- M. Wang, X. Xing, I. F. Perepichka, Y. Shi, D. Zhou, P. Wu and H. Meng, Electrochromic Smart Windows Can Achieve an Absolute Private State through Thermochromically Engineered Electrolyte, Adv. Energy Mater., 2019, 9, 1900433 CrossRef
.
- X. Xing, M. Wang and H. Meng, Color Palette Tactic for Multicolor Electrochromics Enabled by Electrolyte Thermochromic Engineering, Adv. Opt. Mater., 2022, 10, 2200941 CrossRef CAS
.
- C. Périé, V. Mary, B. Faceira and A. Rougier, Colored electrolytes for electrochromic devices, Sol. Energ. Mat. Sol. C., 2022, 238, 111626 CrossRef
.
- X. Zhang, W. Li, X. Li, L. Wang, W. Sun, H. Zhang, J. Wang, J. Zhao and Y. Li, Bio-inspired electrochromic skin based on tungsten oxide, Sol. Energ. Mat. Sol. C., 2021, 230, 111195 CrossRef CAS
.
- Z. Li, Y. Zhao, Y. Xiao, W. Sun, M. Chen, W. Li, X. Zhang, J. Zhao and Y. Li, A feasible strategy of Prussian blue reflective electrochromic devices capable of reversible switching between sand-yellow and leaf-green, Mater. Lett., 2022, 307, 130969 CrossRef CAS
.
- W. M. Kline, R. G. Lorenzini and G. A. Sotzing, A review of organic electrochromic fabric devices, Color. Technol., 2014, 130, 73–80 CAS
.
- H. Yu, M. Qi, J. Wang, Y. Yin, Y. He, H. Meng and W. Huang, A feasible strategy for the fabrication of camouflage electrochromic fabric and unconventional devices, Electrochem. Commun., 2019, 102, 31–36 CrossRef CAS
.
- K. Li, Q. Zhang, H. Wang and Y. Li, Red, green, blue (RGB) electrochromic fibers for the new smart color change fabrics, ACS Appl. Mater. Interfaces, 2014, 6, 13043–13050 CrossRef CAS PubMed
.
- H. Fan, K. Li, X. Liu, K. Xu, Y. Su, C. Hou, Q. Zhang, Y. Li and H. Wang, Continuously Processed, Long Electrochromic Fibers with Multi-Environmental Stability, ACS Appl. Mater. Interfaces, 2020, 12, 28451–28460 CrossRef CAS PubMed
.
- Y. Ding, M. A. Invernale and G. A. Sotzing, Conductivity trends of PEDOT-PSS impregnated fabric and the effect of conductivity on electrochromic textile, ACS Appl. Mater. Interfaces, 2010, 2, 1588–1593 CrossRef CAS PubMed
.
- M. A. Invernale, Y. Ding and G. A. Sotzing, All-Organic Electrochromic Spandex, ACS Appl. Mater. Interfaces, 2010, 2, 296–300 CrossRef CAS
.
- X. Chen, H. Lin, J. Deng, Y. Zhang, X. Sun, P. Chen, X. Fang, Z. Zhang, G. Guan and H. Peng, Electrochromic fiber-shaped supercapacitors, Adv. Mater., 2014, 26, 8126–8132 CrossRef CAS PubMed
.
- Y. Zhou, J. Fang, H. Wang, H. Zhou, G. Yan, Y. Zhao, L. Dai and T. Lin, Multicolor Electrochromic Fibers with Helix-Patterned Electrodes, Adv. Electron. Mater., 2018, 4, 1800104 CrossRef
.
- Y. Zhai, J. Li, S. Shen, Z. Zhu, S. Mao, X. Xiao, C. Zhu, J. Tang, X. Lu and J. Chen, Recent Advances on Dual-Band Electrochromic Materials and Devices, Adv. Funct. Mater., 2022, 32, 2109848 CrossRef CAS
.
- R. Hu, W. Xi, Y. Liu, K. Tang, J. Song, X. Luo, J. Wu and C.-W. Qiu, Thermal camouflaging metamaterials, Mater. Today, 2021, 45, 120–141 CrossRef CAS
.
- K. Sauvet, L. Sauques and A. Rougier, Electrochromic properties of WO3 as a single layer and in a full device: From the visible to the infrared, J. Phys. Chem. Solids, 2010, 71, 696–699 CrossRef CAS
.
- K. Sauvet, L. Sauques and A. Rougier, IR electrochromic WO3 thin films: From optimization to devices, Sol. Energ. Mat. Sol. C., 2009, 93, 2045–2049 CrossRef CAS
.
- X. Zhang, Y. Tian, W. Li, S. Dou, L. Wang, H. Qu, J. Zhao and Y. Li, Preparation and performances of all-solid-state variable infrared emittance devices based on amorphous and crystalline WO3 electrochromic thin films, Sol. Energ. Mat. Sol. C., 2019, 200, 109916 CrossRef CAS
.
- J. Mandal, S. Du, M. Dontigny, K. Zaghib, N. Yu and Y. Yang, Li4Ti5O12: A Visible-to-Infrared Broadband Electrochromic Material for Optical and Thermal Management, Adv. Funct. Mater., 2018, 28, 1802180 CrossRef
.
- Y. Sun, Y. Wang, C. Zhang, S. Chen, H. Chang, N. Guo, J. Liu, Y. Jia, L. Wang, Y. Weng, W. Zhao, K. Jiang and L. Xiao, Flexible Mid-Infrared Radiation Modulator with Multilayer Graphene Thin Film by Ionic Liquid Gating, ACS Appl. Mater. Interfaces, 2019, 11, 13538–13544 CrossRef CAS PubMed
.
- Y. Sun, H. Chang, J. Hu, Y. Wang, Y. Weng, C. Zhang, S. Niu, L. Cao, Z. Chen, N. Guo, J. Liu, J. Chi, G. Li and L. Xiao, Large-Scale Multifunctional Carbon Nanotube Thin Film as Effective Mid-Infrared Radiation Modulator with Long-Term Stability, Adv. Opt. Mater., 2020, 9, 2001216 CrossRef
.
- B. Wang, G. Xu, S. Song, Z. Ren, D. Liu, L. Hao, F. Xing, H. Wei, L. Zhang and Y. Li, Flexible, infrared adjustable, thermal radiation control device based on electro-emissive PANI/Ce4+ thin films inspired by chameleon, Chem. Eng. J., 2022, 445, 136819 CrossRef CAS
.
- H. Gong, J. Ai, W. Li, J. Zhu, Q. Zhang, J. Liu, Y. Jin and H. Wang, Self-Driven Infrared Electrochromic Device with Tunable Optical and Thermal Management, ACS Appl. Mater. Interfaces, 2021, 13, 50319–50328 CrossRef CAS PubMed
.
- J. S. Hale and J. A. Woollam, Prospects for IR emissivity control using electrochromic structures, Thin Solid Films, 1999, 339, 174–180 CrossRef CAS
.
- G. F. Cai, J. P. Tu, D. Zhou, X. L. Wang and C. D. Gu, Growth of vertically aligned hierarchical WO3 nano-architecture arrays on transparent conducting substrates with outstanding electrochromic performance, Sol. Energ. Mat. Sol. C., 2014, 124, 103–110 CrossRef CAS
.
- T. Ohzuku, A. Ueda and N. Yamamota, Zero-Strain Insertion Material of Li[Li1/3Ti5/3]O4 for Rechargeable Lithium Cells, J. Electrochem. Soc., 1995, 142, 1431–1435 CrossRef CAS
.
- M. Roeder, A. B. Beleke, U. Guntow, J. Buensow, A. Guerfi, U. Posset, H. Lorrmann, K. Zaghib and G. Sextl, Li4Ti5O12 and LiMn2O4 thin-film electrodes on transparent conducting oxides for all-solid-state and electrochromic applications, J. Power Sources, 2016, 301, 35–40 CrossRef CAS
.
- R. Brooke, E. Mitraka, S. Sardar, M. Sandberg, A. Sawatdee, M. Berggren, X. Crispin and M. P. Jonsson, Infrared electrochromic conducting polymer devices, J. Mater. Chem. C, 2017, 5, 5824–5830 RSC
.
- P. Topart and P. Hourquebie, Infrared switching electroemissive devices based on highly conducting polymers, Thin Solid Films, 1999, 352, 243–248 CrossRef CAS
.
- H. Li, K. Xie, Y. Pan, H. Wang and H. Wang, Study of the mechanism of the variable emissivity infrared electrochromic device based on polyaniline conducting polymer, Synthetic Met., 2012, 162, 22–25 CrossRef CAS
.
- D. Liu, G. Xu, S. Song, B. Wang, Z. Ren, L. Zhang, J. Zhao and Y. Li, Fiber-shaped dynamic thermal radiation-regulated device based on carbon fiber and polyaniline, Sol. Energ. Mat. Sol. C., 2022, 245, 111855 CrossRef CAS
.
- B. Wang, G. Xu, S. Song, Z. Ren, X. Li, J. Li, X. Xu, W. Zhou, H. Li, L. Zhang and Y. Li, Electro-emissive device based on novel PANI/Au composite films with neoteric mosaic structure for infrared stealth and thermal radiation control, Electrochim. Acta, 2021, 390, 138891 CrossRef CAS
.
|
This journal is © the Partner Organisations 2023 |
Click here to see how this site uses Cookies. View our privacy policy here.