DOI:
10.1039/D3QM00657C
(Review Article)
Mater. Chem. Front., 2023,
7, 5744-5759
Nitrides: a promising class of nonlinear optical material candidates†
Received
9th June 2023
, Accepted 9th August 2023
First published on 10th August 2023
Abstract
Nonlinear optical (NLO) materials play a crucial role in all-solid-state lasers, as their frequency conversion effects enable the expansion of the limited and fixed frequency outputs of lasers to encompass both ultraviolet and infrared regions. Nitrides have emerged as highly promising NLO candidate materials, primarily due to their potentially large second-order NLO coefficients and extensive band gaps. In recent years, nitride NLO crystals have garnered significant interest from researchers, leading to the discovery of several NLO nitrides. This review provides a comprehensive overview of both reported and potential NLO nitrides, with a particular focus on their crystal structures, in order to gain a deeper understanding of the correlations between their structure and properties. Potential NLO nitrides are analyzed using density functional theory (DFT) as a basis. Additionally, this review addresses the existing challenges and offers insights into the prospective advancements in the field of NLO nitrides, fostering further discussion and exploration.
1. Introduction
Nonlinear optical (NLO) crystals have garnered significant attention due to their crucial role in advancing laser science and technology.1–7 The conversion of laser frequency can be achieved through various up- and down-conversion processes in NLO crystals, such as second-order harmonic generation (SHG), sum frequency generation (SFG), difference frequency generation (DFG), optical parametric oscillation (OPO), and optical parametric amplification (OPA).8 Over the past few decades, several promising deep-ultraviolet (DUV), ultraviolet-visible (UV-Vis), and infrared (IR) crystals have been discovered and extensively researched, such as KBe2BO3F2 (KBBF),9 LiB3O5 (LBO),10 β-BaB2O4 (β-BBO),11 KH2PO4 (KDP),12 KTiOPO4 (KTP),13 AgGaS2(AGS),14 AgGaSe2(AGSe)15 and ZnGeP2(ZGP).16 In general, an outstanding NLO crystal should exhibit a comprehensive set of performance characteristics, including: (i) a strong second-order harmonic generation (SHG) response, (ii) a high laser damage threshold (LDT) inherently related to wide band gaps (Eg) of materials, (iii) a wide optical transparency range, (iv) appropriate birefringence (Δn) for achieving the phase-matching behavior, and (v) favourable physical and chemical properties.
Over the past decades, the exploration of inorganic NLO crystals in the DUV and UV-Vis regions has mainly focused on borates, carbonates, nitrates, phosphates and sulfates.17–22 Borates have been extensively synthesized and reported as UV and DUV NLO crystals due to their remarkable structural diversity, which combines various structural motifs such as BO33−, BO45−, B2O54−, B3O63− and B3O75− anionic groups, resulting in exceptional properties. Carbonates and nitrates have exhibited significant SHG responses and birefringence, attributed to their π-conjugated planar triangles. Phosphates and sulfates, on the other hand, possess broad band gaps and optical transparency, but their hyperpolarizabilities and optical anisotropy are weaker. As regarding the study of IR NLO materials, inorganic chalcogenides,23 halides,24 and pnictides25,26 have been widely acknowledged as promising systems for IR NLO materials. Among these, chalcogenides have received the most attention due to their comprehensive NLO properties. Generally, halides exhibit excellent IR transparency and large band gaps resulting in high laser damage threshold (LDT). However, their SHG coefficients are typically much smaller compared to chalcogenides and pnictides. In contrast, pnictides tend to have larger effective nonlinear coefficients (deff) but smaller band gaps (Eg < 2.5 eV) when compared to chalcogenides and halides.
Nitrides are considered multifunctional materials with widespread applications in various fields, such as semiconductors, catalysis, energy storage, and spintronics.27–29 In fact, nitrides hold great potential as a system for developing NLO crystals, owing to their several advantages: (i) a wide band gap and high thermal conductivity, which contribute to a high LDT in crystals; (ii) excellent thermal stability; (iii) broad transparency windows ranging from the UV to mid-IR region. Consequently, nitrides have garnered significant interest among researchers, and several nitrides have been investigated as NLO materials through experimental approaches and computational tools, such as Zn2NX (X = Cl, Br), MoZn3N4, TiZnN2 and AGeN2 (A = Sr, Ba).30–33 However, NLO nitrides with outstanding properties remain scarce, and the structure–property relationships in NLO nitrides have not been thoroughly explored. Therefore, a comprehensive study of the characteristics and structure–property correlations in NLO nitrides is urgently needed. In this work, we summarize and categorize recently reported and potential NLO nitrides into three groups based on their structural features: (i) 19 diamond-like (DL) type NLO nitrides; (ii) 19 polyhedra-stacking type NLO nitrides; and (iii) 7 other NLO nitrides with distinctive structures, the structural features of the three categories of NLO nitrides are illustrated in Fig. 1. A detailed analysis of these compounds' crystal structures, NLO properties, and structure–property correlations was provided. Furthermore, the current challenges and future directions for the development of NLO nitrides were discussed.
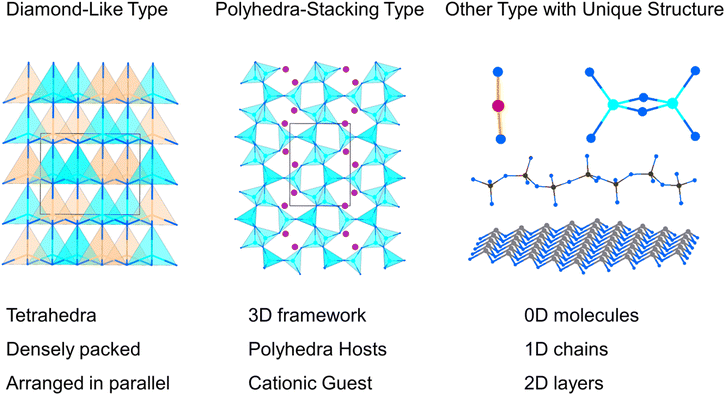 |
| Fig. 1 Schematic representation of the three categories of NLO nitrides according to structural features. | |
2. Diamond-like type NLO nitrides
Diamond-like (DL) structures represent a rich source of non-centrosymmetric (NCS) structures found in nature. These DL compounds have garnered significant interest as potential mid-IR NLO candidates due to several key advantages: (i) their intrinsic NCS crystal structures and diverse building motifs, which are formed by MQ4 (M = Si, Ge, Sn, Ga, In, Zn, Cd, Hg, Cu, Ag and Li; Q = S, Se, N and P) tetrahedra, (ii) their broad IR transparency range, which is attributed to the covalent M–Q bonds; and (iii) the fundamental building blocks of [MQ4] tetrahedra in DL structures, which are consistently interconnected through an aligned arrangement.34 This alignment is anticipated to lead to an additive superposition of the microscopic second-order susceptibility, thereby facilitating a strong SHG response. As a result, numerous DL metal chalcogenides and pnictides with exceptional NLO performance have been reported, such as Li4MgGe2S7, HgCuPS4, Li2ZnGeS4, MnSiP2, MII3PnI3 (MII = Zn and Cd, Pn = P and As) and Mg2In3Si2P7.35–40 DL nitrides, when employed as NLO materials, may offer the following benefits: (i) large NLO coefficients ascribed to the parallel alignment of [MN4] tetrahedra; (ii) wide band gaps and high thermal conductivity, which contribute to a significant LDT; and (iii) stable physical and chemical properties.
2.1. GaN and AlN
Group-III nitrides are promising materials for various technological applications, such as short-wavelength light-emitting diodes, semiconductor lasers, and optical detectors.41 The linear and nonlinear optical properties of GaN and AlN have been both theoretically and experimentally studied in the past decades.42,43 GaN and AlN crystalized in the hexagonal crystal system with space group of P63mc, and the structure of AlN was shown in Fig. 2a. The [AlN4] tetrahedra were interconnected by sharing corners and aligned along the c-axis. The experimental optical band gaps of GaN and AlN were 3.50 and 6.20 eV,44,45 respectively, and their band gaps between valence bands and conduction bands are mainly dominated by the covalent Al–N and Ga–N bands (Fig. S9a and b, ESI†). As listed in Table 1, the calculated NLO coefficients were d31 = 2.42, d33 = −3.64 pm V−1 for GaN, and d31 = 0.23, d33 = 0.86 pm V−1 for AlN, and the calculated birefringence is 0.017 for GaN and 0.023 for AlN at 1064 nm.
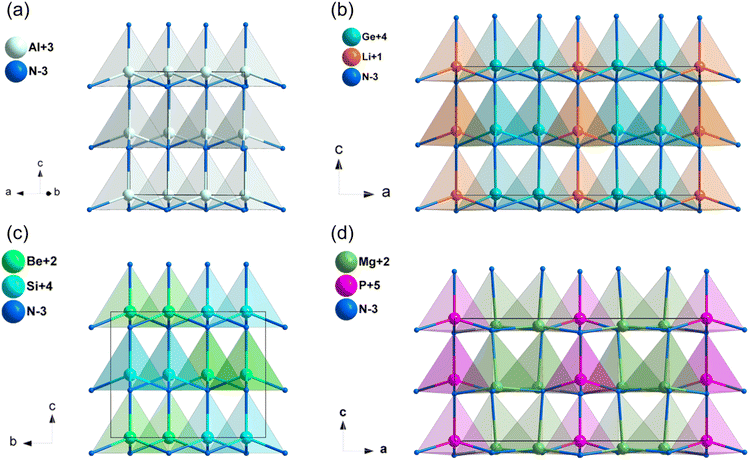 |
| Fig. 2 Crystal structure of AlN (a), LiGe2N3 (b), BeSiN2 (c) and Mg2PN3 (f). | |
Table 1 The NLO data of diamond-like type Nitrides
Compounds |
Space group |
Band gap (eV) |
NLO coefficientsa (pm V−1) |
Δna |
GGAa |
HSE06a |
Exp.b |
At 1064 nm |
At 2050 nm |
This work.
Other work from ref. 27, 41, 42, 44, 45, 48, 49, 53.
|
GaN |
P63mc |
1.93 |
2.87 |
3.5 |
d
31 = 2.42, d33 = −3.64 |
0.017 |
0.015 |
AlN |
P63mc |
3.86 |
5.12 |
6.2 |
d
31 = 0.23, d33 = 0.86 |
0.023 |
0.022 |
LiSi2N3 |
Cmc21 |
5.16 |
6.45 |
6.4 |
d
31 = 1.01, d32 = 0.24, d33 = −0.32 |
0.043 |
0.042 |
LiGe2N3 |
Cmc21 |
1.45 |
2.57 |
3.9 |
d
31 = 4.14, d32 = 5.30, d33 = −2.60 |
0.071 |
0.069 |
BeSiN2 |
Pna21 |
5.22 |
6.53 |
— |
d
31 = 0.74, d32 = 0.91, d33 = 0.45 |
0.031 |
0.030 |
MgSiN2 |
Pna21 |
4.18 |
5.49 |
4.8 |
d
31 = 0.52, d32 = 1.86, d33 = 0.32 |
0.017 |
0.017 |
MgGeN2 |
Pna21 |
2.21 |
3.35 |
3.2 |
d
31 = 6.05, d32 = 5.59, d33 = −12 |
0.032 |
0.033 |
ZnSiN2 |
Pna21 |
3.41 |
4.58 |
3.7 |
d
31 = −3.01, d32 = −6.05, d33 = 7.61 |
0.051 |
0.049 |
ZnGeN2 |
Pna21 |
2.01 |
3.01 |
3.2 |
d
31 = −4.82, d32 = −5.2, d33 = 8.13 |
0.039 |
0.038 |
Zn3MoN4 |
Pmn21 |
1.91 |
2.77 |
2.4 |
d
31 = 13.4, d32 = 14.57 |
0.098 |
0.088 |
Mg2PN3 |
Cmc21 |
3.20 |
4.43 |
5.0 |
d
31 = 1.99, d32 = 2.73, d33 = −2.33 |
0.072 |
0.071 |
Zn2PN3 |
Cmc21 |
2.83 |
4.00 |
3.7 |
d
31 = 4.43, d32 = 6.70, d33 = −9.03 |
0.003 |
0.003 |
Mg2SbN3 |
Cmc21 |
1.45 |
2.51 |
— |
d
31 = 0.26, d32 = 1.50, d33 = 3.47 |
0.031 |
0.027 |
LiPN2 |
I 2d |
3.93 |
5.21 |
— |
d
14 = d36 = 0.20 |
0.022 |
0.016 |
CuPN2 |
I 2d |
2.12 |
3.19 |
— |
d
14 = d36 = −8.26 |
0.019 |
0.018 |
Zn2NCl |
Pna21 |
2.25 |
3.31 |
3.21 |
d
31 = 5.14, d32 = 7.30, d33 = 12.89 |
0.065 |
0.062b |
Zn2NBr |
Pna21 |
2.38 |
3.09 |
2.28 |
d
31 = 4.51, d32 = 6.35, d33 = 11.15 |
0.071 |
0.069b |
LiSiON |
Pca21 |
5.48 |
7.03 |
— |
d
31 = 1.33, d32 = −2.40, d33 = −0.14 |
0.044 |
0.043 |
Li2P2ON |
Cmc21 |
5.21 |
6.82 |
— |
d
31 = 0.18, d32 = 0.33, d33 = 0.75 |
0.045 |
0.044 |
2.2. LiSi2N3 and LiGe2N3
Both LiSi2N346 and LiGe2N347 crystalized in the space group of Cmc21. Taking LiGe2N3 as an example, the [LiN4] tetrahedra and [GeN4] tetrahedra connected through sharing N atoms, the [LiN4] and [GeN4] tetrahedra in LiGe2N3 are perfectly aligned parallel to the c-axis (Fig. 2b). However, [SiN4] tetrahedra in LiSi2N3 are at an acute angle to the c-axis (Fig. S1a, ESI†). As shown in Table 1, the experimental band gaps of LiSi2N3 and LiGe2N3 are 6.40 and 3.90 eV,48 and their band gaps between valence bands and conduction bands are mainly dominated by the covalent Si–N and Ge–N bands (Fig. S9c and d, ESI†). The calculated NLO coefficients were d31 = 1.01, d32 = 0.24, d33 = −0.32 pm V−1 for LiSi2N3, and d31 = 4.14, d32 = 5.3, d33 = −2.60 pm V−1 for LiGe2N3. Additionally, their calculated birefringence is about 0.043 (LiSi2N3) and 0.071 (LiGe2N3) at 1064 nm.
2.3. A–MIV–N2 (A = Be, Mg, Zn; MIV = Si, Ge) system
BeSiN2,49 MgSiN2, MgGeN2,50 ZnSiN2 and ZnGeN251 are isostructural and their space group is Pna21, thus BeSiN2 was chosen for the analysis of their structural features. Both [BeN4] and [SiN4] tetrahedra in BeSiN2 are arranged along the same direction and connected by sharing corners (Fig. 2c). The calculated band gap of BeSiN2 is 6.53 eV (HSE06), and the experimental band gaps are 4.80, 3.20, 3.70 and 3.20 eV for MgSiN2, MgGeN2, ZnSiN2 and ZnGeN2, respectively. Their band gaps between valence bands and conduction bands are mainly dominated by the covalent A–N and MIV–N bands (Fig. S9e, f and S10a–c, ESI†). In addition, the calculated NLO coefficients and birefringence of A–MIV–N2 (A = Be, Mg, Zn; MIV = Si, Ge) system were listed in Table 1. The results show that MgGeN2 (Eg = 3.2 eV, d33 = −12 pm V−1) exhibits balanced NLO properties.
2.4. Zn3MoN4
The compound Zn3MoN452 crystallizes the space group of Pmn21 and its structure is displayed in Fig. 3a. The crystal structure of Zn3MoN4 is formed by stacking [ZnN4] and [MoN4] tetrahedra along the c-axis, and these tetrahedra are connected by sharing tetrahedral vertices. As shown in Table 1, the calculated band gap by HSE06 is 2.77 eV for Zn3MoN4, and the band gap between valence bands and conduction bands is mainly dominated by the covalent Mo–N bands (Fig. S10d, ESI†). Moreover, the calculated NLO coefficients are d31 = 13.4, d32 = 14.57 and d33 = −26.04 pm V−1, and the d33 coefficient is about 1.7 times that of AGS (15.3 pm V−1). According to the results, its calculated birefringence is about 0.098 at 1064 nm, illustrating that Zn3MoN4 could achieve phase-matching behavior in the IR NLO application.
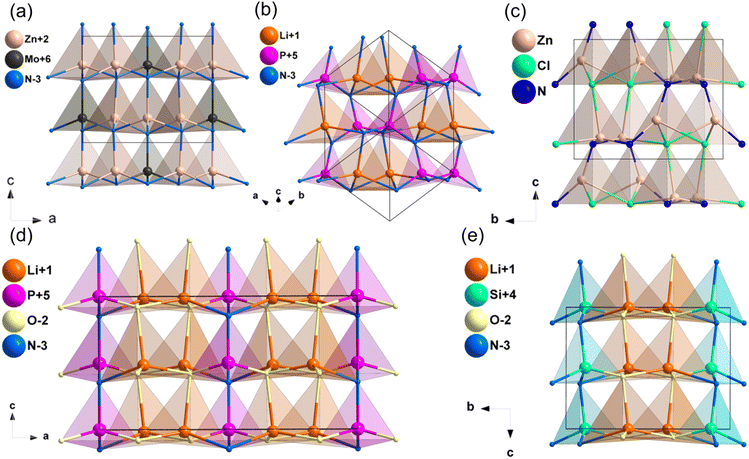 |
| Fig. 3 Crystal structure of Zn3MoN4 (a), LiPN2 (b), Zn2NCl (c), Li2PO2N (d) and LiSiON (e). | |
2.5. A–M–N (A = Li, Cu, Mg, Zn; M = P, Sb) system
Mg2PN3, Zn2PN3 and MgSbN3.
Mg2PN3,53 Zn2PN354 and MgSbN355 crystalized in the same space group Cmc21. Taking Mg2PN3 as an example, its crystal structure featured densely packed layers consisting of [MgN4] and [PN4] tetrahedra, which were all oriented towards the c-axis (Fig. 2d). The experimental band gaps were 5.0 and 3.7 eV for Mg2PN3 and Zn2PN3,56 and the calculated band gap of MgSbN3 was 2.51 eV (HSE06). The band gaps of Mg2PN3 and MgSbN3 between valence bands and conduction bands are mainly dominated by the covalent Mg–N, P–N and Sb–N bands, while the band gap of Zn2PN3 is mainly contributed by Zn–N bonds (Fig. S10e, f and S11a, ESI†). Furthermore, the calculated NLO coefficients are d31 = 1.99, d32 = 2.73, d33 = −2.33 pm V−1 for Mg2PN3, d31 = 4.43, d32 = 6.70, d33 = −9.03 pm V−1 for Zn2PN3, and d31 = 0.26, d32 = 1.50, d33 = 3.47 pm V−1 for MgSbN3, respectively. In addition, the calculated birefringence of Mg2PN3, Zn2PN3 and MgSbN3 is 0.072, 0.003 and 0.031 at 1064 nm, respectively (Table 1).
LiPN2 and CuPN2.
LiPN257 and CuPN258 are isostructural with the space group of I
2d. LiPN2 was chosen as an example to describe the structure (Fig. 3b), which was composed of [LiN4] and [PN4] tetrahedra, and they were arranged in nearly the same direction. As shown in Table 1, the HSE06 band gaps of LiPN2 and CuPN2 were 5.21 and 3.19 eV, respectively, which were larger than LiGaS2 (3.62 eV), and their band gaps between valence bands and conduction bands are mainly dominated by the Li–N, Cu–N and P–N bands (Fig. S11b and c, ESI†). The NLO coefficients of LiPN2 and CuPN2 were calculated to be d14 = d36 = 0.20 pm V−1 (LiPN2) and d14 = d36 = −8.26 pm V−1 (CuPN2), and their calculated birefringence is 0.022 and 0.019 at 1064 nm, respectively.
2.6. Diamond-like nitrides with mixed anions
Zn2NX (X = Cl, Br).
Zn2NX (X = Cl, Br) were synthesized and reported as mid-IR NLO materials in 2021 by our group.30 These two compounds are isostructural and crystallize in space group Pna21. In the structure of Zn2NCl, the distorted [ZnN2Cl2] tetrahedra are connected to each other via sharing N and Cl atoms and stacked along the same direction (Fig. 3c). The two compounds exhibit excellent mid-IR NLO performances. The experimental band gaps are 3.21 and 3.28 eV for Zn2NCl and Zn2NBr, both of which are larger than 3.00 eV. The two compounds exhibit large SHG responses which are 0.9 and 0.8 times that of AGS at 2050 nm, respectively. They also have high LDTs of 20.7 and 25.9 times that of AGS respectively. All the mentioned above mid-IR NLO properties are listed in Table 1. These results showed that Zn2NX (X = Cl, Br) are potential candidates as good IR NLO materials.
LiSiON.
LiSiON59 crystallizes in space group Pca21 and its crystal structure is displayed in Fig. 3e. The [SiN3O] and [LiNO3] tetrahedra with mixed anions were interconnected via sharing the N and O atoms, which were aligned in nearly the same direction. The calculated results of LiSiON are given in Table 1, the HSE06 band gap is 7.03 eV, which is mainly dominated by the covalent Si–O and Si–N bands (Fig. S11d, ESI†). LiSiON has three independent non-vanishing NLO tensor components (d31, d32, d33), and the calculated value are d31 = 1.33, d32 = −2.40 and d33 = −0.14 pm V−1. LiSiON exhibits a large birefringence with the calculated value of 0.044 at 1064 nm.
Li2PO2N.
The compound Li2PO2N60 crystallizes in space group Cmc21. In the structure of Li2PO2N, [PN2O2] tetrahedra are stacked parallel to the c-axis, and [LiNO3] tetrahedra are at an acute angle to the c-axis, both of which are connected by sharing N and O atoms (Fig. 3d). The HSE06 band gap of Li2PO2N is 6.82 eV, which is mainly dominated by the covalent P–O and P–N bands (Fig. S11e, ESI†). The calculated NLO coefficients are d31 = 0.18, d32 = 0.33 and d33 = 0.75 pm V−1. Notably, the computational birefringence of Li2PO2N is 0.045 at 1064 nm (Table 1).
3. Polyhedra-stacking type NLO nitrides
The design of polyhedra-stacking type compounds serves as a prevalent and effective approach for discovering exceptional NLO crystals. These compounds are characterized by a three-dimensional (3D) framework formed through the combination of [MQ4] (M = Ag, Cd, Ga, P, S,Ge, etc. Q = P, O, S and Se) with highly electropositive alkali earth elements (Li, Na, K, Rb, Cs) and alkali metals (Mg, Ca, Sr, Ba), or rare earth elements (La, Sc, Y, Lu) possessing a full shell. Polyhedra-stacking type NLO nitrides offer two primary advantages. Firstly, the structural frameworks of these compounds exhibit a wide variety, presenting opportunities for the development of innovative structures. Secondly, the structure of these compounds possesses the potential for rational adjustment, allowing for the achievement of a balance between a robust SHG response and a broad bandgap. This can be accomplished by modifying the cations to enable parallel alignment of the [MQ4] tetrahedra.
3.1. Alkali metal-based polyhedra-stacking type NLO nitrides
NaSi2N3 and NaGe2N3.
NaSi2N361 and NaGe2N362 are isostructural and their space group is Cmc21. In their structure, the adjacent [Si/GeN4] tetrahedra connected by sharing N atoms to form a layer in b–c plane, the structural framework made up by connecting layers and Na+ filled in the holes (Fig. 4). The calculated band gaps by HSE06 are 5.31 eV for NaSi2N3 and 4.17 eV for NaGe2N3, which are mainly dominated by the covalent Si/Ge–N bonds and ionic Na–N interactions (Fig. S12a and b, ESI†). The calculated NLO coefficients are d31 = 0.33, d32 = 0.73, d33 = −0.65 pm V−1 for NaSi2N3 and d31 = 4.74, d32 = 1.59, d33 = −4.36 pm V−1, respectively. In addition, their calculated birefringence is 0.064 and 0.048 at 1064 nm, respectively. All the above results are listed in Table 2.
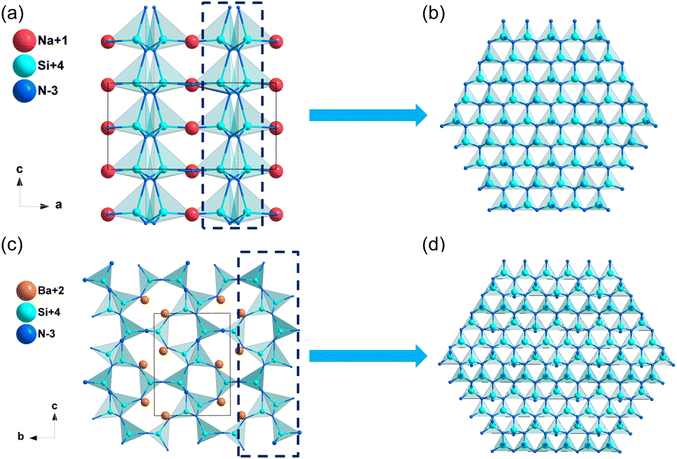 |
| Fig. 4 Crystal structure of NaSi2N3 (a) and (b) and Ba2Si5N8 (c) and (d). | |
Table 2 The NLO data of polyhedra-stacking type nitrides
Compounds |
Space group |
Band gap (eV) |
NLO coefficientsa (pm V−1) |
Δna |
GGAa |
HSE06a |
Exp.b |
At 1064 nm |
At 2050 nm |
This work.
Other work from ref. 74, 76.
|
NaSi2N3 |
Cmc21 |
4.02 |
5.31 |
— |
d
31 = 0.33, d32 = 0.73, d33 = −0.65 |
0.064 |
0.063 |
NaGe2N3 |
Cmc21 |
2.88 |
4.17 |
— |
d
31 = 4.74, d32 = 1.59, d33 = −4.36 |
0.048 |
0.047 |
NaPN2 |
I 2d |
4.77 |
6.16 |
— |
d
14 = d36 = −1.9 |
0.042 |
0.042 |
CaGeN2 |
I 2d |
3.16 |
4.31 |
— |
d
14 = d36 = −5.7 |
0.060 |
0.061 |
CaP2N4 |
P63 |
4.37 |
5.77 |
— |
d
31 = −0.02, d33 = 0.58 |
0.008 |
0.007 |
SrP2N4 |
P63 |
3.35 |
4.59 |
— |
d
31 = 0.29, d33 = 0.94 |
0.014 |
0.013 |
Sr2Si5N8 |
Pmn21 |
3.25 |
4.45 |
— |
d
31 = 1.01, d32 = 0.24, d33 = −0.32 |
0.023 |
0.023 |
Ba2Si5N8 |
Pmn21 |
2.92 |
4.03 |
— |
d
31 = 1.01, d32 = 0.24, d33 = −0.32 |
0.024 |
0.023 |
SrSi7N10 |
Pc
|
4.05 |
5.27 |
— |
d
11 = −0.22, d12 = 0.57, d13 = 3.44 |
0.018 |
0.018 |
|
|
|
|
|
d
31 = −0.49, d32 = 4.21, d33 = 0.44 |
|
|
BaSi7N10 |
Pc
|
3.93 |
5.17 |
— |
d
11 = −0.23, d12 = 0.57, d13 = −4.64 |
0.011 |
0.011 |
|
|
|
|
|
d
31 = −0.52, d32 = −3.77, d33 = 0.41 |
|
|
SrSi6N8 |
Imm2 |
3.21 |
4.46 |
— |
d
31 = −1.18, d32 = −0.30, d33 = 3.82 |
0.043 |
0.042 |
α-Ca2Si5N8 |
Cc
|
3.47 |
4.72 |
— |
d
11 = −0.23, d12 = 0.21, d13 = −0.08 |
0.009 |
0.009 |
|
|
|
|
|
d
31 = −0.10, d32 = −0.47, d33 = 0.05 |
|
|
β-Ca2Si5N8 |
P21 |
2.65 |
3.74 |
— |
d
14 = 0.35, d21 = −1.90, d22 = −0.71, d23 = −3.04 |
0.016 |
0.015 |
Ca3Al2N4 |
P212121 |
2.26 |
3.29 |
— |
d
14 = −0.28 |
0.031 |
0.029 |
SrAlSi4N7 |
Pna21 |
2.83 |
4.04 |
— |
d
31 = −1.02, d32 = 1.43, d33 = −1.34 |
0.028 |
0.027 |
SrYSi4N7 |
P63mc |
2.98 |
4.21 |
3.3–3.5 |
d
31 = −1.25, d33 = 6.45 |
0.012 |
0.011 |
CrB4O5N |
P63mc |
1.96b |
— |
— |
0.8 × SiO2b |
— |
— |
LaSi3N5 |
P212121 |
3.38 |
4.53 |
— |
d
14 = 2.50 |
0.037 |
0.036 |
Pb2Si5N8 |
Pmn21 |
2.18 |
3.17 |
— |
d
31 = 6.46, d32 = −3.71, d33 = −11.84 |
0.064 |
0.042 |
NaPN2.
NaPN263,64 crystallizes in the tetragonal space group I
2d. In this structure, [PN4] tetrahedra attached by sharing corners and expanded in the space to form the structural framework, while Na+ filled in the intervals to balance the residual charge (Fig. 5a). As listed in Table 2, the calculated band gap of NaPN2 is 6.16 eV (HSE06), which is mainly dominated by the covalent P–N bonds and ionic Na–N interactions (Fig. S11f, ESI†). The calculated NLO coefficient is d14 = d36 = −1.97 pm V−1, and the birefringence of NaPN2 was calculated to be 0.042 at 1064 nm.
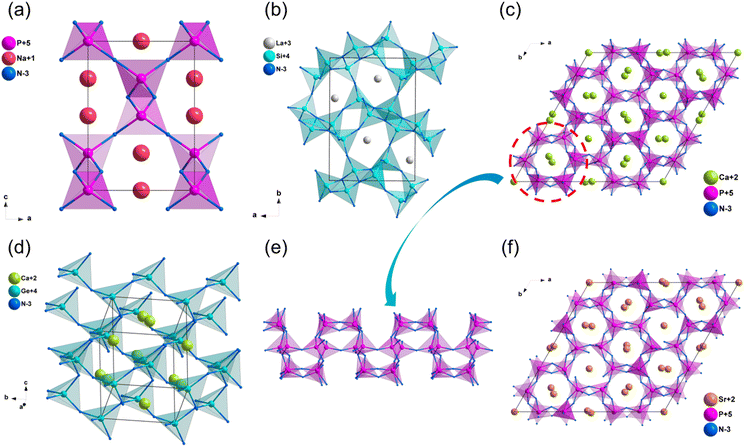 |
| Fig. 5 Crystal structure of NaPN2 (a), LaSi3N5 (b), CaP2N4 (c) and (e), SrP2N4 (f) and CaGeN2 (d). | |
3.2. Alkaline earth metals-based polyhedra-stacking type NLO nitrides
CaGeN2.
CaGeN265 crystallizes in tetragonal space group I
2d. In the structural framework of CaGeN2, [GeN4] tetrahedra interconnected by vertex-sharing and arranged along the same direction (Fig. 5d). CaGeN2 has a wider HSE06 band gap of 4.31 eV compared to ZnGeN2 (3.01 eV), which is mainly dominated by the covalent Ge–N bonds (Fig. S12c, ESI†). The calculated NLO coefficient d14 and birefringence are −5.74 pm V−1 and 0.060 at 1064 nm, respectively (Table 2).
CaP2N4 and SrP2N4.
CaP2N466 and SrP2N467 are isostructural and they crystallized in the polar space group P63. Taking CaP2N4 as an example, the [PN4] tetrahedra stacked according to the symmetry of 63 screw axis and connected via vertex-sharing, while Ca2+ are added into the holes to balance the framework (Fig. 5c, e and f). Their computational band gaps are 5.77 and 4.59 eV (HSE06), respectively, which are mainly dominated by the covalent P–N bonds (Fig. S12d and e, ESI†). The calculated NLO coefficients are d31 = −0.02, d33 = 0.58 pm V−1 for CaP2N4 and d31 = 0.29, d33 = 0.94 pm V−1 for SrP2N4 (Table 2), respectively. In addition, the calculated birefringence of CaP2N4 and SrP2N4 is 0.008 and 0.014 at 1064 nm.
Sr2Si5N8 and Ba2Si5N8.
Sr2Si5N8 and Ba2Si5N868 are isostructural and crystallized in the orthorhombic space group Pmn21. In their structure, [SiN4] tetrahedra interconnected via corners sharing to form interlayers in a–c plane, which consist of the structural framework through [SiN4] tetrahedra, and Sr2+/Ba2+ are accessed to empty positions to balance the framework (Fig. 4c and d). The optical properties of Sr2Si5N8 and Ba2Si5N8 were calculated and listed in Table 2, the calculated band gaps are 4.45 eV for Sr2Si5N8 and 4.03 eV for Ba2Si5N8 (HSE06), which are mainly dominated by the covalent Si–N bonds (Fig. S12f and S13a, ESI†). The calculated NLO coefficients are d31 = 1.7, d32 = 1.73, d33 = 0.76 pm V−1 for Sr2Si5N8 and d31 = −1.22, d32 = −0.91, d33 = −0.4 pm V−1 for Ba2Si5N8. In addition, the calculated birefringence are 0.023 (Sr2Si5N8) and 0.024 (Ba2Si5N8) at 1064 nm, respectively.
SrSi7N10 and BaSi7N10.
SrSi7N1069 and BaSi7N1070 are isostructural and their space group is Pc. In their structure, the structural framework is formed by layers (in a–c plane) and chains (parallel to c-axis) with Sr2+/Ba2+ filled in the holes, where the layers are made up of corner-shared [SiN4] tetrahedra as well as the chains constructed of corner- and prism-shared [SiN4] tetrahedra (Fig. 6a and b). The calculated band gaps of SrSi7N10 and BaSi7N10 are 5.27 and 5.17 eV, respectively, which are mainly dominated by the covalent Si–N bonds (Fig. S13b and c, ESI†). Moreover, the calculated NLO coefficients are d11 = −0.22, d12 = 0.57, d13 = 3.44, d31 = −0.49, d32 = 4.21, d33 = 0.44 pm V−1 for SrSi7N10 and d11 = −0.23, d12 = 0.57, d13 = −4.64, d31 = −0.52 pm V−1, d32 = −3.77, d33 = 0.41 pm V−1 for BaSi7N10. The calculated birefringence of SrSi7N10 and BaSi7N10 is 0.018 and 0.011 at 1064 nm, respectively.
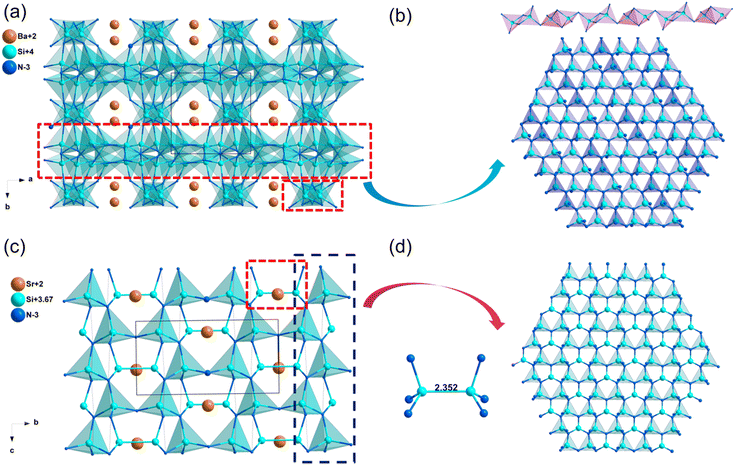 |
| Fig. 6 Crystal structure of BaSi7N10 (a) and (b) and SrSi6N8 (c) and (d). | |
SrSi6N8.
The synthesis and crystal structure of SrSi6N871 were first reported in 2005 by Schnick et al. SrSi6N8 crystallize in the orthorhombic space group Imm2. As described in Fig. 6, the structural framework is constructed by [SiN4] tetrahedra and [N3Si–SiN3] entities, which are bridged through N atoms with Sr2+ distributed in the holes. Interestingly, [N3Si–SiN3] entities contain additional Si–Si single bonds, which might enhance the SHG performance of SrSi6N8. The HSE06 band gap of SrSi6N8 is 4.46 eV, and the band gap between valence bands and conduction bands is mainly dominated by the covalent Si–N bonds (Fig. S13d, ESI†). The calculated NLO coefficient are d31 = −1.18, d32 = −0.3, d33 = 3.82 pm V−1. Notably, the computational birefringence of SrSi6N8 is 0.043 at 1064 nm (Table 2).
α-Ca2Si5N8 and β-Ca2Si5N8.
α-Ca2Si5N872,73 and β-Ca2Si5N874 are isomers, where the former crystallizes in the monoclinic space group Cc and the latter crystallizes in the monoclinic space group P21. As can be seen in Fig. 7, both α-Ca2Si5N8 and β-Ca2Si5N8 have a structural framework similar to Ba2Si5N8, including layers made up of [SiN4] tetrahedra and the ways they connected. The HSE06 band gaps are 4.72 and 3.74 eV for α-Ca2Si5N8 and β-Ca2Si5N8, respectively, which are mainly dominated by the covalent Si–N bonds (Fig. S13e and f, ESI†). The calculated NLO coefficients are d11 = −0.23, d12 = 0.21, d13 = −0.08, d31 = 0.1, d32 = −0.47, d33 = 0.05 pm V−1 for α-Ca2Si5N8 and d21 = −1.99, d22 = −0.71, d23 = −3.04, d14 = 0.35 pm V−1 for β-Ca2Si5N8. Furthermore, the calculated birefringence of α-Ca2Si5N8 and β-Ca2Si5N8 is 0.009 and 0.016 at 1064 nm.
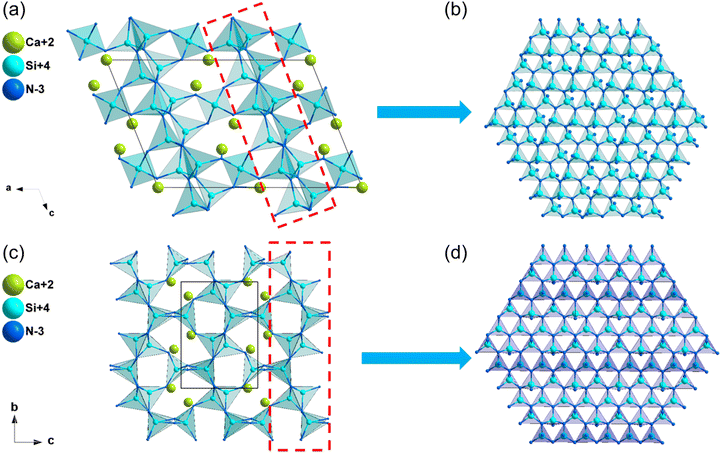 |
| Fig. 7 Crystal structure of α-Ca2Si5N8 (a) and (b) and β-Ca2Si5N8 (c) and (d). | |
Ca3Al2N4.
The compound Ca3Al2N475 crystallizes in orthorhombic space group P212121. In the structure, [AlN4] tetrahedra are connected by sharing corners and prisms to form chains, which extend into the space to constitute the framework with Ca2+ dispersed in the intervals to stabilize the structure (Fig. 8a and b). The calculated band gap of Ca3Al2N4 is 3.29 eV (HSE06), which is mainly dominated by the covalent Al–N bonds and ionic Ca–N interactions (Fig. S14a, ESI†). The calculated NLO coefficient is d14 = −0.28 pm V−1. In addition, the birefringence of Ca3Al2N4 is calculated to be 0.031 at 1064 nm. All these results were listed in Table 2.
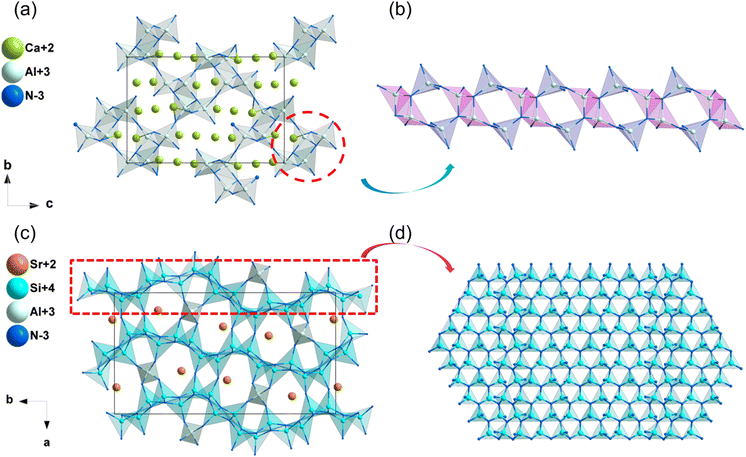 |
| Fig. 8 Crystal structure of Ca3Al2N4 (a) and (b) and SrAlSi4N7 (c) and (d). | |
SrAlSi4N7.
The compound SrAlSi4N7 was first synthesized and studied in 2009 by Schnick et al.76 SrAlSi4N7 crystallize in the polar space group Pna21, its structure featured layers consisting of [SiN4] tetrahedra, which were linked inside the layers by sharing N atoms. And the layers are attached using [AlN4] tetrahedra and N atoms as bridges to form the structural framework of SrAlSi4N7, where Sr2+ are added into the pores to balance the framework (Fig. 8c and d). The band gap calculated by HSE06 of SrAlSi4N7 is 4.04 eV, which is mainly dominated by the covalent Si–N bonds (Fig. S13b, ESI†). The calculated NLO coefficients are d31 = −1.02, d32 = 1.43, d33 = −1.37 pm V−1, respectively. In addition, the birefringence of SrAlSi4N7 is calculated to be 0.028 at 1064 nm. All the above results were listed in Table 2.
SrYSi4N7.
The synthesis and crystal structure of the compound SrYSi4N7 were reported in 2004.77 SrYSi4N7 crystallizes in the hexagonal space group P63mc and features C2-type supertetrahedra.78 The structural framework of SrYSi4N7 is assembled from layers constructed by [Si4N11] C2-type supertetrahedra, which are connected by sharing the corners of the bottom [SiP4] tetrahedra, and Sr2+ cations as well as [YN6] octahedra are filled in the pores (Fig. 9a and b). The experimental band gap of SrYSi4N7 is 3.3–3.5 eV, while the calculated band gap is 4.21 eV (HSE06), which is mainly dominated by the covalent Y–N and Si–N bonds (Fig. S14c, ESI†). Moreover, the calculated NLO coefficients of SrYSi4N7 are d31 = −1.25 and d33 = 6.45 pm V−1. The birefringence of SrYSi4N7 is calculated to be 0.012 at 1064 nm.
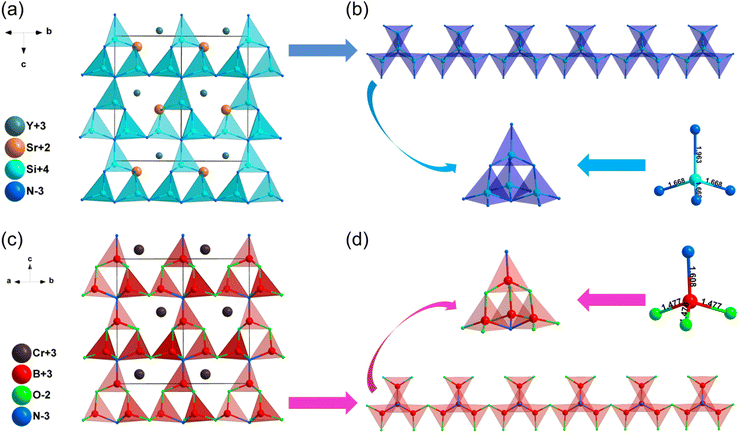 |
| Fig. 9 Crystal structure of SrYSi4N7 (a) and (b) and CrB4O5N (c) and (d). | |
3.3. Other polyhedra-stacking type NLO nitrides
CrB4O5N.
The synthesis and physical properties (including SHG performance) of CrB4O5N were investigated in 2021 by Huppertz et al.79 CrB4O5N crystallizes in hexagonal space group P63mc. Interestingly, the structure of the chromium oxonitridoborate CrB4O5N is similar to that of the nitridosilicates SrYSi4N7. The structural framework of CrB4O5N could be regarded that the [Si4N11] C2-type supertetrahedra were replaced by [B4O9N2] C2-type supertetrahedra(Fig. 9c and d). The calculated band gap of CrB4O5N is 1.96 eV (GGA), and the frontier orbitals (top of valence band and bottom of conduction band) of this compound are mainly occupied by the Cr-3d, indicating that the optical band gap is mainly decided on the Cr-3d orbital. Furthermore, the SHG response of CrB4O5N was about 0.8 times that of quartz.
LaSi3N5.
LaSi3N5 was first synthesized and studied in 1995.80 LaSi3N5 crystallizes in orthorhombic space group P212121 and its crystal structure is depicted in Fig. 5b. In this structure, the [SiN4] tetrahedra are interconnected by sharing N atoms and extend in space with La3+ added into the intervals to build the framework of LaSi3N5. The calculated results demonstrate that LaSi3N5 owns a wide band gap 4.53 eV (HSE06), which is mainly dominated by the covalent La–N bonds (Fig. S14d, ESI†). The NLO coefficient based on the DFT calculation for LaSi3N5 is d14 = 2.50 pm V−1, and LaSi3N5 exhibits large birefringence with the calculated value of 0.037 at 1064 nm.
Pb2Si5N8.
The compound Pb2Si5N881 crystallizes in orthorhombic space group Pmn21. Pb2Si5N8 is isomorphic to Sr2Si5N8 and Ba2Si5N8, and features layers consisting of [SiN4] tetrahedra. Notably, cations filled in the structural framework of Pb2Si5N8 are different from that of Sr2Si5N8 and Ba2Si5N8 (Fig. S1b, ESI†), because Pb2+ contains activated lone pair electrons, which could improve the SHG response for Pb2Si5N8 compared with Sr2Si5N8 and Ba2Si5N8.82–84 The calculated band gap of Pb2Si5N8 is 3.17 eV (HSE06), which is mainly dominated by the covalent Si–N bonds (Fig. S14e, ESI†). Its calculated NLO coefficients are d31 = 6.46, d32 = −3.71, d33 = −11.84 pm V−1, and the largest NLO tensor of Pb2Si5N8 is larger than that of Sr2Si5N8 (−1.22 pm V−1) and Ba2Si5N8 (1.73 pm V−1). Furthermore, the birefringence of Pb2Si5N8 is calculated to be 0.064 at 1064 nm.
4. Other NLO nitrides with featured structures
The structures of some nitrides exhibit low-dimensional special features, such as zero-dimensional (0D) molecules, one-dimensional (1D) chains and two-dimensional (2D) layers, and excellent properties may be realized in these nitrides with unique structures. Low-dimensional units, including [BN2] entities in Ba3B2N4, [Si2N6] dual-tetrahedra in Ba2Si2N6, [MoN4]n/[WN4]n chains in Na3MoN3/Na3WN3, [SnN3]n layers in NaSnN and [ZrN6]n layers in ZnZrN2 are discussed in detail in this section. Results indicated that these NLO nitrides with low-dimensional structures exhibited large NLO coefficients and birefringence.
NaSnN
The synthesis and physical properties (including SHG performance) of compound NaSnN were first reported in 2005 by Clarke et al,85 and the NLO properties were study by Lin et al. based on the first-principles calculations recently.86 NaSnN crystallizes in hexagonal space group P63mc, and its structural framework built by infinite two-dimensional (2D) layers with Na+ dispersed between layers, which are made up of [SnN3] triangular pyramids connecting each other through N atoms (Fig. 10a and b). In addition, the activated lone pair electrons in Sn2+ could enhance the second-order NLO response. The band gap by HSE06 of NaSnN is 1.70 eV, and the band gap between valence bands (VB) and conduction bands (CB) is dominated by the ionic Na–N coupling and covalent Sn–N hybridization. Moreover, the calculated NLO coefficients and birefringence are d13 = d23 = −2.7; d33 = 27 pm V−1 and 0.629 at 2.0 μm, respectively. All these results were listed in Table 3. The SHG-weighted density of NaSnN suggested that the [SnN3]∞ backbone with the lone electron pairs effect leading to the large NLO coefficients.
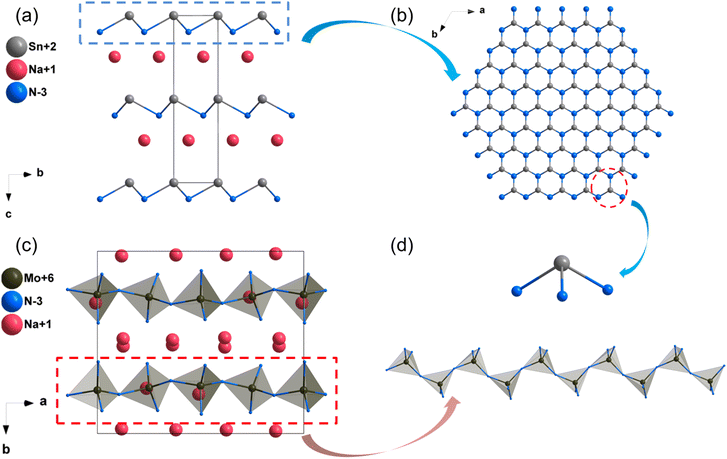 |
| Fig. 10 Crystal structure of NaSnN (a), (b) and (d) Na3MoN4 (c) and (d). | |
Table 3 The NLO data of other nitrides with featured structures
Compounds |
Space group |
Band gap (eV) |
NLO coefficientsa (pm V−1) |
Δna |
GGAa |
HSE06a |
Exp.b |
At 1064 nm |
At 2050 nm |
This work.
Other work from ref. 29, 83, 91–93.
|
NaSnN |
P63mc |
1.07 |
1.70 |
— |
d
31 = d32 = −2.7, d33 = −27 b |
0.578a |
0.629b |
Na3MoN3 |
Cc
|
1.25 |
2.10 |
— |
d
11 = 11.98, d12 = −5.98, d13 = −6.07 |
0.119 |
0.086 |
|
|
|
|
— |
d
31 = −5.74, d32 = −3.42, d33 = −1.52 |
|
|
Na3WN3 |
Cc
|
1.51 |
2.41 |
— |
d
11 = 6.21, d12 = −4.51, d13 = −3.04 |
0.079 |
0.042 |
|
|
|
|
— |
d
31 = −0.65, d32 = −3.93, d33 = −1.49 |
|
|
Ba3B2N4 |
P212121 |
2.45 |
3.41 |
— |
d
14 = d25 = d36 = −1.18 |
0.189 |
0.182 |
Ba5Si2N6 |
P212121 |
1.45 |
2.32 |
— |
d
14 = d25 = d36 = 1.88 |
0.104 |
0.096 |
ZrZnN2 |
P3m1 |
1.98b |
3.11b |
— |
d
15 = 10.06, d33 = 2.15b |
0.276b |
— |
MoSi2N4 |
— |
1.78b |
2.24b |
— |
300 × SiO2 |
— |
— |
Na3MoN3 and Na3WN3
Na3MoN387 and Na3WN388 are isostructural and crystallize in the monoclinic space group Cc. Their crystal structure featured one-dimensional (1D) infinite chains parallel to each other, constructed by vertex-sharing [MoN4] tetrahedra, and Na+ are distributed between chains to balance the charge (Fig. 10c and d). Their computational band gaps are 2.1 and 2.41 eV (HSE06), respectively. The band gaps of Na3MoN3 and Na3WN3 between valence bands and conduction bands are dominated by the ionic Na–N bands and covalent Mo/W–N bands (Fig. S14f and S15a, ESI†). The calculated NLO coefficients are d11 = 11.98, d12 = −5.98, d13 = −6.04, d31 = −5.74, d32 = −3.42, d33 = −1.52 pm V−1 for Na3MoN3 and d11 = 11.98, d12 = −5.98, d13 = −6.04, d31 = −5.74, d32 = −3.42, d33 = −1.52 pm V−1 for Na3WN3 (Table 3), respectively. In addition, the calculated birefringence of Na3MoN3 and Na3WN3 is 0.119 and 0.079 at 1064 nm.
Ba3B2N4
The compound Ba3B2N489 crystallizes in the orthorhombic space group P212121. The structural framework of Ba3B2N4 was made up of dumbbell-shaped [BN2] entities with Ba2+ cations filled in the framework. Moreover, the dumbbell-shaped [BN2] entities were almost parallel to the b-axis (Fig. 11a). According to calculated results, the HSE06 band gap of Ba3B2N4 is 3.41 eV, and the band gap between valence bands and conduction bands is mainly dominated by the ionic Ba–N bands (Fig. S15b, ESI†). The calculated NLO coefficient is d14 = −1.18 pm V−1. Furthermore, the birefringence of Ba3B2N4 is calculated to be 0.189 at 1064 nm.
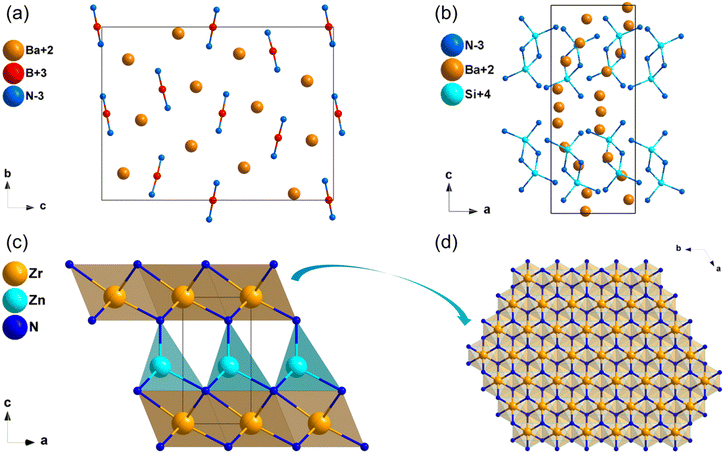 |
| Fig. 11 Crystal structure of Ba3B2N4 (a), Ba5Si2N6 (b) and ZrZnN2 (c) and (d). | |
Ba5Si2N6
The synthesis and crystal structure of compound Ba5Si2N690 was reported in 1996 by DiSalvo et al. Ba5Si2N6 crystallizes in the orthorhombic space group P212121. In this structure, the isolated prism-sharing [Si2N6] dual-tetrahedra are the backbone of the structural framework of Ba5Si2N6, and Ba2+ are dispersed in intervals between these tetrahedra to balance the charge (Fig. 11b). The calculated results implied that Ba5Si2N6 owns a wide band gap of 2.32 eV (HSE06), and the band gap between valence bands and conduction bands is mainly dominated by the ionic Ba–N bands (Fig. S15c, ESI†). The calculated NLO coefficient is d14 = 1.88 pm V−1. Moreover, the calculated birefringence of Ba5Si2N6 is 0.104 at 1064 nm.
ZrZnN2
ZrZnN2 with high thermal conductivity has been studied as an IR NLO material by Yang et al. recently.32 ZrZnN2 crystalizes in the trigonal space group P3m1, in this structure, [ZrN6] octahedra are connected by edge-sharing to build octahedral layers, which are stacked in the c direction and connected by [ZnN4] tetrahedral (Fig. 11c and d). The band gap of ZrZnN2 based on HSE06 is 3.11 eV, which is larger than that of AgGaS2 (2.73 eV). Furthermore, ZrZnN2 show balanced optical performance with the NLO coefficient of about 0.9 × AGS and the birefringence is of 0.276 at 1064 nm. Both [ZrN6] octahedra and [ZnN4] tetrahedra contributed to the NLO properties, including wide band gap and large NLO coefficients.
MoSi2N4
The growth of centimeter-scale monolayer films of MoSi2N4 was achieved in 2020 by Hong et al,91 and the NLO properties were further studied by Lin et al. based on the first-principles calculations recently.91,92 The atomic structure of MoSi2N4 was shown in Fig. 12, its structure features infinite two-dimensional (2D) N–Si–N–Mo–N–Si–N layers, which can be viewed as a MoN2 layer sandwiched between two Si–N bilayers. The experimental optical band gap of monolayer MoSi2N4 film is 1.94 eV, and the band gap between valence bands (VB) and conduction bands (CB) is dominated by Mo–N bonds and Mo4+ nonbonding states. Furthermore, the SHG response of MoSi2N4 was about 300 times that of quartz, and the SHG response of MoSi2N4 mainly comes from the inner Mo–N bands of MoN2 layers.
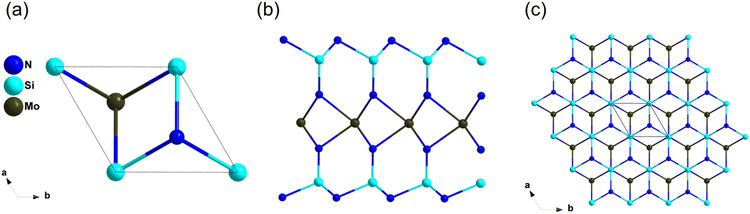 |
| Fig. 12 The atomic structure of MoSi2N4. | |
Conclusions and outlooks
In this review, we systematically summarize the potential and recent advancements in nitride-based NLO crystals, which include 45 nitrides. These crystals are classified into three categories based on their structural features: diamond-like type NLO nitrides, polyhedra-stacking type NLO nitrides, and other NLO nitrides with unique structures. We consistently discuss their crystal structures, physical properties, and structure–property relationships. Our findings indicate that nitrides possess wide band gaps and transparency windows, ranging from the ultraviolet to the infrared region. Notably, several NLO nitrides exhibit outstanding NLO properties including BeSiN2, LiSi2N3, LiSiON, LiPO2N, Zn3MoN4, Zn2NX (X = Cl, Br), NaPN2, Pb2Si5N8, NaSnN, Na3MoN4 and ZrZnN2. Furthermore, approximately 40% of these NLO nitrides contain elemental silicon, suggesting that Si-based nitrides warrant further exploration as NLO materials. However, the investigation of NLO nitrides is far from complete and faces numerous challenges, such as difficult synthesis conditions and unclear property influencing mechanisms. Consequently, the following aspects should be considered for the further application and development of NLO nitrides:
1. The development of new synthetic methods and advanced equipment technology is necessary to obtain more NLO nitrides with exceptional properties. Currently, most non-centrosymmetric nitrides are obtained using traditional solid-state synthesis technology under high temperature and pressure conditions. Advanced equipment technology is crucial for meeting these demanding conditions. Additionally, the ammonothermal method and salt-flux method may be effective ways to synthesize more NLO nitrides. Moreover, since high-performance NLO nitrides are predominantly available in powder or micron-sized crystal forms, further research on large-size crystal growth is needed.
2. A deeper understanding of the chemical bonding mechanisms between nitrides and pnictides is essential. Nitrogen and phosphorus belong to the same main group VA, while homo-cationic and homo-anionic bonds often form in pnictide crystal structures, they do not in nitrides. These homoatomic bonds significantly impact linear optical and NLO properties. Therefore, studying the bonding habits of nitrides and pnictides is crucial for designing excellent NLO materials.
3. Prioritizing the exploration of mixed-anion inorganic nitrides as NLO materials is recommended. Combining different anions into one structure can effectively enhance NLO properties. Consequently, halogens (Cl, Br, I) and phosphorus (P) can be introduced into nitrides to form [MNyX4−y] (M = mental elements, X = Cl, Br, I, P) mixed anionic tetrahedra. These distorted tetrahedra possess large anisotropic polarizability and strong hyperpolarizability, which can improve the SHG response and birefringence.
4. Developing metal nitrides with triangular anionic groups MN3 (M = Sb, Te, Sn) is suggested. MN3 groups possess stereochemically active lone-pair electrons, and compounds containing these electrons exhibit significant SHG response and birefringence, as seen in NaSnN. Therefore, more in-depth investigations should be conducted to design NLO nitrides with triangular anionic groups.
Author contributions
Xin Zhao performed the theoretical calculation, data analysis, and paper writing; Chensheng Lin and Haotian Tian offered help in theoretical calculation; Chao Wang offered help in analyzing data; Ning Ye and Min Luo guided and revised the manuscript. All authors contributed to the general discussion.
Conflicts of interest
There are no conflicts to declare. The authors declare no competing financial interests.
Acknowledgements
This work was supported by the National Natural Science Foundation of China (22222510, 21975255 and 21921001), Natural Science Foundation of Fujian Province (2023J02026), the Foundation of Fujian Science & Technology Innovation Laboratory (2021ZR202), Youth Innovation Promotion Association CAS (2019303).
References
- Z. H. Yang and S. L. Pan, Computationally assisted multistage design and prediction driving the discovery of deep-ultraviolet nonlinear optical materials, Mater. Chem. Front., 2021, 5, 3507–3523 RSC.
- S. Cho and K. M. Ok, LiRE(SO4)2 (RE = Y, Gd, Eu): noncentrosymmetric chiral rare-earth sulfates with very large band gaps, Mater. Chem. Front., 2022, 7, 65–71 RSC.
- L. Kang, F. Liang, X. X. Jiang, Z. S. Lin and C. T. Chen, First-Principles Design and Simulations Promote the Development of Nonlinear Optical Crystals, Acc. Chem. Res., 2020, 53, 209–217 CrossRef CAS PubMed.
- F. Xu, G. Zhang, M. Luo, G. Peng, Y. Chen, T. Yan and N. Ye, A Powder Method for The High-efficacy Evaluation of Electro-optic Crystals, Natl. Sci. Rev., 2020, 8, nwaa104 CrossRef PubMed.
- X. M. Liu, L. Kang, P. F. Gong and Z. S. Lin, LiZn(OH)CO3: A Deep-Ultraviolet Nonlinear Optical Hydroxycarbonate Designed from a Diamond-like Structure, Angew. Chem., Int. Ed., 2021, 60, 13574–13578 CrossRef CAS PubMed.
- C. Wu, C. B. Jiang, G. F. Wei, X. X. Jiang, Z. J. Wang, Z. S. Lin, Z. P. Huang, M. G. Humphrey and C. Zhang, Toward Large Second-Harmonic Generation and Deep-UV Transparency in Strongly Electropositive Transition Metal Sulfates, J. Am. Chem. Soc., 2023, 145, 3040–3046 CrossRef CAS PubMed.
- L. Wu, H. Fan, C. Lin and M. Luo, Compounds consisting of coplanar π-conjugated B3O6 – typed structures: An emerging source of ultraviolet nonlinear optical materials, Chin. J. Struct. Chem., 2023, 42, 100019 CrossRef.
- F. J. Duarte, Tunable laser optics: Applications to optics and quantum optics, Prog. Quantum Electron., 2013, 37, 326–347 CrossRef.
- C. T. Chen, G. L. Wang, X. Y. Wang and Z. Y. Xu, Deep-UV nonlinear optical crystal KBe2BO3F2 – discovery, growth, optical properties and applications, Appl. Phys. B: Lasers Opt., 2009, 97, 9–25 CrossRef CAS.
- C. T. Chen, Y. C. Wu, A. D. Jiang, B. C. Wu, G. M. You, R. K. Li and S. J. Lin, New Nonlinear-optical crystal – LiB3O5, J. Opt. Soc. Am. B, 1989, 6, 616–621 CrossRef CAS.
-
D. N. Nikogosyan, Nonlinear Optical Crystals: a Complete Survey, Springer-Science and Business Media, New York, 2005 Search PubMed.
- W. L. Smith, KDP and ADP transmission in vacuum ultraviolet, Appl. Optics, 1977, 16, 1798 CrossRef CAS PubMed.
- K. Kato, Parametric oscillation at 3.2 μm in KTP pumped at 1.064 μm, IEEE J. Quantum Electron., 1991, 27, 1137–1140 CrossRef CAS.
- A. O. Okorogu, S. B. Mirov, W. Lee, D. I. Crouthamel, N. Jenkins, A. Y. Dergachev, K. L. Vodopyanov and V. V. Badikov, Tunable middle infrared down-conversion in GaSe and AgGaS2, Opt. Commun., 1998, 155, 307–312 CrossRef CAS.
- G. D. Boyd, F. G. Storz, J. H. McFee and H. M. Kasper, Linear and Nonlinear Optical Properties of Some Ternary Selenides, IEEE J. Quantum Electron., 1972, 8, 900–908 CrossRef CAS.
- G. D. Boyd, E. Buehler and F. G. Storz, Linear and Nonlinear Optical Properties of ZnGeP2 and CdSe, Appl. Phys. Lett., 1971, 18, 301–304 CrossRef CAS.
- M. Mutailipu and S. L. Pan, Emergent Deep-Ultraviolet Nonlinear Optical Candidates, Angew. Chem., Int. Ed., 2020, 59, 20302–20317 CrossRef CAS PubMed.
- T. T. Tran, H. W. Yu, J. M. Rondinelli, K. R. Poeppelmeier and P. S. Halasyamani, Deep Ultraviolet Nonlinear Optical Materials, Chem. Mater., 2016, 28, 5238–5258 CrossRef CAS.
- W. Q. Huang, S. G. Zhao and J. H. Luo, Recent Development of Non-pi-Conjugated Deep Ultraviolet Nonlinear Optical Materials, Chem. Mater., 2022, 34, 5–28 CrossRef CAS.
- P. F. Gong, X. M. Liu, L. Kang and Z. S. Lin, Inorganic planar pi-conjugated groups in nonlinear optical crystals: review and outlook, Inorg. Chem. Front., 2020, 7, 839–852 RSC.
- H. Su, Z. Yan, X. Hou and M. Zhang, Fluorooxoborates: A precious treasure of deep-ultraviolet nonlinear optical materials, Chin. J. Struct. Chem., 2023, 42, 100027 CrossRef.
- B. Zhang and Z. Chen, Recent advances of inorganic phosphates with UV/DUV cutoff edge and large second harmonic response, Chin. J. Struct. Chem., 2023, 42, 100033 CrossRef.
- L. Gao, J. Huang, S. Guo, Z. Yang and S. Pan, Structure-property survey and computer-assisted screening of mid-infrared nonlinear optical chalcohalides, Coord. Chem. Rev., 2020, 421, 213379 CrossRef CAS.
- P. Gong, F. Liang, L. Kang, X. Chen, J. Qin, Y. Wu and Z. Lin, Recent advances and future perspectives on infrared nonlinear optical metal halides, Coord. Chem. Rev., 2019, 380, 83–102 CrossRef CAS.
- H. D. Yang, M. Y. Ran, W. B. Wei, X. T. Wu, H. Lin and Q. L. Zhu, The Rise of Infrared Nonlinear Optical Pnictides: Advances and Outlooks, Chem. – Asian J., 2021, 16, 3299–3310 CrossRef CAS PubMed.
- J. Chen, X. Jiang, Q. Wu, Z. Lin, M. Luo and N. Ye, Pnictides: An emerging class of infrared nonlinear optical material candidates, J. Alloys Compd., 2022, 901, 163384 CrossRef CAS.
- W. H. Sun, C. J. Bartel, E. Arca, S. R. Bauers, B. Matthews, B. Orvananos, B. R. Chen, M. F. Toney, L. T. Schelhas, W. Tumas, J. Tate, A. Zakutayev, S. Lany, A. M. Holder and G. Ceder, A map of the inorganic ternary metal nitrides, Nat. Mater., 2019, 18, 732–739 CrossRef CAS PubMed.
- S. Yourdkhani, T. Korona and N. L. Hadipour, Structure and Energetics of Complexes of B12N12 with Hydrogen Halides—SAPT (DFT) and MP2 Study, J. Phys. Chem. A, 2015, 119, 6446–6467 CrossRef CAS PubMed.
- G. Seifert, P. W. Fowler, D. Mitchell, D. Porezag and T. Frauenheim, Boron-nitrogen analogues of the fullerenes: Electronic and structural properties, Chem. Phys. Lett., 1997, 268, 352–358 CrossRef CAS.
-
X. Zhao, C. Lin, J. Chen, M. Luo, F. Xu, S. Yang, S. Shi, B. Li and N. Ye, Halonitrides Zn2NX (X = Cl, Br): Novel Mid-Infrared Nonlinear Optical Materials, Chem. Mater., 2021, 33, 1462–1470 Search PubMed.
- J. Huang, S. Shu and G.-M. Cai, Screening Nitrides with High Debye Temperatures as Nonlinear Optical Materials, J. Phys. Chem. C, 2022, 126, 7047–7053 CrossRef CAS.
- D. Chu, Y. Huang, C. Xie, E. Tikhonov, I. Kruglov, G. Li, S. Pan and Z. Yang, Unbiased Screening of Novel Infrared Nonlinear Optical Materials with High Thermal Conductivity: Long-neglected Nitrides and Popular Chalcogenides, Angew. Chem., Int. Ed., 2023, 62, e202300581 CrossRef CAS PubMed.
- J. B. Huang, C. W. Xie, L. Wei, Q. Bian, Z. H. Yang and S. Pan, Predicting Diamond-like Nitrides as Infrared Nonlinear Optical Materials with High Thermal Conductivity, Chem. Mater., 2022, 34, 10059–10067 CrossRef CAS.
- F. Liang, L. Kang, Z. Lin, Y. Wu and C. Chen, Analysis and prediction of mid-IR nonlinear optical metal sulfides with diamond-like structures, Coord. Chem. Rev., 2017, 333, 57–70 CrossRef CAS.
- A. Abudurusuli, J. B. Huang, P. Wang, Z. H. Yang, S. L. Pan and J. J. Li, Li4MgGe2S7: The First Alkali and Alkaline-Earth Diamond-Like Infrared Nonlinear Optical Material with Exceptional Large Band Gap, Angew. Chem., Int. Ed., 2021, 60, 24131–24136 CrossRef CAS PubMed.
- M. Y. Li, Z. J. Ma, B. X. Li, X. T. Wu, H. Lin and Q. L. Zhu, HgCuPS4: An Exceptional Infrared Nonlinear Optical Material with Defect Diamond-like Structure, Chem. Mater., 2020, 32, 4331–4339 CrossRef CAS.
- Y. Huang, K. Wu, J. N. Cheng, Y. Chu, Z. H. Yang and S. L. Pan, Li2ZnGeS4: a promising diamond-like infrared nonlinear optical material with high laser damage threshold and outstanding second-harmonic generation response, Dalton Trans., 2019, 48, 4484–4488 RSC.
- T. T. Yu, S. P. Wang, X. Zhang, C. N. Li, J. Qiao, N. Jia, B. Han, S. Q. Xia and X. T. Tao, MnSiP2: A New Mid-IR Ternary Phosphide with Strong SHG Effect and Ultrabroad Transparency Range, Chem. Mater., 2019, 31, 2010–2018 CrossRef CAS.
- J. Chen, C. Lin, D. Zhao, M. Luo, G. Peng, B. Li, S. Yang, Y. Sun and N. Ye, Anionic Aliovalent Substitution from Structure Models of ZnS: Novel Defect Diamond-like Halopnictide Infrared Nonlinear Optical Materials with Wide Band Gaps and Large SHG Effects, Angew. Chem., Int. Ed., 2020, 59, 23549–23553 CrossRef CAS PubMed.
- J. Chen, H. Chen, F. Xu, L. Cao, X. Jiang, S. Yang, Y. Sun, X. Zhao, C. Lin and N. Ye, Mg2In3Si2P7: A Quaternary Diamond-like Phosphide Infrared Nonlinear Optical Material Derived from ZnGeP2, J. Am. Chem. Soc., 2021, 143, 10309–10316 CrossRef CAS PubMed.
-
R. A. Ferreyra, C. Zhu, A. Teke and H. Morkoç, in Springer Handbook of Electronic and Photonic Materials, ed. S. Kasap and P. Capper, Springer International Publishing, Cham, Switzerland, 2nd edn, 2017, Part D, 743–801 Search PubMed.
- V. I. Gavrilenko and R. Q. Wu, Linear and nonlinear optical properties of group-III nitrides, Phys. Rev. B: Condens. Matter Mater. Phys., 2000, 61, 2632–2642 CrossRef CAS.
- J. Chen, Z. H. Levine and J. W. Wilkins, Calculated second harmonic susceptibilities of BN, AlN, and GaN, Appl. Phys. Lett., 1995, 66, 1129–1131 CrossRef CAS.
- P. B. Perry and R. F. Rutz, The optical absorption edge of single crystal AlN prepared by a close spaced vapor process, Appl. Phys. Lett., 1978, 33, 319–321 CrossRef CAS.
- B. Monemar, Fundamental energy gap of GaN from photoluminescence excitation spectra, Phys. Rev. B: Condens. Matter Mater. Phys., 1974, 10, 676–681 CrossRef CAS.
- M. Orth and W. Schnick, On LiSi2N3 – Synthesis and crystal structure refinement, Z. Anorg. Allg. Chem., 1999, 625, 1426–1428 CrossRef CAS.
- J. Hausler, R. Niklaus, J. Minar and W. Schnick, Ammonothermal Synthesis and Optical Properties of Ternary Nitride Semiconductors Mg-IV-N2, Mn-IV-N2 and Li-IV2-N3 (IV = Si, Ge), Chem. – Eur. J., 2018, 24, 1686–1693 CrossRef PubMed.
- Y. Q. Li, N. Hirosaki, R. J. Xie, T. Takeka and M. Mitomo, Crystal, electronic structures and photoluminescence properties of rare-earth doped LiSi2N3, J. Solid State Chem., 2009, 182, 301–311 CrossRef CAS.
- P. Eckerlin, Zur Kenntnis des Systems Be3N2–Si3N4.4. Die Kristallstruktur von BeSiN2, Z. Anorg. Allg. Chem., 1967, 353, 225–236 CrossRef CAS.
- J. David, Y. Laurent and J. Lang, Structure of MgSiN2 and Mg GeN2, Bull. Mineral., 1970, 93, 153–159 CAS.
- J. Hausler, S. Schimmel, P. Wellmann and W. Schnick, Ammonothermal Synthesis of Earth-Abundant Nitride Semiconductors ZnSiN2 and ZnGeN2 and Dissolution Monitoring by In Situ X-ray Imaging, Chem. – Eur. J., 2017, 23, 12275–12282 CrossRef PubMed.
- E. Arca, S. Lany, J. D. Perkins, C. Bartel, J. Mangum, W. H. Sun, A. Holder, G. Ceder, B. Gorman, G. Teeter, W. Tumas and A. Zakutayev, Redox-Mediated Stabilization in Zinc Molybdenum Nitrides, J. Am. Chem. Soc., 2018, 140, 4293–4301 CrossRef CAS PubMed.
- V. SchultzCoulon and W. Schnick, Mg2PN3 and Ca2PN3 – Phosphorus(V) nitrides with infinite chains of corner sharing PN4 tetrahedra, Z. Anorg. Allg. Chem., 1997, 623, 69–74 CrossRef CAS.
- S. J. Sedlmaier, M. Eberspacher and W. Schnick, High-Pressure Synthesis, Crystal Structure, and Characterization of Zn2PN3 – A New catena-Polynitridophosphate, Z. Anorg. Allg. Chem., 2011, 637, 362–367 CrossRef CAS.
- K. N. Heinselman, S. Lany, J. D. Perkins, K. R. Talley and A. Zakutayev, Thin Film Synthesis of Semiconductors in the Mg–Sb–N Materials System, Chem. Mater., 2019, 31, 8717–8724 CrossRef CAS.
- M. Mallmann, C. Maak, R. Niklaus and W. Schnick, Ammonothermal Synthesis, Optical Properties, and DFT Calculations of Mg2PN3 and Zn2PN3, Chem. – Eur. J., 2018, 24, 13963–13970 CrossRef CAS PubMed.
- R. Marchand, P. Lharidon and Y. Laurent, Crystallochemical Study of LiPN2 – A Structure Derived from Cristobalite, J. Solid State Chem., 1982, 43, 126–130 CrossRef CAS.
- F. J. Pucher, F. Hummel and W. Schnick, CuPN2: Synthesis, Crystal Structure, and Electronic Properties, Eur. J. Inorg. Chem., 2015, 1886–1891 CrossRef CAS.
- Y. Laurent, J. Guyader and G. Roult, Time-of-flight neutron diffraction study of α-LiSiON, Acta Crystallogr., Sect. B: Struct. Sci., 1981, 37, 911–913 CrossRef.
- K. Senevirathne, C. S. Day, M. D. Gross, A. Lachgar and N. A. W. Holzwarth, A new crystalline LiPON electrolyte: Synthesis, properties, and electronic structure, Solid State Ion., 2013, 233, 95–101 CrossRef CAS.
- H. Jacobs and H. Mengis, Preparation and Crystal-Structure of a Sodium SiliconI-nitride, NaSi2N3, Eur. J. Solid State Inorg. Chem., 1993, 30, 45–53 CAS.
- J. Guyader, P. L. Haridon, Y. Laurent, R. Jacquet and G. Roult, A new nitrogen tetrahedron NaN4: Preparation and structure of NaGe2N3, J. Solid State Chem., 1984, 54, 251–255 CrossRef CAS.
- K. Landskron, S. Schmid and W. Schnick, High-pressure synthesis, crystal structure, and properties of NaPN2, Z. Anorg. Allg. Chem., 2001, 627, 2469–2472 CrossRef CAS.
- Z. Li, A. Tudi, P. Ren, Y. Yang, T. Iitaka, T. Tohyama, Z. Yang, S. Pan and H. Su, NaPN2: Deep-ultraviolet nonlinear optical material with unprecedented strong second-harmonic generation coefficient, Phys. Rev. Mater., 2019, 3, 025201 CrossRef CAS.
- Y. Laurent, M. Maunaye, J. Guyader and J. Lang, Étude structurale de CaGeN2 et Ca1−xGeN2, Bull. Mineral., 1971, 347–352 Search PubMed.
- F. J. Pucher, A. Marchuk, P. J. Schmidt, D. Wiechert and W. Schnick, Luminescent Nitridophosphates CaP2N4:Eu2+, SrP2N4:Eu2+, BaP2N4:Eu2+, and BaSr2P6N12:Eu2+, Chem. – Eur. J., 2015, 21, 6443–6448 CrossRef CAS PubMed.
- F. W. Karau, L. Seyfarth, O. Oeckler, J. Senker, K. Landskron and W. Schnick, The stuffed framework structure of SrP2N4: Challenges to synthesis and crystal structure determination, Chem. – Eur. J., 2007, 13, 6841–6852 CrossRef CAS PubMed.
- T. Schlieper, W. Milius and W. Schnick, Nitridosilicates 2. High-temperature Syntheses and Crystal-structure of Sr2Si5N8 and Ba2Si5N8, Z. Anorg. Allg. Chem., 1995, 621, 1380–1384 CrossRef CAS.
- G. Pilet, H. A. Hoppe, W. Schnick and S. Esmaeilzadeh, Crystal structure and mechanical properties of SrSi7N10, Solid-State Sci., 2005, 7, 391–396 CrossRef CAS.
- H. Huppertz and W. Schnick, Edge-sharing SiN4 tetrahedra in the highly condensed nitridosilicate BaSi7N10, Chem. – Eur. J., 1997, 3, 249–252 CrossRef CAS PubMed.
- F. Stadler, O. Oeckler, J. Senker, H. A. Hoppe, P. Kroll and W. Schnick, SrSi6N8 – A reduced nitridosilicate with a Si–Si bond, Angew. Chem., Int. Ed., 2005, 44, 567–570 CrossRef CAS PubMed.
- T. Schlieper and W. Schnick, Nitridosilicates 1. High-temperature Syntheses and Crystal-structure of Ca2Si5N8, Z. Anorg. Allg. Chem., 1995, 621, 1037–1041 CrossRef CAS.
- P. Bielec, O. Janka, T. Block, R. Pottgen and W. Schnick, Fe2Si5N8: Access to Open-Shell Transition-Metal Nitridosilicates, Angew. Chem., Int. Ed., 2018, 57, 2409–2412 CrossRef CAS PubMed.
- P. Bielec and W. Schnick, Increased Synthetic Control-Gaining Access to Predicted Mg2Si5N8 and β-Ca2Si5N8, Angew. Chem., Int. Ed., 2017, 56, 4810–4813 CrossRef CAS PubMed.
- M. Ludwig, J. Jager, R. Niewa and R. Kniep, Crystal structures of two polymorphs of Ca3Al2N4, Inorg. Chem., 2000, 39, 5909–5911 CrossRef CAS PubMed.
- C. Hecht, F. Stadler, P. J. Schmidt, J. S. A. der Guenne, V. Baumann and W. Schnick, SrAlSi4N7:Eu2+ – A Nitridoalumosilicate Phosphor for Warm White Light (pc)LEDs with Edge-Sharing Tetrahedra, Chem. Mater., 2009, 21, 1595–1601 CrossRef CAS.
- Y. Q. Li, C. M. Fang, G. de With and H. T. Hintzen, Preparation, structure and photoluminescence properties of Eu2+ and Ce3+-doped SrYSi4N7, J. Solid State Chem., 2004, 177, 4687–4694 CrossRef CAS.
- Z. Qian, Q. Bian, H. P. Wu, H. W. Yu, Z. S. Lin, Z. G. Hu, J. Y. Wang and Y. C. Wu, β-BaGa4Se7: a promising IR nonlinear optical crystal designed by predictable structural rearrangement, J. Mater. Chem. C, 2021, 10, 96–101 RSC.
- B. Fuchs, D. Johrendt, L. Bayarjargal and H. Huppertz, The First High-Pressure Chromium Oxonitridoborate CrB4O6N-an Unexpected Link to Nitridosilicate-Chemistry, Angew. Chem., Int. Ed., 2021, 60, 21801–21806 CrossRef CAS PubMed.
- M. Woike and W. Jeitschko, Preparation and Crystal-structure of the Nitridosilicates Ln3Si6N11(Ln = La, Ce, Pr, Nd, Sm) and LnSi3N5 (Ln = Ce, Pr, Nd), Inorg. Chem., 1995, 34, 5105–5108 CrossRef CAS.
- P. Bielec, R. Nelson, R. P. Stoffel, L. Eisenburger, D. Gunther, A. K. Henss, J. P. Wright, O. Oeckler, R. Dronskowski and W. Schnick, Cationic Pb2 Dumbbells Stabilized in the Highly Covalent Lead Nitridosilicate Pb2Si5N8, Angew. Chem., Int. Ed., 2019, 58, 1432–1436 CrossRef CAS PubMed.
- W. F. Chen, B. W. Liu, S. M. Pei, X. M. Jiang and G. C. Guo, K2PbX Ga7S12 (X = Cl, Br, I): The First Lead-Containing Cationic Moieties with Ultrahigh Second-Harmonic Generation and Band Gaps Exceeding the Criterion of 2.33 eV, Adv. Sci., 2023, 10, 2207630 CrossRef CAS PubMed.
- X. L. Chen, Q. Jing and K. M. Ok, Pb18O8Cl15I5: A Polar Lead Mixed Oxyhalide with Unprecedented Architecture and Excellent Infrared Nonlinear Optical Properties, Angew. Chem., Int. Ed., 2020, 59, 20323–20327 CrossRef CAS PubMed.
- M. Abudoureheman, S. J. Han, B. H. Lei, Z. H. Yang, X. F. Long and S. L. Pan, KPb2(PO3)5: a novel nonlinear optical lead polyphosphate with a short deep-UV cutoff edge, J. Mater. Chem. C, 2016, 4, 10630–10637 RSC.
- N. S. P. Watney, Z. A. Gal, M. D. S. Webster and S. J. Clarke, The first ternary tin(II) nitride: NaSnN, Chem. Commun., 2005, 4190–4192 RSC.
- S. Zhang, L. Kang and Z. Lin, Nonlinear optical ASnX (A = Na, H; X = N, P) nanosheets with divalent tin lone electron pair effect by first-principles design, Nanoscale, 2020, 12, 14895–14902 RSC.
- D. Ostermann, U. Zachwieja and H. Jacobs, Sodium nitride metallates, Na3MN3, of molybd/in (VI) and Tungsten(VI) with [CrO202/2]-isosteric [MoN2N2/2]3 chains, J. Alloys Compd., 1992, 190, 137–140 CrossRef CAS.
- H. Jacobs and R. Niewa, Synthesis and crystal-structure- of a Sodium Nitrido tungstate(VI), Na3WN3, Eur. J. Solid State Inorg. Chem., 1994, 31, 105–113 CAS.
- O. Reckeweg, F. J. DiSalvo and M. Somer, Orthorhombic Ba3(BN2)2: a new structure type for an alkaline earth metal nitrido borate, J. Alloys Compd., 2003, 361, 102–107 CrossRef CAS.
- H. Yamane and F. J. DiSalvo, Preparation and crystal structure of a new barium silicon nitride, Ba5Si2N6, J. Alloys Compd., 1996, 240, 33–36 CrossRef CAS.
- Y. L. Hong, Z. B. Liu, L. Wang, T. Y. Zhou, W. Ma, C. Xu, S. Feng, L. Chen, M. L. Chen, D. M. Sun, X. Q. Chen, H. M. Cheng and W. C. Ren, Chemical vapor deposition of layered two-dimensional MoSi2N4 materials, Science, 2020, 369, 670–674 CrossRef CAS PubMed.
- L. Kang and Z. S. Lin, Second harmonic generation of MoSi2N4 – type layers, Phys. Rev. B, 2021, 103, 195404 CrossRef CAS.
- J. S. Yang, L. N. Zhao, S. Q. Li, H. S. Liu, L. Wang, M. D. Chen, J. F. Gao and J. J. Zhao, Accurate electronic properties and non-linear optical response of two-dimensional MA2Z4, Nanoscale, 2021, 13, 5479–5488 RSC.
Footnote |
† Electronic supplementary information (ESI) available: The method of theoretical calculation, band structure (HSE06), PDOS, calculated frequency-dependent coefficients and calculated refractive index dispersion curves of compounds. See DOI: https://doi.org/10.1039/d3qm00657c |
|
This journal is © the Partner Organisations 2023 |
Click here to see how this site uses Cookies. View our privacy policy here.