DOI:
10.1039/D2RA07898H
(Paper)
RSC Adv., 2023,
13, 6356-6367
Polyoxometalate supported on a magnetic Fe3O4/MIL-88A rod-like nanocomposite as an adsorbent for the removal of ciprofloxacin, tetracycline and cationic organic dyes from aqueous solutions†
Received
10th December 2022
, Accepted 17th February 2023
First published on 22nd February 2023
Abstract
In this work, a magnetic H3PW12O40/Fe3O4/MIL-88A (Fe) rod-like nanocomposite as a stable and effective ternary adsorbent was fabricated by the hydrothermal method and utilized for the removal of ciprofloxacin (CIP), tetracycline (TC) and organic dyes from aqueous solution. Characterization of the magnetic nanocomposite was accomplished by FT-IR, XRD, Raman spectroscopy, SEM, EDX, TEM, VSM, BET specific surface area and zeta potential analyses. The influencing factors on the adsorption potency of the H3PW12O40/Fe3O4/MIL-88A (Fe) rod-like nanocomposite including initial dye concentration, temperature and adsorbent dose were studied. The maximum adsorption capacities of H3PW12O40/Fe3O4/MIL-88A (Fe) for TC and CIP were 370.37 mg g−1 and 333.33 mg g−1 at 25 °C, respectively. In addition, the H3PW12O40/Fe3O4/MIL-88A (Fe) adsorbent had high regeneration and reusability capacity after four cycles. In addition, the adsorbent was recovered through magnetic decantation and reused for three consecutive cycles without a considerable reduction in its performance. The adsorption mechanism was mainly ascribed to electrostatic and π–π interactions. According to these results, H3PW12O40/Fe3O4/MIL-88A (Fe) can act as a reusable effective adsorbent for the fast elimination of tetracycline (TC), ciprofloxacin (CIP) and cationic dyes from aqueous solutions.
1. Introduction
The rapid development of industrialization, has led to an increasing number of water pollutants such as dyes and antibiotics entering the environment. Organic dyes are a potential hazard to human health due to their various harmful effects.1–4 The most important sources of industrial dye pollutants originate from different industries such as the textile, cosmetic, leather, food, pharmaceutical, paint and varnish, and pulp and paper industries.5–8 Antibiotics play a major role in human and animal disease treatment, growth promotion, and prophylaxis.9,10 Antibiotic pollution is becoming a serious problem. The main source of this contamination in the aquatic environment is wastewater from antibiotic manufacturers, large scale animal farming, and hospitals.11 Tetracycline (TC), which belongs to polyketides has broad-spectrum antibacterial activities against various disease-causing pathogens.12 Ciprofloxacin (CIP) is a type of antibiotic that is derived from the fluoroquinolones family, and used for healing the contagions induced by Gram-positive and Gram-negative bacteria.13 However, extensive use of antibiotics has resulted in their frequent detection in the effluents of wastewater treatment plants (WWTPs). The incomplete metabolism of humans or animals and the undesirable removal performance of traditional technologies caused the residual CIP and TC to be continuously discharged into the natural water. There are many methods to remove cationic dyes, CIP, and TC, such as electrochemical purification, membrane processes.14,15 biodegradation, and adsorption process using new adsorbents such as MOFs and Mxenes to remove different types of antibiotics and dyes.16–18 Adsorption is widely used as an excellent and promising technique due to the great advantages which are based on its lower expenses, higher performance, and convenience in use.19–21
Polyoxometalates (POMs) are a big group of metal–oxygen clusters having acid–base properties, consisting of primary transition metals such as tungsten, vanadium, and molybdenum.22,23 POMs are significant metal-oxide clusters with a highly negative charge and abundant topologies, which have been employed in many research fields such as optics, magnetism, catalysis, and biological medicine.24,25 However, there are some disadvantages for the use of POMs as adsorbents: (1) their relatively small surface area seriously obstructs accessibility to the active sites and (2) their excellent solubility in aqueous solution determines that they cannot be reused and recycled in the process of wastewater treatment. To solve these limitations, substrates including mof have been utilized to anchor polyoxometalates to the formation of heterogeneous composites.26
Metal–organic frameworks (MOFs) are hybrid crystalline porous materials with structures consisting of a regular array of positively charged metal ions or metallic clusters associated with organic linkers.27 MOFs are porous materials with fascinating structures. The high surface area, crystallinity, controllable pore size, flexibility, and fictionalization of the porous surface are some of the main characteristics which determine the versatility of MOFs.28,29 Among MOFs, one of the most attractive materials is MIL-88A (Fe). MIL-88A (Fe) was a 3D structured framework built up from trimers of Fe3+ octahedral linked to fumarate dianions. This structure exhibited a pore-channel system along the cages (5–7 Å).30 Thus, these features make MIL-88A (Fe) to be a good candidate for environmental contaminant elimination.
Based on the above considerations, in this paper, a magnetic H3PW12O40/Fe3O4/MIL-88A (Fe) rod-like nanocomposite was first fabricated by a hydrothermal method, and its chemical properties and physical structure were characterized. The adsorption of tetracycline (TC), ciprofloxacin (CIP), and organic dyes onto the H3PW12O40/Fe3O4/MIL-88A (Fe) and the influences of the adsorbent dose, primary adsorbate concentration and temperature on the adsorption process were checked.
2. Experimental
2.1. Materials and procedures
Fumaric acid, iron(III) chloride (FeCl3·6H2O, 99%), H3PW12O40 (98%), ammonium iron(III) sulfate ((NH4)Fe(SO4)2·12H2O, 98%), ammonium iron(II) sulfate ((NH4)2Fe(SO4)2·12H2O, 98.5%), ammonia NH3, methylene blue (C16H18ClN3S, MB, 99%), methyl orange (C14H14N3NaO3S, MO, 99%), and rhodamine B (C28H31ClN2O3,RhB, 99%) were obtained from Merck company. Tetracycline (C22H24N2O8, TC, 99.5%) and ciprofloxacin (C17H18FN3O3, CIP, 95.5%) drugs were obtained from Exir pharmaceutic company (Boroujerd, Iran). All of the chemicals were used without further purification.
2.2. Synthesis of iron-based MIL-88A (Fe) nanorods
The sample of MIL-88A (Fe) was prepared through a hydrothermal procedure. The accurate action to the synthesis of iron-based MOF is as follows: FeCl3·6H2O (1.2 g) and fumaric acid (0.49 g) were poured into 25 mL Water and the obtained combine was stirred for 15 min at ambient temperature. The mixture within a 30 mL Teflon-lined autoclave was transferred and warmed for 12 h at 65 °C. Then, it was slowly cooled at 25 °C and the obtained light brown solid was separated through centrifugation. The solid result was washed with water and also with ethanol solvent three times. Eventually, the product was dried in an oven for 1 h at 60 °C.
2.3. Synthesis of magnetic Fe3O4/MIL-88A (Fe) nanorods
The Fe3O4/MIL-88A (Fe) nanocomposite was produced as follows: FeCl3·6H2O (1.2 g), fumaric acid (0.49 g), Fe3O4 (0.1 g), in 25 mL of water solvent was poured and the combine was stirred for 15 min at 25 °C. The mixture within a 30 mL Teflon-lined autoclave was transferred and warmed at 65 °C for 12 h. Afterward slowly cooled at 25 °C and the light brown solid product was separated the solid product was detached from the solution by applying a magnet and then washed with Water solvent and dried in an oven at 70 °C for 1 h.
2.4. Synthesis of H3P2W12O40/Fe3O4/MIL-88A (Fe) rod-like composite
For the fabrication of H3PW12O40/Fe3O4/MIL-88A (Fe), 1 g of the Fe3O4/MIL-88A (Fe) and 1 g of H3PW12O40 were solved within 20 mL of deionized water. The combine was stirred at 25 °C for 20 h. The resulting solid was washed through deionized water and cooled gradually at 25 °C. Finally, the solid product was detached from the solution by applying a magnet.
2.5. Characterization methods
FTIR spectra were registered with a Shimadzu-8400S spectrometer (Japan) in the wavenumber range of 400–4000 cm−1. Powder XRD patterns were recorded by an X-ray diffractometer under a current of 40 mA and voltage of 40 kV with Cu Kα radiation (k = 0.1542 nm). The distribution and morphology of pure MOF and magnetic nanocomposite were studied utilizing (SEM, MIRA3 TESCAN) scanning electron microscopy connected with (EDX) energy-dispersive X-ray analysis. Spectra of UV-vis were performed on a Carry 100 Conc Varian spectrophotometer. The surface area of the nanocomposites was performed by N2 adsorption isotherm with the BET method (Micro metrics PHS-1020, Japan). The magnetic (VSM) measurement was checked by MDKFD vibrating magnetometer (Daneshpajoohan Co., Iran) via a high magnetic field of 10 kOe. The adsorption process of dyes was measured on a Varian Cary 100 spectrophotometer (USA). Raman spectra were obtained using a Raman microscope (Senterra 2009, Germany) with a 514 nm line.
2.6. Adsorption experiments of drug
The adsorption of TC and CIP was performed in a 50 ml glass beaker and the adsorption reaction temperature was maintained at 25 °C. The removal experiments were carried out in 50 ml reaction volume of TC and CIP 10 mg L−1 and the parameters chosen for the optimization are nanoparticle concentration: 25–100 mg L−1 and temperature: 25–65 °C. After the reaction was completed, the adsorbent was separated with an external magnet. All parallel experiments were performed in triplicate to ensure accuracy, and the average results were employed for further data analysis. The main absorbance peak of TC and CIP was observed at 375 and 326 nm and its removal efficiency were calculated according to the following equation: The degradation efficiency (R (%)) is defined as (Co − Ct)/Co × 100%, where Co is the initial concentration of TC and CIP solution and Ct is the remaining concentration of CIP at reaction time t.
R (%) = (Co − Ct) × 100/Co |
and equilibrium adsorption capacity qe (mg g−1) could be computed:
2.7. Adsorption experiments of organic dyes
The adsorption experiments were carried out using cationic dyes i.e., MB, rhodamine B (RhB), and anionic dye, i.e., methyl orange (MO) as model organic dye pollutants of the water at 25 °C. In a typical experiment, 20 mg of the as-prepared H3PW12O40/Fe3O4/MIL-88A (Fe), the hybrid adsorbent was added into 30 ml of dye aqueous solution with the initial concentration of 30 mg L−1 and stirred in the dark. The concentrations of MB, RhB, and MO were determined using UV-visible spectrophotometry at wavelengths of 664, 553, and 467 nm, respectively. The effects of important parameters on the adsorption performance, like amount of nanocomposite (10, 20, 30 and 40 mg) and initial dye concentration (25, 50, 75, 125, 150 and 200 mg), were studied under similar conditions as described above. To evaluate the selective adsorption ability of the hybrid nanomaterial, 20 mg of H3PW12O40/Fe3O4/MIL-88A (Fe) was stirred with 30 ml of mixed dyes of MB + MO (Co (MB) = Co (MO) = 30 mg L−1), MB + RhB (Co (MB) = Co (RhB) = 30 mg L−1), MO + RhB (Co (MB) = Co (RhB) = 30 mg L−1) and the ternary mixture of MB + MO + RhB (Co (MB) = Co (MO) = Co (RhB) = 30 mg L−1) and then the process was monitored using UV-visible spectroscopy at given time intervals. The elimination rate (R%) was computed based on the following equation:
(R%) = (Co − Ct) × 100/Co |
3. Results and discussion
3.1. Characterization of the adsorbent
3.1.1. FTIR analysis. The FT-IR spectra of MIL-88A (Fe) (a), Fe3O4 (b), H3PW12O40 (c), Fe3O4/MIL-88A (Fe) (d), and H3PW12O40/Fe3O4/MIL-88A (Fe) rod-like composite (e) are displayed in Fig. 1. In Fig. 1(a), the broadband at about 3465 cm−1 is related to the stretching vibration of water molecules. The two sharp peaks at 1400 and 1610 cm−1 are assigned to symmetric and asymmetric vibrations of carboxyl groups, respectively, confirming the presence of the dicarboxylate linker within the sample.31 Also, the peak that appeared at 574 cm−1 is specified to the Fe–O vibration. The FT-IR spectrum of Fe3O4 (Fig. 1(b)) demonstrated an adsorption peak at 567 cm−1 which is related to the vibration of the Fe–O bond of Fe3O4.5 As revealed in Fig. 1(c), the characteristic peaks of 789 and 898 cm−1 are related to the vibration of (W–Ob–W), and the bands appeared at 960, and 1083 cm−1 are assigned to the vibrations of (W–Od) and (P–Oa) of H3PW12O40 polyanion, respectively.32 Comparing the spectra of H3PW12O40/Fe3O4/MIL-88A (Fe) samples (Fig. 1(d)) shows that after forming this nanocomposite, the characteristic peaks related to iron-based MIL-88A, H3PW12O40, and Fe3O4 particles are slightly shifted. These shifts emphasize that a strong interaction exists between the H3PW12O40 anion and Fe3O4 nanoparticles with MIL-88A (Fe) MOF.
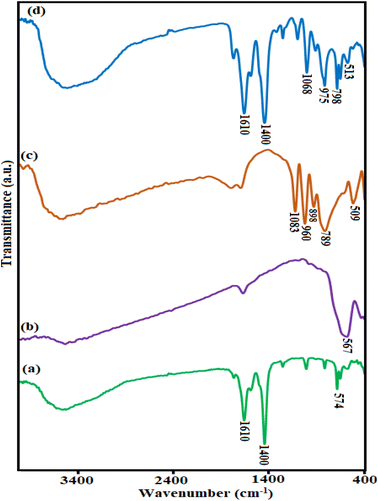 |
| Fig. 1 The FTIR spectra of (a) MIL-88A (Fe), (b) Fe3O4, (c) H3PW12O40, and (d) the H3PW12O40/Fe3O4/MIL-88A Fe nanocomposite. | |
3.1.2. XRD diffraction patterns. The crystallinity and phase of the fabricated samples MIL-88A (Fe), Fe3O4, H3PW12O40, Fe3O4/MIL-88A (Fe), and H3PW12O40/Fe3O4/MIL-88A (Fe) rod-like composite were analyzed via XRD as elucidated in Fig. 2. As shown in Fig. 2(a), the XRD pattern of MIL-88A showed two diffraction peaks at 2θ = 10–12°, which was accordance with the reported information.31 As exhibited in Fig. 2(b), the typical peaks at 2θ = 75°, 63°, 53.7°, 43.3°, 36°, and 30.2° assigning to (440), (511), (422), (400), (311), and (220) the crystal planes of Fe3O4 particles (JCPDS card No. 19-0629).33 In Fig. 2(c), the diffraction peaks of POM alone are observed. The XRD pattern of the nanocomposite sample in Fig. 2(d) exhibits the characteristic diffraction peaks corresponding to Fe3O4 and MIL-88A. Also, some diffraction peaks contributed to H3PW12O40 phase are observed with low intensities because of the homogeneous distribution of the H3PW12O40 molecules within the porous structure of MIL-88A (Fe). These findings confirm that the nanocomposite sample is composed of Fe3O4, MIL-88A and H3PW12O40 components and it has been successfully prepared.34
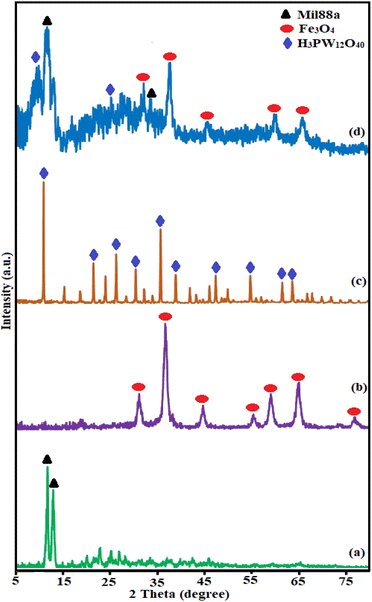 |
| Fig. 2 The XRD patterns of (a) MIL-88A (Fe), (b) Fe3O4, (c) H3PW12O40, and (d) the H3PW12O40/Fe3O4/MIL-88A (Fe) nanocomposite. | |
3.1.3. Raman spectroscopy. Raman spectroscopy was used to confirm the structural phase of MIL-88A (Fe), H3PW12O40, H3PW12O40/MIL-88A (Fe), and H3PW12O40/Fe3O4/MIL-88A (Fe) nanomaterials. In Fig. S1(a),† the bond of the fumaric acid molecule in MIL-88A was evidenced by the appearance of a band at 3059 cm−1, corresponding to the stretching sp2 C–H vibrations, and a strong peak at 1649 cm−1 associated to the symmetric vibration modes C
C. The bands located at 1592 and 1435 cm−1, in the case of MIL-88A, materials are attributed to the anti-symmetric and symmetric vibrations, respectively, of the carboxylate groups. The C–O bond was observed as an intense peak centered at 1280 cm−1. Additionally, the bending vibrations outside the plane of the bond
C–H were placed at Raman shifts of 998, 896, and 763 cm−1.35 As exhibited in Fig. S1(b),† the Raman spectrum of H3PW12O40 shows characteristic bands at 1010 cm−1 (stretching vibration of P–O), 990 cm−1 (stretching of W
O), 913 cm−1 (bending of W–Oc–W) and 519 cm−1 (bending of O–P–O). According to Fig. S1(c),† the appearance of some vibrational modes of the Fe3O4 and three bands of H3PW12O40 at 292, 751, and 1010 cm−1, beside the characteristic bands of MIL-88A (Fe), proved the existence of both Fe3O4 and H3PW12O40 in the framework of MIL-88A (Fe).
3.1.4. SEM and TEM images. The morphology and microstructure of the iron-based MIL-88A (Fe), Fe3O4, Fe3O4/MIL-88A (Fe), and H3PW12O40/Fe3O4/MIL-88A (Fe) samples were studied by utilizing SEM analyses as depicted in Fig. 3. According to Fig. 3(a) pure iron-based MIL-88a particles have a shape bar-like with two prismatic heads. In Fig. 3(b), the SEM image of Fe3O4 consists of small and near-spherical particles which is highly agglomerated. The SEM photographs of the binary Fe3O4/MIL-88A (Fe) (Fig. 3(c)) and ternary H3PW12O40/Fe3O4/MIL-88A (Fe) nanocomposite (Fig. 3(d) and (e)) demonstrate that the morphology and shape of these samples are analogous to MIL-88A (Fe), which established that the framework of iron-based MIL-88A (Fe) remains unaltered after modification with Fe3O4 nanoparticles and H3PW12O40. The morphology and microstructure of the as-prepared H3PW12O40/Fe3O4/MIL-88A (Fe) nanocomposite were further investigated by TEM analysis. As evident from the image in Fig. 3(f), the morphology of the H3PW12O40/Fe3O4/MIL-88A (Fe) rod-like composite sample from TEM images agreed with the SEM results.
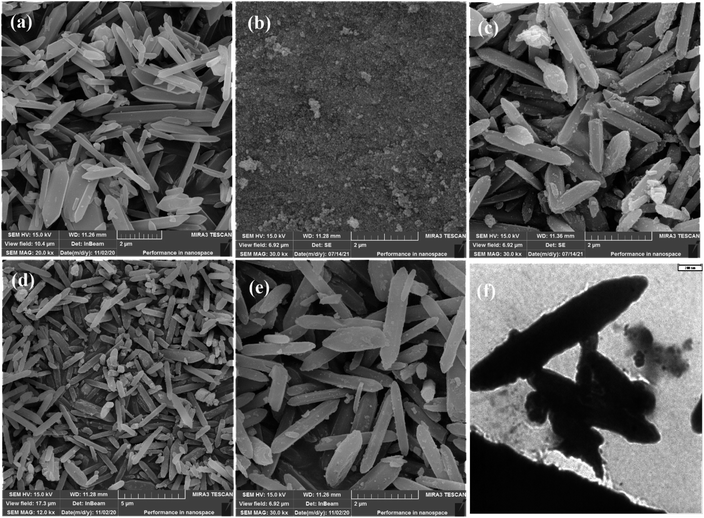 |
| Fig. 3 The SEM photographs of (a) iron-based MIL-88A, (b) Fe3O4, (c) Fe3O4/MIL-88A (Fe), (d and e) the H3PW12O40/Fe3O4/MIL-88A Fe nanocomposite, and (f) TEM image of the H3PW12O40/Fe3O4/MIL-88A (Fe) nanocomposite. | |
3.1.5. EDX analysis. The EDX analysis of the H3PW12O40/Fe3O4/MIL-88A (Fe) rod-like composite is displayed in Fig. S2.† In Fig. S2(a),† the EDX elemental spectrum of the ternary nanohybrid sample exhibits elemental peaks corresponding to H3PW12O40 (O, P and W), Fe3O4 (Fe, O), and MIL-88A (Fe) (C, Cl, and Fe), with no other impure peaks observed, showing that the composite sample was composed of H3PW12O40, Fe3O4, and MIL-88A (Fe). Further, the EDX elemental mappings of the composite in Fig. S2(b)† show the homogeneous dispersion of elements within the nanocomposite.
3.1.6. BET analysis. The N2 adsorption–desorption isotherms of the activated MIL-88A (Fe) and H3PW12O40/Fe3O4/MIL-88A (Fe) are illustrated in Fig. 4(a) and (b). The Brunauer–Emmett–Teller (BET) surface area values for MIL-88A (Fe) and H3PW12O40/Fe3O4/MIL-88A (Fe) were calculated to be 3.19 m2 g−1 and 4.16 m2 g−1, respectively, in agreement with literature.24 Their average pore diameter of them was measured as 41.2 nm and 47.4 nm, respectively. The BJH pore-size dispersions in the inset of the figures affirm the mesoporous cages of MIL-88A (Fe) and showed that the overall volume changes through the incorporation. The results suggested that the H3PW12O40 polyoxometalate was mostly encapsulated within the channels and on the surface of MIL-88A (Fe).
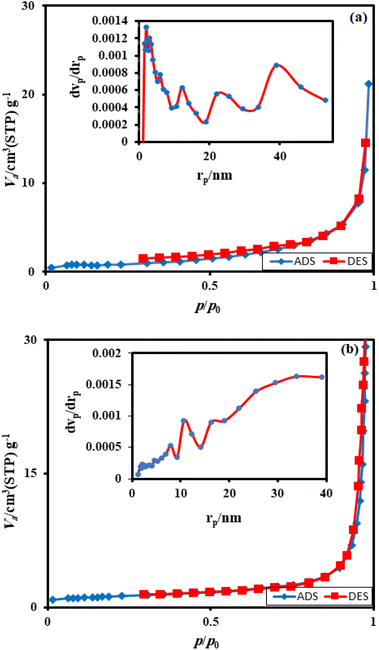 |
| Fig. 4 N2 adsorption–desorption isotherms and pore-size distribution curves (the insets) of (a) MIL-88A (Fe) and (b) the H3PW12O40/Fe3O4/MIL-88A (Fe) nanocomposite. | |
3.1.7. VSM analysis. The magnetic properties of the pure Fe3O4, Fe3O4/MIL-88A (Fe), and H3PW12O40/Fe3O4/MIL-88A (Fe) samples were investigated by VSM at room temperature, and the magnetic hysteresis loops are depicted in Fig. 5(a)–(c). The magnetic saturation values (Ms) of the Fe3O4 nanoparticles, Fe3O4/MIL-88A and H3PW12O40/Fe3O4/MIL-88A (Fe) were about 72, 51 and 43 emu g−1, respectively, which was sufficient for quick separation, via the aid of an outer magnet. This value was less than that of bare Fe3O4 (about 51 emu g−1) because of the existence of nonmagnetic H3PW12O40 and MIL-88A (Fe) components. The magnetic separability of the H3PW12O40/Fe3O4/MIL-88A (Fe) nanocomposite was disclosed by approaching the glass container with a magnet.
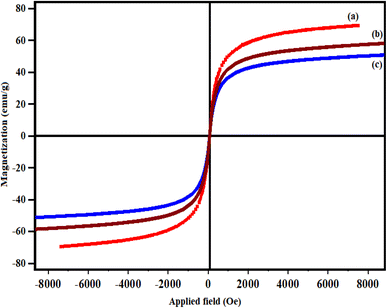 |
| Fig. 5 Magnetization curves of (a) Fe3O4, (b) Fe3O4/MIL-88A (Fe) and (c) the H3PW12O40/Fe3O4/MIL-88A (Fe) nanocomposite. | |
3.1.8. Zeta potential analysis. The zeta potential investigation results got for analyzed MIL-88A (Fe) and H3PW12O40/Fe3O4/MIL-88A (Fe) are presented in Fig. 6. As shown in Fig. 6, the positive zeta potential value of MIL-88A (Fe) (+4.59 mV) is changed to a negative charge for the H3PW12O40/Fe3O4/MIL-88A (Fe) composite (−17.85 mV). This significant change in zeta potential value is mostly because of the introducing the negatively charged PW12O403− component into the MIL-88A (Fe) framework and makes it more suitable for selective adsorption of cationic species.
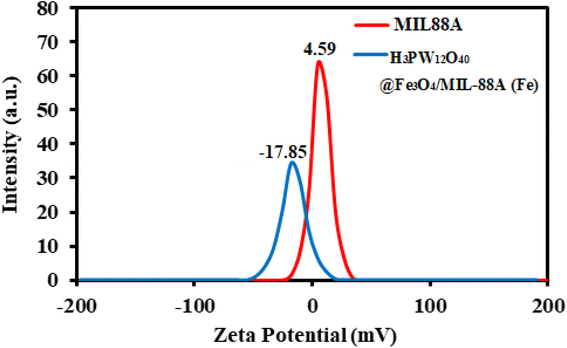 |
| Fig. 6 Zeta potential distribution curve of the MIL-88A (Fe) and H3PW12O40/Fe3O4/MIL-88A (Fe) samples in aqueous solutions. | |
3.2. TC and CIP drugs adsorption investigations
Different factors affect the adsorption process. The effect of H3PW12O40/Fe3O4/MIL-88A (Fe) dosage, temperature and primary concentration of TC and CIP were investigated by a variable-controlling strategy. To determine the optimum adsorbent dosage, we explored the adsorbent effect on the TC and CIP removal efficiency. As shown in Fig. 7(a) and (b), the adsorption efficiency for TC is about 85% in 3 min and for CIP is approximately 100% in 12 min.
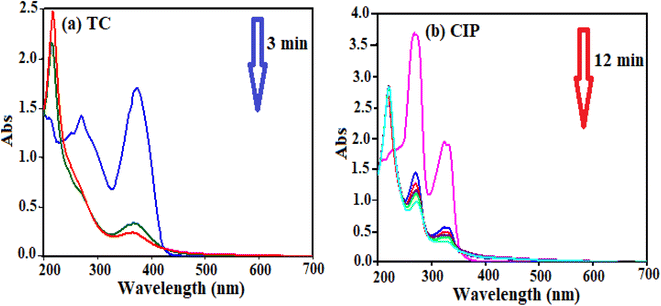 |
| Fig. 7 UV-vis spectral changes of (a) TC and (b) CIP drugs in aqueous solutions over the H3PW12O40/Fe3O4/MIL-88A (Fe) nanocomposite at different time intervals: conditions: Co (drug) = 50 mg L−1, Vdrug = 50 mL, adsorbent dose: 10 mg at 25 °C. | |
3.2.1. Influence of adsorbent dose. Different amounts of H3PW12O40/Fe3O4/MIL-88A (Fe) nanocomposite (5, 10, 15, 20 mg) were added to investigate the influence of adsorbent dose on the CIP and TC removal efficiency. The results in Fig. 8(a) and (b) show that the removal efficiency increased with the nanocomposite dosage from 5 to 20 mg.17,32 As expected, the removal (%) of drugs sharply increased with increasing the H3PW12O40/Fe3O4/MIL-88A (Fe) nanocomposite composite dosage; this mainly attributed to the presence of further active sites for adsorption of drugs molecules. According to the above results, the amount of 10 mg was selected used for the subsequent adsorption experiments.
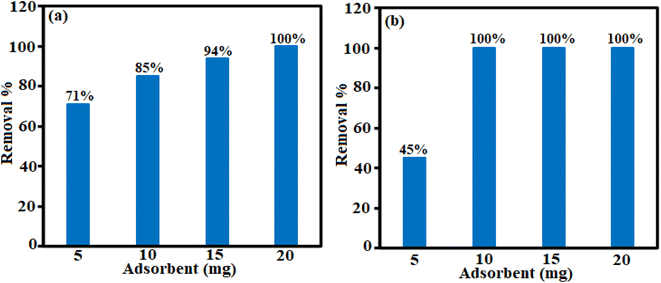 |
| Fig. 8 Effect of adsorbent doses on the removal of (a) TC and (b) CIP antibiotics. | |
3.2.2. Influence of temperature. The adsorption behavior of H3PW12O40/Fe3O4/MIL-88A (Fe) demonstrated at different temperatures in Fig. 9. As shown in Fig. 9(a), the progressive increase of temperature from 25 to 65 °C is adversely affecting the removal of 10 mg L−1 of TC by H3PW12O40/Fe3O4/MIL-88A (Fe) nanocomposite, because the removal efficiency increase from 85% to 100%. This shows that the reaction between TC and H3PW12O40/Fe3O4/MIL-88A (Fe) nanoparticles is the endothermic method. In Fig. 9(b), the influence of temperature on the elimination of CIP via H3PW12O40/Fe3O4/MIL-88A (Fe) nanocomposite was checked at several temperatures from 25 to 65 °C under an adsorbent dosage of 10 mg. The results indicate that the adsorption process between ciprofloxacin and H3PW12O40/Fe3O4/MIL-88A (Fe) nanoparticles is exothermic.36,37
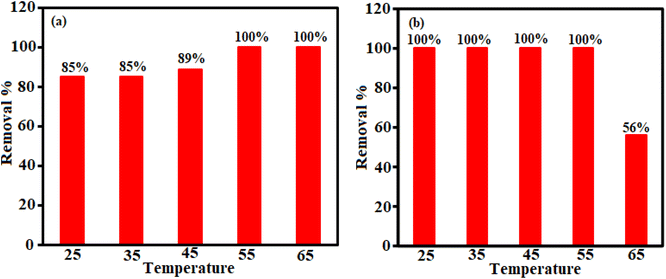 |
| Fig. 9 Effects of temperature on the removal of (a) TC and (b) CIP antibiotics. | |
3.2.3. Influence of primary TC and CIP concentration. The influence of the various initial concentrations (25, 50, 75, and 100 mg L−1) of TC and CIP drugs on the adsorption efficiency of H3PW12O40/Fe3O4/MIL-88A (Fe) adsorbent under the constant conditions of 50 mg L−1 absorbent, pH 6, and 25 °C are shown in Fig. 10. As can be seen in Fig. 10(a) and (b), the removal efficiency decreased with increasing the CIP and TC concentrations, probably due to the fast saturation of accessible active sites on the surface of adsorbent in higher initial concentration of drugs and the deficiency of active sites needed for high drug concentration.32
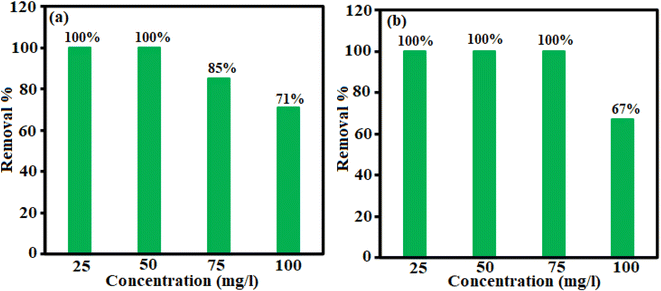 |
| Fig. 10 Effect of concentration on the removal of (a) TC and (b) CIP antibiotics. | |
3.2.4. Removal efficiency of the nanocomposite components. The removal efficiency of pure MIL88A (Fe) and Fe3O4/MIL101 (Fe) in the elimination of TC and CIP from aqueous solutions were studied under the optimum reaction conditions in Fig. 11. The removal efficiency of these components into TC and CIP was 59–85% and 35–100% after adsorption times of 3–12 minutes and 12–24 minutes, respectively. The elimination percentage of the H3PW12O40/Fe3O4/MIL-88A (Fe) nanocomposite is higher than the individual components and Fe3O4/MIL101 (Fe) composite, which showed the magnetic nanocomposite to be a better choice for the removal of the antibiotic drugs.
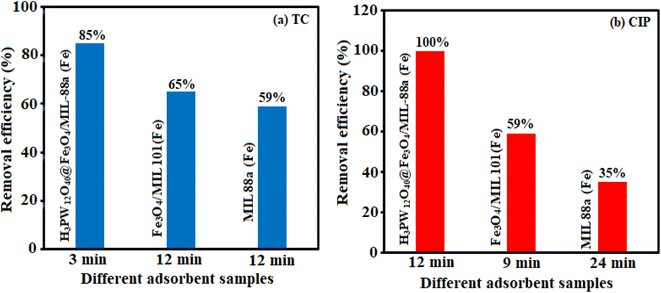 |
| Fig. 11 The efficiency of the nanocomposite components on the removal of (a) TC and (b) CIP antibiotics. | |
3.2.5. Comparison with some reported adsorbents. To indicate the advantage of the present adsorbent, we have compared the results in the removal of TC and CIP from aqueous solution by H3PW12O40/Fe3O4/MIL-88A (Fe) nanocomposite with some reported similar adsorbents in the literature.16,17,38–53 From Table 1, concerning the adsorption capacities, the present method is more suitable and/or superior. We can see that the adsorption process in the presence of some reported adsorbents demonstrated lower adsorption capacities compared to the present nanocomposite. The higher adsorption capacity of the H3PW12O40/Fe3O4/MIL-88A (Fe) is the because of the synergistic effect between MIL-88A (Fe) and H3PW12O40.
Table 1 Comparison of the maximum adsorption capacities of some reported adsorbents for TC and CIP drugs
Adsorbent |
Drug |
Maximum adsorption capacity (mg g−1) |
Ref. |
HAP/MIL101 (Fe)/Fe3O4 |
CIP |
112.35 |
16 |
Fe3O4/cellulose |
CIP |
6.90 |
17 |
Fe3O4/cellulose |
CIP |
168.03 |
17 |
CS-g-PA/TSM |
CIP |
238.3 |
38 |
MIL101 (Cr)–HSO3 |
CIP |
564.9 |
39 |
c-FD |
CIP |
328 |
40 |
mGOCP |
CIP |
283.44 |
41 |
Ga-cl-PAM/C3N4 |
CIP |
169.49 |
42 |
Fe/Zn-SBC |
CIP |
74.2 |
43 |
Fe/Zn-SBC |
TC |
145 |
43 |
Fe3O4/MIL-53 (Al)–NH2 |
TC |
84.8 |
44 |
MnO2/graphene |
TC |
195.31 |
45 |
Graphene oxide/ZnO |
TC |
159 |
46 |
Fe3O4@MIL-53 (Al) |
TC |
47.8 |
47 |
MnFe2O4/rGO |
TC |
41 |
48 |
Scallop shell coated Fe2O3 nanocomposite |
TC |
49.26 |
49 |
CTM/Fe3O4 |
TC |
215.31 |
50 |
CDF/MF |
TC |
168.24 |
51 |
MWCNT/MIL-53 (Fe) |
TC |
180.68 |
52 |
CNT/MnFe2O4 |
TC |
86.48 |
53 |
CNT/β-CD/MnFe2O4 |
TC |
89.53 |
53 |
ZnAl-LDH/biochar |
TC |
41.98 |
54 |
H3PW12O40/Fe3O4/MIL-88A (Fe) |
TC |
370.37 |
This work |
H3PW12O40/Fe3O4/MIL-88A (Fe) |
CIP |
333.33 |
This work |
3.3. Dye adsorption investigations
To study and evaluate the absorption potential of H3PW12O40/Fe3O4/MIL-88A (Fe) nanocomposite for the removal of dyes from contaminated waters, two organic dyes (MB and MO) with different charges were used for initial experiments. The adsorption was monitored using their special absorption bands, which are 664, and 463 nm for MB and MO, respectively. The time-dependent UV-visible absorption spectra of dyes in the presence of H3PW12O40/Fe3O4/MIL-88A (Fe) are shown in Fig. 12. Fig. 12(a) and (b) shows that the characteristic absorption band of cationic MB dye at 664 nm almost completely disappeared within 6 min but the disappearance of band at about 463 nm for MO is almost negligible. This result shows that the nanocomposite is a poor absorbent for removing anionic MO dye from aqueous solution.
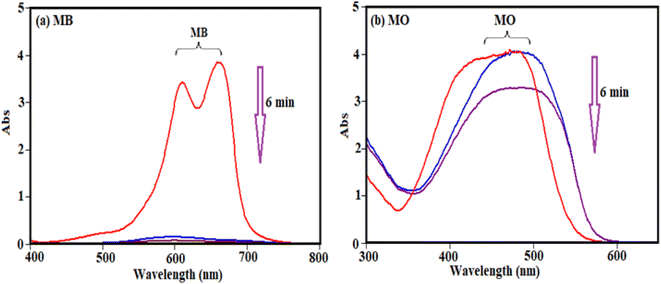 |
| Fig. 12 The UV-vis spectra during adsorption of (a) cationic MB and (b) anionic MO dyes over the H3PW12O40/Fe3O4/MIL-88A (Fe): Co (dye) = 30 mg L−1, Vdye = 30 mL, adsorbent dosage = 20 mg, temp = 25 °C. | |
The selective uptake of dyes was investigated using the binary mixtures of MB + MO, MB + RhB, MO + RhB, and MB + MO + RhB with H3PW12O40/Fe3O4/MIL-88A (Fe) as absorbent. As shown in Fig. 13(a) and (b), the preferable uptake of cationic MB and RhB dyes from the MB + MO and RhB + MO mixtures can be attributed to the anionic nature of H3PW12O40/Fe3O4/MIL-88A (Fe). For further comparison, the binary mixture of cationic dyes (MB + RhB) was selected and the results in Fig. 13(c) revealed that MB and RhB were also adsorbed completely in the initial 3 min. As exhibited in Fig. 13(d), the representative peaks of MB + MO + RhB all disappeared quickly for the mixed dyes, and only the characteristic absorption peaks of MO remained, suggesting that H3PW12O40/Fe3O4/MIL-88A (Fe) could selectively capture cationic dyes when utilized in the corresponding ternary mixture. This finding confirms that H3PW12O40/Fe3O4/MIL-88A (Fe) nanocomposite also possesses selective adsorption ability towards cationic dyes in wastewater. So, the H3PW12O40/Fe3O4/MIL-88A (Fe) nanocomposite exceptional adsorption properties towards the cationic MB and RhB molecules were clearly demonstrated. Some diverse effects on removal of colorants can be linked to the structure of the dye molecules and the nanocomposite as well. This is due to the existence of vastly electronegative PW12O403−, which mostly enhanced the adsorption ability of the porous material MIL-88A, which together with enormous numbers of negative charges may have a stronger force with the positive charges of the colorants. The fabricated material in this study is not an efficient adsorbent for the removal of MO, because of negative charges of both the adsorbent and dye molecules. As a consequence, there is neither electrostatic attraction nor adsorption property between the PW12O403− encapsulated into the nanocomposite and MO molecules.
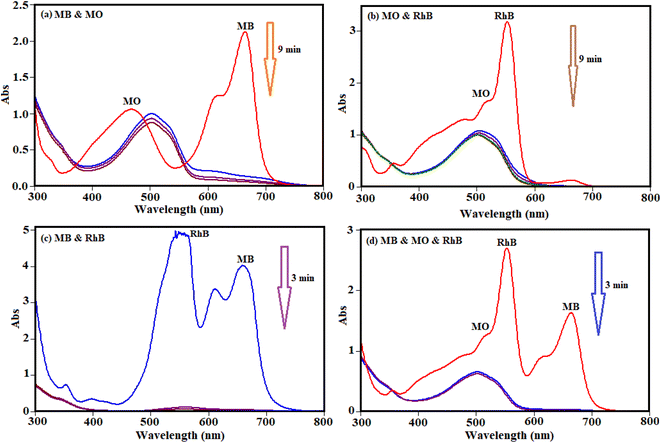 |
| Fig. 13 Selective adsorption capability of H3PW12O40/Fe3O4/MIL-88A (Fe) into the combined solution of (a) MB + MO, (b) MB + RhB, (c) MO + RhB, and (d) MB + MO + RhB. Conditions: Co (MB) = Co (RhB) = Co (MO) = 35 mg L−1, and adsorbent dosage = 20 mg in 30 mL. | |
3.4. Possible adsorption mechanism
The behavior of TC and MB adsorption onto H3PW12O40/Fe3O4/MIL-88A (Fe) were studied by the adsorbent properties, the TC and MB nature, electrostatic interaction, π–π interaction, hydrogen bonding, van der Waals, hydrophobic interaction sand surface complexation in this study. MB has a flat extended conjugated structure, which has stronger π–π stacking interactions compared to the TC. In addition, MB is a cationic dye, which exists as positively charged in an aqueous solution. The adsorption efficiency of the MIL-88A (Fe), sample, despite its higher surface area, was lower than that of the H3PW12O40/Fe3O4/MIL-88A (Fe) composite. This confirms that other factors influence the adsorption efficiency besides to the specific surface area of the adsorbent. After introducing the PW12O403− polyanions into the cavities of MIL-88A (Fe), it was confirmed that the adsorption capacity of the nanocomposite changed, in comparison with that of MIL-88A (Fe). This is because of the rapid and effective adsorption of cationic MB dye by PW12O403− polyanions, whereas it is unfavorable for H3PW12O40/Fe3O4/MIL-88A (Fe) to adsorb the anionic dye MO. These findings show that the PW12O403− polyoxoanion clusters encapsulated in the mesoporous cages of MIL-88A (Fe) act as the active sites for the adsorption of cationic dye molecules. The zeta potential of a material is another key factor influencing its adsorption capacity; therefore, this was tested to understand further why the H3PW12O40/Fe3O4/MIL-88A (Fe) sample can remove the cationic dyes MB more effectively than the anionic MO dye. The zeta potentials of MIL-88A (Fe) and H3PW12O40/Fe3O4/MIL-88A (Fe) samples were measured as +4.59 and −17.85 mV, respectively, in water at natural pH. After modification with H3PW12O40, the H3PW12O40/Fe3O4/MIL-88A (Fe) nanocomposite showed a more negative zeta potential than the pristine MIL-88A (Fe) sample, thus, it can adsorb more of the cationic dyes such as MB, whereas it is unfavorable to adsorb the anionic dyes such as MO.
Also in this work, the adsorption efficiency of H3PW12O40/Fe3O4/MIL-88A (Fe) was found to be higher as compared to pure H3PW12O40, MIL-88A (Fe), and Fe3O4/MIL-88A (Fe). First, there are many open metal sites on MIL-88A (Fe) that facilitate the adsorption of specific guest molecules with corresponding functional groups.55,56 Since the adsorption could happen inside the MOFs, hydrogen bonding may also occur during the tetracycline adsorption process. TC molecule has a complex structure with several carbonyls, amino and hydroxyl functional groups, and O-containing groups of the H3PW12O40/Fe3O4/MIL-88A (Fe) composite such as OH, NH2 and carboxylate.57 The aromatic structure of the drugs molecule adsorption onto MIL-88A (Fe), series mainly occurred by π–π interaction. The O-containing groups in MIL-88A (Fe) and PW12O403− play an important role in the adsorption process.58 The reason for high adsorption of TC and MB on samples should be further investigated.
3.5. Stability and reusability of the magnetic nanocomposite
To check the reusability of the H3PW12O40/Fe3O4/MIL-88A (Fe) as an adsorbent, cycle experiments were carried out by applying a combination of methanol and deionized water as desorption solvents. For this goal, 10 mg of nanocomposite was added in TC and CIP solutions with a concentration of 50 mg L−1, and stirred for 30 minutes, in continuing the nanocomposite was thoroughly detached by utilizing a magnet. In the next, the magnetic nanocomposite was eluted with a methanol solution several times and finally dried for 12 h at 25 °C and reused for another adsorption process under the same condition. The results in Fig. 14(a) show the adsorbent is reusable for at minimum 3 runs without notable loss of activity. As indicated in Fig. 14 (b)–(d), SEM, FT-IR, and XRD analyses of the recycled H3PW12O40/Fe3O4/MIL-88A (Fe) after 4 runs were identical to the fresh nanocomposite. These results established that the structure of the magnetic nanocomposite was stable and was not altered by the reactants.
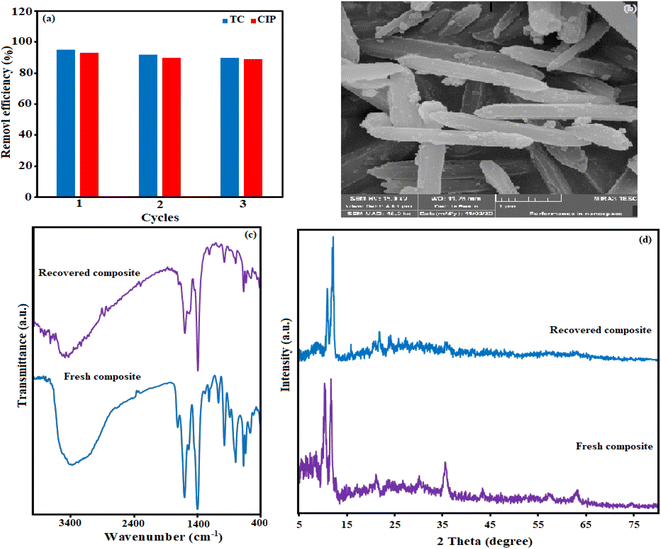 |
| Fig. 14 (a) Reusability of the H3PW12O40/Fe3O4/MIL-88A (Fe) nanocomposite in the removal of TC and CIP antibiotics, (b) SEM image, (c) FT-IR spectrum and (d) XRD pattern of the fresh and recovered nanocomposite after three runs. | |
4. Conclusions
In summary, a magnetically separable H3PW12O40/Fe3O4/MIL-88A (Fe) adsorbent was successfully prepared using a hydrothermal method and its adsorptive property of organic dyes and antibiotics entering the environment were investigated. The tests showed that the magnetic nanocomposite H3PW12O40/Fe3O4/MIL-88A (Fe) offers high removal efficiency against cationic dyes, TC, and CIP antibiotics. The maximum adsorption capacities of TC and CIP drugs onto H3PW12O40/Fe3O4/MIL-88A (Fe) composite were found to be 370.33 and 333.33 mg g−1, respectively. Also, reusability tests showed that H3PW12O40/Fe3O4/MIL-88A (Fe) composite can be reused for three cycles without a considerable reduction in its performance. As a result, magnetic nanocomposite could be used as an efficient to remove cationic dyes antibiotics from water.
Conflicts of interest
There are no conflicts to declare.
Acknowledgements
The authors give their sincere thanks to the Lorestan University and Iran Nanotechnology Initiative Council (INIC) for all provided supports.
References
- R. Hajavazzadeh, M. Kargar Razi and A. R. Mahjoub, Int. J. Nano Dimens., 2021, 12, 67–75 Search PubMed.
- A. Golshan Tafti, A. Rashidi, H.-A. Tayebi and M. E. Yazdanshenas, Int. J. Nano Dimens., 2018, 9, 79–88 Search PubMed.
- S. Ahmadi, L. Mohammadi, A. Rahdar, S. Rahdar, R. Dehghani, C. Adaobi Igwegbe and G. Z. Kyzas, Nanomaterials, 2020, 10, 556 CrossRef CAS.
- S. Rahdar, A. Rahdar, M. N. Zafar, S. S. Shafqat and S. Ahmadi, J. Mater. Res. Technol., 2019, 8, 3800–3810 CrossRef CAS.
- A. Jarrah and S. Farhadi, J. Solid State Chem., 2020, 285, 121264 CrossRef CAS.
- L. Zhu, L. Zong, X. Wu, M. Li, H. Wang, J. You and C. Li, ACS Nano, 2018, 12, 4462–4468 CrossRef CAS.
- Z. Wang, L. Song, Y. Wang, X.-F. Zhang and J. Yao, J. Phys. Chem. Solids, 2021, 150, 109839 CrossRef CAS.
- M. Fayazi, D. Afzali, R. Ghanei-Motlagh and A. Iraji, Environ. Sci. Pollut., 2019, 26, 18893–18903 CrossRef CAS.
- X. Song, J. Mo, Y. Fang, S. Luo, J. Xu and X. Wang, Environ. Sci. Pollut., 2022, 29, 35204–35216 CrossRef CAS.
- G. Gopal, H. Sankar, C. Natarajan and A. Mukherjee, J. Environ. Manage., 2020, 254, 109812 CrossRef CAS.
- C. Liu, L. Tan, L. Zhang, W. Tian and L. Ma, Front. Environ. Sci., 2021, 9, 692298 CrossRef.
- Y. Zhang, Z. Jiao, Y. Hu, S. Lv, H. Fan, Y. Zeng, J. Hu and M. Wang, Environ. Sci. Pollut., 2017, 24, 2987–2995 CrossRef CAS.
- N. A. Elessawy, M. Elnouby, M. H. Gouda, H. A. Hamad, N. A. Taha, M. Gouda and M. S. M. Eldin, Chemosphere, 2020, 239, 124728 CrossRef CAS.
- Z. Liu, M. Zhu, L. Zhao, C. Deng, J. Ma, Z. Wang, H. Liu and H. Wang, Chem. Eng. J., 2017, 314, 59–68 CrossRef.
- X. Liu, D. Yang, Y. Zhou, J. Zhang, L. Luo, S. Meng, S. Chen, M. Tan, Z. Li and L. Tang, Chemosphere, 2017, 182, 306–315 CrossRef CAS.
- M. Beiranvand, S. Farhadi and A. Mohammadi-Gholami, RSC Adv., 2022, 12, 34438–34453 RSC.
- A. Azizi, J. Iran. Chem. Soc., 2021, 18, 331–341 CrossRef CAS.
- L. Zhang, P. Ma, L. Dai, S. Li, W. Yu and J. Guan, Catal. Sci. Technol., 2021, 11, 3834–3844 RSC.
- M. Martínez-Cabanas, M. Lopez-Garcia, J. L. Barriada, R. Herrero and M. E. S. de Vicente, Chem. Eng. J., 2016, 301, 83–91 CrossRef.
- J. Yang, Q. Xiao, X. Jia, Y. Li, S. Wang and H. Song, J. Hazard. Mater., 2021, 403, 124016 CrossRef CAS.
- W. Wang, W. Zhao, H. Zhang, J. Xu, L. Zong, Y. Kang and A. Wang, Powder Technol., 2021, 390, 303–314 CrossRef CAS.
- N. I. Gumerova and A. Rompel, Nat. Rev. Chem., 2018, 2, 1–20 CrossRef.
- O. R. Tambunan and R. Mohadi, J. Sci. Technol., 2017, 2, 1–8 Search PubMed.
- N. A. Ramsahye, T. K. Trung, L. Scott, F. Nouar, T. Devic, P. Horcajada, E. Magnier, O. David, C. Serre and P. Trens, Chem. Mater., 2013, 25, 479–488 CrossRef CAS.
- S. M. Wang, J. Hwang and E. Kim, J. Mater. Chem., 2019, 7, 7828–7850 CAS.
- C. T. Buru, P. Li, B. L. Mehdi, A. Dohnalkova, A. E. Platero-Prats, N. D. Browning, K. W. Chapman, J. T. Hupp and O. K. Farha, Chem. Mater., 2017, 29, 5174–5181 CrossRef CAS.
- A. M. Andani, T. Tabatabaie, S. Farhadi and B. Ramavandi, RSC Adv., 2020, 10, 32845–32855 RSC.
- F. Mahmoudi and M. M. Amini, J. Water Proc. Eng., 2020, 35, 101227 CrossRef.
- R. Narayan, U. Y. Nayak, A. M. Raichur and S. Garg, Pharmaceutics, 2018, 10(1–49), 118 CrossRef CAS.
- A. Benítez, J. Amaro-Gahete, D. Esquivel, F. J. Romero-Salguero, J. Morales and Á. Caballero, Nanomaterials, 2020, 10, 424 CrossRef.
- V. P. Viswanathan, K. Divya, D. P. Dubal, N. N. Adarsh and S. Mathew, Dalton Trans., 2021, 50, 2891–2902 RSC.
- A. Jarrah and S. Farhadi, RSC Adv., 2018, 8, 37976–37992 RSC.
- T. Wang, P. Zhao, N. Lu, H. Chen, C. Zhang and X. Hou, Chem. Eng. J., 2016, 295, 403–413 CrossRef CAS.
- J. Wu, S. Jin, X. Wei, F. Gu, Q. Han, Y. Lan, C. Qian, J. Li, X. Wang and R. Zhang, Chem. Eng., 2021, 412, 128712 CrossRef CAS.
- A. Benítez de la Torre, J. Amaro-Gahete, D. Esquivel, F. J. Romero-Salguero, J. Morales and Á. Caballero, Nanomaterials, 2020, 10, 424 CrossRef.
- Y. Yue, Z. Peng, W. Wang, Y. Cai, F. Tan, X. Wang and X. Qiao, Powder Technol., 2019, 347, 1–9 CrossRef CAS.
- Y. X. Song, S. Chen, N. You, H. T. Fan and L. N. Sun, Chemosphere, 2020, 255, 126917 CrossRef CAS.
- Y. K. Manea, A. M. Khan, A. A. Wani, M. T. Qashqoosh, M. Shahadat and M. A. Salem, J. Mol. Liq., 2021, 335, 116144 CrossRef CAS.
- Z. Li, M. Ma, S. Zhang, Z. Zhang, L. Zhou, J. Yun and R. Liu, J. Porous Mater., 2020, 27, 189–204 CrossRef CAS.
- A. Jain, A. Sharma, A. Kapur, S. Wadhawan, M. Garg, S. K. Pandey, S. Singh and S. K. Mehta, J. Nanostruct. Chem., 2021, 11, 437–453 CrossRef CAS.
- Y. Zhou, S. Cao, C. Xi, X. Li, L. Zhang, G. Wang and Z. Chen, Bioresour. Technol., 2019, 292, 121951 CrossRef CAS.
- G. Sharma, B. Thakur, A. Kumar, S. Sharma, M. Naushad and F. J. Stadler, Macromol. Mater. Eng., 2020, 305, 2000274 CrossRef CAS.
- Y. Ma, M. Li, P. Li, L. Yang, L. Wu, F. Gao, X. Qi and Z. Zhang, Bioresour. Technol., 2021, 319, 124199 CrossRef CAS.
- G. Zhang, R. Wo, Z. Sun, L. Xiao, G. Liu, G. Hao, H. Guo and W. Jiang, Front. Chem., 2021, 9, 707559 CrossRef CAS.
- Z. Song, Y. L. Ma and C. E. Li, Sci. Total Environ., 2019, 651, 580–590 CrossRef CAS.
- D. Qiao, Z. Li, J. Duan and X. He, Chem. Eng. J., 2020, 400, 125952 CrossRef CAS.
- H. Molavi, H. Moghimi and R. A. Taheri, Appl. Organomet. Chem., 2020, 34, e5549 CrossRef CAS.
- J. Bao, Y. Zhu, S. Yuan, F. Wang, H. Tang, Z. Bao, H. Zhou and Y. Chen, Nanoscale Res. Lett., 2018, 13, 396 CrossRef.
- D. Naghipour, K. Taghavi, J. Jaafari, I. Kabdaşlı, M. Makkiabadi, M. Javan Mahjoub Doust and F. Javan Mahjoub Doust, Environ. Technol., 2023, 44, 150–160 CrossRef CAS.
- T. Ahamad, M. Naushad, T. Al-Shahrani, N. Al-Hokbany and S. M. Alshehri, Int. J. Biol. Macromol., 2020, 147, 258–267 CrossRef CAS.
- T. Ahamad, A. A. Chaudhary, M. Naushad and S. M. Alshehri, Int. J. Biol. Macromol., 2019, 134, 180–188 CrossRef CAS.
- W. Xiong, G. Zeng, Z. Yang, Y. Zhou, C. Zhang, M. Cheng, Y. Liu, L. Hu, J. Wan, C. Zhou, R. Xu and X. Li, Sci. Total Environ., 2018, 627, 235–244 CrossRef CAS.
- R. Foroutan, S. J. Peighambardoust, P. Latifi, A. Ahmadi, M. Alizadeh and B. Ramavandi, J. Environ. Chem., 2021, 9, 106344 CrossRef CAS.
- L. P. Hoang, T. M. P. Nguyen, H. T. Van, M. Yılmaz, T. K. Hoang, Q. T. Nguyen, T. M. H. Vi and L. T. Q. Nga, Environ. Technol. Innovation, 2022, 28, 102914 CrossRef CAS.
- Y. Sun, M. Chen, H. Liu, Y. Zhu, D. Wang and M. Yan, Appl. Surf. Sci., 2020, 525, 146614 CrossRef CAS.
- J. Yu, W. Xiong, X. Li, Z. Yang, J. Cao, M. Jia, R. Xu and Y. Zhang, Microporous Mesoporous Mater., 2019, 290, 109642 CrossRef CAS.
- X. Zhang, X. Lin, Y. He and X. Luo, Int. J. Biol. Macromol., 2019, 136, 445–459 CrossRef CAS.
- N. Tian, Q. Jia, H. Su, Y. Zhi, A. Ma, J. Wu and S. Shan, J. Porous Mater., 2016, 23, 1269–1278 CrossRef CAS.
|
This journal is © The Royal Society of Chemistry 2023 |
Click here to see how this site uses Cookies. View our privacy policy here.